DOI:
10.1039/C7RA03579A
(Paper)
RSC Adv., 2017,
7, 29540-29549
Dual temperature- and pH-responsive polymeric micelle for selective and efficient two-step doxorubicin delivery†
Received
28th March 2017
, Accepted 31st May 2017
First published on 6th June 2017
Abstract
We report a polymeric micelle drug delivery system, which enables selective intracellular uptake with external thermal stimulation, and effective release of a drug at internal acidic endosomal pH. We developed a dual temperature- and pH-responsive polymeric micelle composed of a temperature-responsive corona segment with poly(N-isopropylacrylamide-co-dimethylacrylamide) [P(NIPAAm-co-DMAAm)] and a pH-responsive core segment with poly[2-(diisopropylamino)ethyl methacrylate] (PDPA). A dual temperature- and pH-responsive amphiphilic diblock copolymer was synthesized by reversible addition–fragmentation chain transfer (RAFT) polymerization. The dithiobenzoate end-group was removed with radical-induced ester exchange. This copolymer formed nano-sized micelles in aqueous solution, and encapsulated anti-cancer agent, doxorubicin (DOX). The resultant micelles exhibited a temperature-dependent phase transition at a temperature slightly higher than body temperature, and intracellular uptake of encapsulated DOX was accelerated above the phase transition temperature. A transition from neutral to positive charge, leading to micelle disassembly, accelerated the release of Nile red as a model drug. The cytotoxicity of doxorubicin (DOX)-loaded dual temperature- and pH-responsive micelles against human cervical cancer HeLa cells was significantly greater at 42 °C than at 37 °C, while no significant temperature-dependent cytotoxicity was observed with DOX-loaded micelles that were only temperature-responsive. This proof-of-concept synergistic two-step delivery system with enhanced intracellular uptake upon external thermal stimulation and rapid release of DOX at internal acidic endosomal pH was effective against tumor cells in vitro.
Introduction
Nanomedicines such as micelles, liposomes, and particles with an enhanced permeation and retention (EPR) effect are effective for tumor chemotherapy.1,2 Polyethylene glycol (PEG) contributed to the development of nanomedicines,3,4 as its incorporation onto their surface prolongs their circulation in the bloodstream after intravenous administration. However, PEG prevents intracellular uptake and endosomal escape, which results in the loss of drug activity. These issues regarding the use of PEG for tumor drug delivery are referred to as the “PEG dilemma”.5,6 A variety of strategies were studied to overcome these drawbacks. One of these strategies is the modification of the end-group of the PEG segment with ligands such as folate,7 cyclic Arg–Gly–Asp (cRGD) peptide,8 and antibody fragments9 that recognize cell-specific surface receptors, leading to the improvement of cellular selectivity and increased intracellular delivery of nanomedicines. Stimuli-responsive polymers or “smart materials”,10 whose characteristics undergo appropriate changes in response to surrounding environmental conditions,11–14 were also studied for tumor targeting and effective release of anti-tumor agents.15
Hyperthermia, a procedure during which localized tumor tissue is heated to 40–43 °C, can synergistically enhance tumor cytotoxicity when combined with chemotherapy.16 Besides the therapeutic effect of hyperthermia, temperature-responsive polymers, which exhibited reversible temperature-dependent hydrophilic/hydrophobic phase transition, were applied to a drug carrier for a stimuli-responsive targeting.17,18 When this system was applied to in vivo systems, temperature-responsive polymer accumulated to local heated tissue as aggregation and precipitation. In some cases, the polymer aggregate were sheared from local heated tissue, and carried away by the blood flow. Some polymeric micelles washed from the local heated tissue would be expected to rapidly disaggregate, and recovery its hydrophilic corona due to reversible temperature-responsive characteristic.19 The tumor extracellular microenvironment exhibits acidic pH values (pHe: pH ∼6.8) due to the high rate of glycolysis in tumor cells resulted in high lactic acid levels of extracellular microenvironment, both in aerobic and anaerobic conditions.20 Intracellular endo/lysosome are an acidic organelle (pHi: pH 5.0–6.0).21 These different pH values are focus of attention for efficient anti-tumor drug delivery with pH responsive materials. Various pH-responsive nanomedicines have been developed for efficient drug delivery.22 Furthermore, dual stimuli responsive nanomedicines in response to an internal or external stimulus, such as dual pH,23,24 reduction/pH,25,26 enzyme/temperature,27 light/pH,28,29 were developed.30
Stimuli-responsive polymers are categorized into two types. The first type is characterized by a change in the equilibrium of the stimulus-responsive moiety.31 The second type is characterized by the cleavage of covalent chemical bonds upon stimuli. The former has the advantage to be reversible, and to respond more rapidly than the latter. The dual stimuli-responsive nanomedicines that have been developed to date undergo cleavage of covalent chemical bonds as a response to at least one of both stimuli and to the best of our knowledge, double equilibrium changing stimuli-responsive micelles have never been reported. In the present study, we developed dual temperature- and pH-responsive polymeric micelles based on reversible- and rapid-phase transition polymer blocks. These polymeric micelles were applied for a selective intracellular uptake in solid tumor cells upon external local heating, and an effective intracellular release of the anti-tumor agent at internal endosomal pH (Scheme 1).
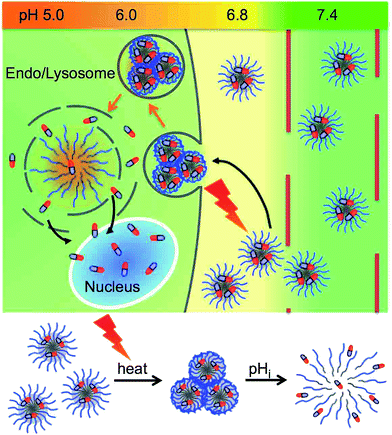 |
| Scheme 1 Schematic illustration of temperature-responsive intracellular uptake, and pH-responsive drug release mechanism of drug loaded dual temperature- and pH-responsive polymeric micelle. | |
The amphiphilic diblock copolymer comprises temperature-responsive corona segments of poly(N-isopropylacrylamide-co-N,N-dimethylacrylamide) P(NIPAAm-co-DMAAm), and a pH-responsive core segment of poly[2-(diisopropylamino)ethyl methacrylate] (PDPA). This diblock copolymer forms a core–shell type polymeric micelle in water, which can encapsulate the hydrophobic anti-tumor agent doxorubicin (DOX). This polymeric micelle was characterized for their temperature-, and pH-responsive, hydrodynamic diameters, and critical micelle concentrations. The efficacy of polymeric micelles in anti-tumor agent delivery is evaluated in human cervical cancer HeLa cells.
Experimental section
Materials
N-Isopropylacrylamide (NIPAAm) provided by KJ Chemicals Co. (Tokyo, Japan) was purified by recrystallization from n-hexane and dried at 25 °C in vacuo. Butyl methacrylate (BMA), N,N-dimethylacrylamide (DMAAm), 2,2′-azobisisobutyronitrile (AIBN), 2-aminoethanol, 1,4-dioxane, and dimethylformamide (DMF) were purchased from Wako Pure Chemical Industries Ltd (Osaka, Japan). BMA and DMAAm were distilled under reduced pressure before use. AIBN was recrystallized from methanol. 2-Cyano-2-propyl benzodithioate (CPBD), 2-(diisopropylamino)ethyl methacrylate (DPA) were purchased from Sigma-Aldrich (St. Louis, MO, USA). DPA passed through a neutral alumina column before use. Doxorubicin hydrochloride (DOX·HCl), and 5-dimethylthiazol-2-yl)-2,5-diphenyl tetrazolium bromide (MTT) were purchased from Tokyo Kasei Industry (Tokyo, Japan). Dialysis membranes [Spectra/Por 2 or 3, molecular weight cut-off (MWCO) 12–14 kDa and 3500 Da] were purchased from Spectrum Laboratories (Rancho dominguez, CA, USA). Fetal bovine serum (Central America production, USDA approved) was purchased from Biosera (Kansas City, MO, USA).
Synthesis of poly[2-(diisopropylamino)ethyl methacrylate] (PDPA)
DPA (2.00 g, 9.41 mmol), and CPBD (86 mg, 0.39 mmol) as the RAFT chain transfer agent (CTA), AIBN (14 mg, 0.085 mmol) as a radical initiator, and the internal standard of 1,3,5-trioxane (one thirty weight of monomers) were dissolved in 3 mL 1,4-dioxane. The reaction mixture was degassed by a bubbling with nitrogen for 30 min, and after heating it to 70 °C for 20 h. The reaction solution was poured into methanol to precipitate the polymer, followed by drying to afford a pink solid. DPA conversion rate was 99%.
Synthesis of poly(butyl methacrylate) (PBMA)
BMA (2.00 g, 14.1 mmol), and CPBD (84 mg, 0.38 mmol) as the RAFT-CTA, AIBN (14 mg, 0.085 mmol) as a radical initiator, and the internal standard of 1,3,5-trioxane (one thirty weight of monomers) were dissolved in 4 mL 1,4-dioxane. The reaction mixture was degassed by a bubbling with nitrogen for 30 min, and after heating it to 70 °C for 20 h. The reaction solution was poured into methanol to precipitate the polymer, followed by drying to afford a pink solid. BMA conversion rate was 98%.
Diblock copolymerization of poly(NIPAAm-co-DMAAm) with PDPA (PDP-b-ND)
NIPAAm (1.21 g, 10.7 mmol), and DMAAm (0.458 g, 4.62 mmol) were dissolved in 4 mL 1,4-dioxane. Then, AIBN (3.1 mg, 0.019 mmol) as a radical initiator, PDPA (0.500 g, 0.0939 mmol) as MacroCTA, and the internal standard of 1,3,5-trioxane (one thirty weight of monomers) were added to the solution. The reaction mixture was degassed by a bubbling with nitrogen for 30 min, and after heating it to 70 °C. After reacting for 20 h, the reaction solution was poured into hexane
:
diethylether = 4
:
1 to precipitate the polymer. The crude was further purified by repeated precipitation from an acetone solution into hexane
:
diethylether = 4
:
1, followed by drying to afford a pale pink solid. NIPAAm, and DMAAm conversion rate were 57%, and 67%, respectively.
This solid (800 mg) was dissolved in 50 mL ethanol. Then, AIBN (149 mg, 0.907 mmol) was added to the solution. The reaction mixture was degassed by a bubbling with nitrogen for 30 min, and after heating to 80 °C. After reacting for 15 h, the solvent was evaporated and the resulting residue was dialyzed against methanol, and finally deionized water using dialysis membranes (MWCO 3500 Da) at room temperature. The obtained micelles solution was freeze-dried.
Diblock copolymerization of poly(NIPAAm-co-DMAAm) with PBMA (PBM-b-ND)
NIPAAm (1.10 g, 9.72 mmol), DMAAm (0.441 g, 4.45 mmol) were dissolved in 5 mL 1,4-dioxane. Then, AIBN (3.1 mg, 0.019 mmol) as a radical initiator, PBMA (0.502 g, 0.0930 mmol) as MacroCTA, and the internal standard of 1,3,5-trioxane (one thirty weight of monomers) were added to the solution. The reaction mixture was degassed by a bubbling with nitrogen for 30 min, and after heating it to 70 °C. After reacting for 20 h, the reaction solution was poured into hexane
:
diethylether = 4
:
1 to precipitate the polymer. The crude was further purified by repeated precipitation from an acetone solution into hexane
:
diethylether = 4
:
1, followed by drying to afford a pale pink solid. NIPAAm, and DMAAm conversion rate were 55%, and 65%.
This solid (800 mg) was dissolved in 50 mL ethanol. Then, AIBN (149 mg, 0.907 mmol) was added to the solution. The reaction mixture was degassed by a bubbling with nitrogen for 30 min, and after heating to 80 °C. After reacting for 15 h, the solvent was evaporated and the resulting residue was dialyzed against methanol, and finally deionized water using dialysis membranes (MWCO 3500 Da) at room temperature. The obtained micelles solutions were freeze-dried.
Characterization of polymers
1H NMR spectra were recorded at 400 MHz on an Agilent 400-MR spectrometer. The monomer conversions of each reaction were determined by 1H NMR analysis. For the conversion rate calculation, 1,3,5-trioxane peak was compared to the unreacted monomer peaks. The molecular weight of the polymer was determined by Gel Permeation Chromatography (GPC) analysis (GPC-8020 system; column, one TSKgel guardcolumn α and two TSKgel α-M connecting in a series; mobile phase, DMF containing 10 mM LiCl; TOSOH, Tokyo, Japan), calibrated with polyethylene oxide standards.
Characterization of polymeric micelles
The obtained micelles powders were dissolved in aqueous buffer solutions. The LCST of polymeric micelles as a function of pH were determined by measuring the optical transmittance of its aqueous buffer solution (0.1 w/v%). The optical transmittance of the polymer solution was measured at 500 nm at various temperatures using a UV-Vis spectrophotometer (V-630, JASCO, Tokyo, Japan) and a PT-31 Peltier system (KRÜSS, Hamburg, Germany); the heating rate was 0.1 °C min−1. The LCST was determined as the temperature at 50% of the optical transmittance of the polymer solution. In the case of reversible temperature-responsiveness evaluation, optical transmittances were measured after 30 seconds temperature was changed. The hydrodynamic diameters of polymeric micelles were determined using dynamic light scattering (Zetasizer Nano Series, Malvern Instrument Ltd., Malvern, UK). Zeta potential was measured using a zeta potential analyzer (ELSZ-2KOP, Otsuka Electronics Co., Ltd., Osaka, Japan). Fluorescence spectra of pyrene were recorded by a FP-6300 spectrofluorometer (JASCO) with the excitation wavelength 339 nm at 37 °C for the determining critical micelle concentration (CMC) of polymeric micelles. Pyrene was dissolved in acetone (100 μM), and 5 μL pyrene solution was added into 2 mL aqueous micelle solution with various concentrations. The fluorescence emission intensities at 373 and 383 nm were monitored.
Preparation of DOX loading polymeric micelles
DOX was loaded into polymeric micelles by the dialysis method. 40 mg diblock copolymer, 4 mg DOX·HCl, 10 μL triethyl amine were dissolved in 2 mL DMF, and then the solutions were stored for 3 h at room temperature. DOX was neutralized with triethylamine. After 2 mL deionized water was then slowly added into the DMF solution under vigorous stirring, and then the solution was dialyzed against deionized water using dialysis membranes (MWCO 12–14 kDa), and finally dialysis was continued until no DOX leakage. DOX concentration was measured based on the absorbance intensity of DOX at 480 nm using a UV-Vis spectrophotometer. The drug loading content (DLC) and drug loading efficiency (DLE) were calculated using the following equations:
DLC (%) = (weight of loaded DOX/weight of DOX loaded NPs) × 100% |
DLE (%) = (weight of loaded DOX/weight of DOX in feed) × 100% |
Nile red assay
The pH-responsive drug release behavior of PDP-b-ND, and PBM-b-ND micelles was proved by Nile red assay. To examine the pH-dependent dye release, 100 μL of Nile red loaded micelle solutions (4 mg mL−1) were incubated in 1.9 mL of 5 mmol L−1 Na2HPO4/NaH2PO4 containing 150 mmol L−1 NaCl buffer solutions as a function of pH for 1 min, then, the fluorescence spectra (from 550 nm to 700 nm) were measured by a FP-6300 spectrofluorometer (JASCO) with the excitation wavelength 540 nm at 37 °C.
Cell culture
Human cervical cancer HeLa cells (RIKEN BRC CELL BANK) were cultured as subconfluent monolayers in a 100 mm culture dish in minimum essential medium Eagle (MEM) with non-essential amino acids (NEAA), supplemented with 10% fetal bovine serum (FBS), 50 units mL−1 of penicillin, and 50 μg mL−1 of streptomycin, in a humidified incubator at 37 °C with 5% CO2.
Temperature-dependent intracellular uptake of DOX loaded polymeric micelles
HeLa cells were seeded into 35 mm glass bottom dishes (5 × 104 cells, 2 mL per dish) and cultured for 1 day at 37 °C in MEM with NEAA supplemented with 10% FBS in a humidified atmosphere containing 5% CO2. Cultured cells were exposed to DOX loaded PDP-b-ND, or PBM-b-ND (100 μg mL−1) in MEM with 10% FBS at 37 °C or at 42 °C for 2 h with 5% CO2. After incubation, HeLa cells were rinsed once with a DPBS (−) buffer. For observing subcellular distribution, HeLa cells were seeded according to the description mentioned above. After incubation with PDP-b-ND, or PBM-b-ND (10 μg mL−1) for 2 h at 42 °C, Hela cells were rinsed once with a DPBS (−) buffer and incubated for another 15 min with LysoTracker® Green (Invitrogen, 1.5 μM), and cell nuclei staining Hoechst 33342 (Invitrogen, 1 mg mL−1) in MEM followed by rinsing once with DPBS (−). Samples were visualized with glass bottom dishes using a BZ-9000 microscope (Keyence, Osaka, Japan).
Cytotoxicity assay
The cytotoxicity to HeLa cells was evaluated by MTT assay. HeLa cells were seeded in a 24-well plates (1 × 104 cells, 500 μL per well) and cultured for 1 day at 37 °C in MEM with NEAA supplemented with 10% FBS in a humidified atmosphere containing 5% CO2. Cultured cells were exposed to DOX loaded PDP-b-ND, and PBM-b-ND in MEM with 10% FBS at 37 °C or at 42 °C for 2 h with 5% CO2. After incubation, HeLa cells were rinsed twice with a DPBS (−) buffer. The medium replaced with 500 μL of fresh MEM with 10% FBS. After incubation for another 46 h, medium was replaced with 500 μL of fresh MEM with 5 mg mL−1 MTT in PBS (finally diluted to 500 μg mL−1). After incubation for an additional 2 h, 500 μL of the extraction buffer (acidified isopropanol) was added to the wells and the plate was shaken carefully. The absorbance of the solution was measured at 570 nm, and 630 nm (reference) by TECAN Infinite M1000 plate reader. The relative percentage of cell survival was calculated based on the 100% arbitrary absorbance obtained for the blank control. Reported values represent the mean standard deviation of 3 replicates on the same plate.
When evaluating the cytotoxicity of blank polymeric micelles, cultured cells were exposed to DOX free PDP-b-ND, and PBM-b-ND for 4 h at 42 °C followed for 44 h at 37 °C without medium change, then, MTT assay was performed in the same procedure above.
Results and discussion
Design and synthesis of dual temperature- and pH-responsive polymeric micelle
A pH-responsive core block PDPA was synthesized by RAFT polymerization using CPBD as a chain transfer agent (CTA). Amino groups have been incorporated into the polymers and were imparted a positive charge with decreasing pH, which induced the polymer chain to change from hydrophobic to hydrophilic. When a pH-responsive polymer with amino groups was applied to the core segment, the neutral amphiphilic diblock copolymer self-assembled with hydrophobic core at higher pH, whereas decreasing the pH led to the protonation of the core segment (hydrophilic) and induced fast disassembly of the micelle.32 The pH range, in which PDPA is responsive, is suitable for the disruption of micelles in acidic endo-/lysosomes and promotes drug release from the endocytosed drug carriers. A neutral hydrophobic core block PBMA was also synthesized with the same procedure as was used for the synthesis of PDPA. Next, the temperature-responsive corona segment P(NIPAAm-co-DMAAm) was synthesized by RAFT polymerization using PDPA or PBMA as macro-CTA. The dithiobenzoate end-group derived from the RAFT reagents was removed via radical-induced ester exchange.33 PNIPAAm exhibits a temperature-dependent phase transition at its lower critical solution temperature (LCST),34 which can be accurately raised to a slightly higher temperature than body temperature with hydrophilic co-monomers,35 and intracellular uptake of PNIPAAm-based polymers, and nanomaterials can be controlled with temperature-dependent hydrophilic/hydrophobic phase transition.17,36,37 The two diblock copolymers, obtained using PDPA and PBMA as CTA, were referred to as PDP-b-ND and PBM-b-ND, respectively (Scheme 2). The number- and weight-averaged molecular weight (Mn, and Mw) and ratio of the two synthesized polymers were analyzed by 1H NMR and gel-permeation chromatography (GPC), as shown in Table 1. In the case of 1H NMR analysis, the molecular weights, and copolymer compositions were calculated with monomer conversions. 1H NMR spectra of PDPA, PBMA, PDP-b-ND, and PBM-b-ND were shown in the ESI.† The copolymerization of two diblock polymers was confirmed by significant increase in molecular weight with GPC analysis.
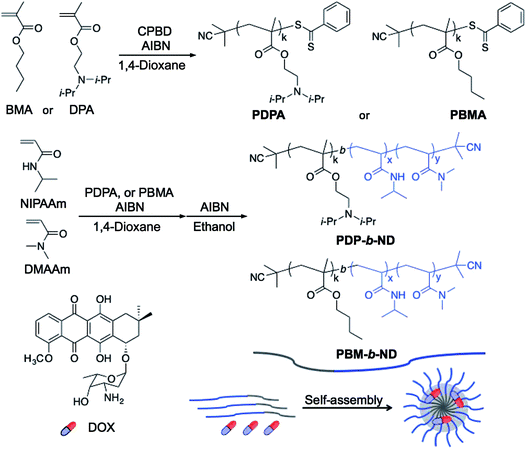 |
| Scheme 2 Synthesis of PDPA, PBMA, PDP-b-ND and PBM-b-ND. Amphiphilic diblock copolymers formed core–shell type polymeric micelles with self-assembly, and encapsulated DOX in hydrophobic core. | |
Table 1 Characterization of the synthesized polymers
|
Monomer unitsa |
Mn,NMRb |
Mn,GPCc |
Mw/Mnd |
DPA |
BMA |
NIPAAm |
DMAAm |
Determined by 1H NMR. Mn determined by 1H NMR. Mn determined by GPC. Estimated by GPC. |
PDPA |
24 |
— |
— |
— |
5300 |
3700 |
1.11 |
PDP-b-ND |
24 |
— |
72 |
36 |
17 000 |
19 000 |
1.38 |
PBMA |
— |
36 |
— |
— |
5400 |
4000 |
1.10 |
PBM-b-ND |
— |
36 |
70 |
37 |
17 000 |
16 800 |
1.35 |
These amphiphilic diblock copolymers formed core–shell type polymeric micelles in aqueous solution via the self-assembly of core segments, which can encapsulate hydrophobic DOX in their hydrophobic core. PDP-b-ND was designed to achieve a two-step DOX delivery with selective intracellular uptake via local tumor heating, and an effective release at acidic endo-/lysosomal pH with each of the segments responding to the stimuli independently. This system enables drug delivery specifically to the solid tumor with minimum drug leakage. DOX contents of PDP-b-ND, and PBM-b-ND micelles were 3.8% and 4.3% with a drug-loading efficiency of 43.4% and 48.6%, respectively.
Characterization of dual temperature- and pH-responsive polymeric micelle
The temperature-dependent optical transmittances of the PDP-b-ND and PBM-b-ND solutions as a function of pH are shown in Fig. 1a and b. Sharp transmittance curves were observed for PDP-b-ND at pH 7.4 and 6.4, and for PBM-b-ND at all pH. Their LCSTs were between 38.5 °C and 39.0 and controlled to a temperature slightly higher than body temperature, adjusting the composition ratio of NIPAAm and DMAAm. In addition, their optical transmittances at 36–37 °C (below LCST) was 90–93%, which were relatively lower than optical transmittance at 36–37 °C of PDP-b-ND at pH 5.4, indicating light scattering with the polymeric micelle (the Tyndall effect). Indeed, intense light scattering of the incident laser could be clearly observed (Fig. 1c). These results indicated that the corona of polymeric micelles was hydrophilic at body temperature, whereas it was hydrophobic at a temperature higher than 39 °C. At 36–40 °C and at pH 5.4, the optical transmittance of PDP-b-ND was above 99% and a lower light scattering of the incident laser was observed (Fig. 1c). This means that the Tyndall effect of the PDP-b-ND micelles was weakened due to a transition from micelle to unimer state, which implies that the PDPA core moiety transitioned from a hydrophobic to a positively charged hydrophilic state with decreasing pH. This transition is probably at the origin of the broadened transmittance curve and elevated LCST (above 42 °C) of PDP-b-ND at pH 5.4. Since the PDPA moiety of PDP-b-ND is a macro terminal group of P(NIPAAm-co-DMAAm), a large LCST shift was observed with lowering pH, which is not surprising as the LCST can even be affected by small molecules as terminal group.38
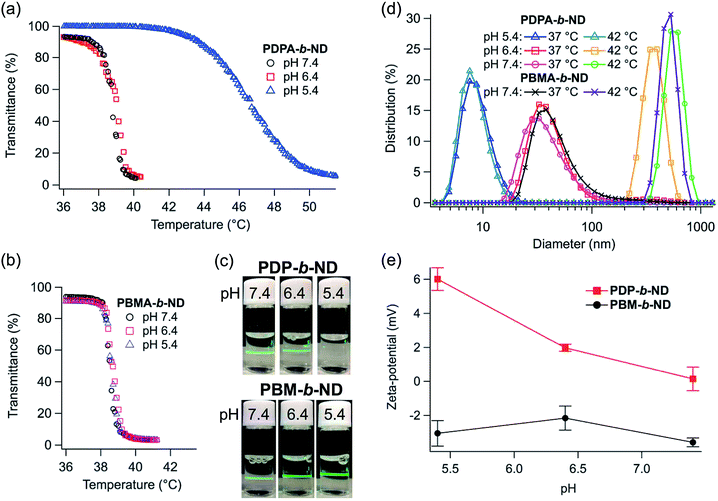 |
| Fig. 1 Temperature-dependent optical transmittances of solutions at 500 nm at a heating rate of 0.1 °C min−1. (a) PDP-b-ND, and (b) PBM-b-ND. (c) Photographs of PDP-b-ND, and PBM-b-ND with a green laser from the right hand side; 0.1 w/v% solutions in 5 mmol L−1 Na2HPO4/NaH2PO4 containing 150 mmol L−1 NaCl at pH 7.4, 6.4, and 5.4. (d) Hydrodynamic diameters of PDP-b-ND, and PBM-b-ND (200 μg mL−1) at pH 7.4, 6.4, and 5.4 in 5 mmol L−1 Na2HPO4/NaH2PO4 containing 150 mmol L−1 NaCl. (e) The zeta-potential of PDP-b-ND, and PBM-b-ND (200 μg mL−1) in 5 mmol L−1 Na2HPO4/NaH2PO4. Error bars represent mean values ±1σ (n = 3). | |
The temperature- and pH-dependent hydrodynamic diameter of PDP-b-ND, and PBM-b-ND were measured, and Fig. 1d shows the size distributions as a function of pH at 37 and 42 °C. In the case of pH 7.4, and 6.4, the hydrodynamic diameters of the PDP-b-ND were around 50 nm, indicating that monodispersed micelles are formed at 37 °C, attributable to the hydrophilic corona. The hydrodynamic diameters increased to several hundred nm sizes at 42 °C, which is caused by the temperature-responsive corona of micelles changing from hydrophilic to hydrophobic characteristic, and subsequent aggregation by hydrophobic interactions between micelles. The temperature-responsive hydrodynamic size behaviors of PBM-b-ND were independent of the pH, and comparable to the behavior of PDP-b-ND at pH 7.4, and 6.4. At pH 5.4, the hydrodynamic diameters were ca. 9 nm regardless of temperature, which supported that micelles of PDP-b-ND were broken down into individual polymers with lowering pH, as mentioned above. Moreover, the zeta-potential of PDP-b-ND increased from around 0 mV to positive (∼+6.0 mV) with lowering pH. PDPA core was positively charged by protonation of (diisopropylamino)ethyl groups. In contrast, the zeta-potential of PBM-b-ND was slightly negative (around −3 mV), and did not appreciably change with pH values (Fig. 1e). Reversible temperature-responsiveness of PDP-b-ND, and PBM-b-ND at pH 7.4 were evaluated for three cycles between 37 °C and 42 °C as shown in Fig. 2. Reversible and fast phase transitions were clearly observed with temperature switching.
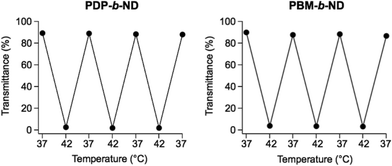 |
| Fig. 2 Reversibility of temperature-dependent optical transmittances at 500 nm of PDP-b-ND, and PBM-b-ND solutions at pH 7.4. The temperatures were cyclically changed between 37 °C and 42 °C. | |
In order to investigate the disruption of PDP-b-ND micelles with lowering pH, critical micelle concentration (CMC) were evaluated from the fluorescence spectra of pyrene, used as hydrophobic fluorescence probe.39 Once the polymeric micelle formed, pyrene molecules were preferentially incorporated into the hydrophobic core of the polymeric micelles and its fluorescence characteristic was changed drastically. Fig. 3a shows polymer concentration-dependent fluorescence intensity ratio (I373/I383) of pyrene. The CMC was estimated as the cross-point of two straight lines at low and high concentration regions. The CMC of PDP-b-ND at pH 7.4 was 7 μg mL−1, which increased to 159 μg mL−1 at pH 6.4, and was not observed (concentration < 600 μg mL−1) at pH 5.4. These results indicated that PDP-b-ND micelle at physiological pH is transformed to unimer state with lowering pH. The CMC of PBM-b-ND micelle was 1 μg mL−1, regardless of the pH, and was slightly lower than the CMC value of PDP-b-ND. The PBMA core moiety of PBM-b-ND, which was more hydrophobic than the PDPA core moiety of PDP-b-ND, formed stable micelles. The state of PDP-b-ND depending on temperature and pH is summarized in Fig. 3b.
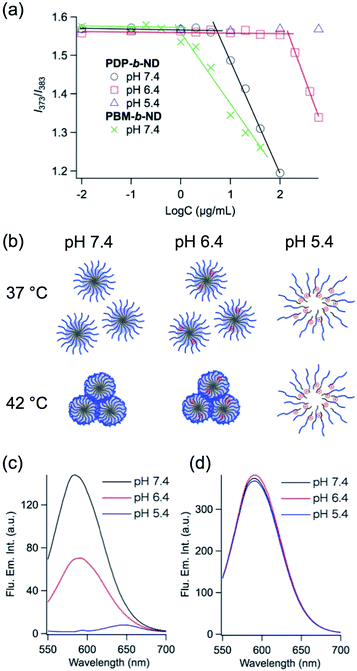 |
| Fig. 3 (a) Block polymer concentration-dependent pyrene fluorescence emission ratios (I373/I383) in 5 mmol L−1 Na2HPO4/NaH2PO4 containing 150 mmol L−1 NaCl with PDP-b-ND at pH 7.4 (open circle), pH 6.4 (open square), pH 5.4 (open triangle), and PBM-b-ND at pH 7.4 (cross mark) at 37 °C. The concentration of pyrene is 0.25 μM; the samples were excited at 339 nm. (b) Schematic illustration of PDP-b-ND micelles as a function of temperature and pH. Fluorescence emission spectra in function of the pH of Nile red (excited at 540 nm) encapsulated in (c) PDP-b-ND and (d) PBM-b-ND in 5 mmol L−1 Na2HPO4/NaH2PO4 solution containing 150 mmol L−1 NaCl buffer after incubation for 1 min. | |
Nile red is a fluorescent probe whose fluorescence is strongly influenced by the polarity of its environment, and inherently insoluble in water. Once Nile red as a hydrophobic model drug was embedded in hydrophobic core of micelles, it had strong fluorescence, whereas fluorescence was very weak after the release to aqueous medium from micelle. Therefore, the Nile red assay is a sensitive and convenient method to test the release behavior of nanomaterials.40,41 Fig. 3c and d show fluorescence emission spectra of Nile red encapsulated in PDP-b-ND, and PBM-b-ND micelles (200 μg mL−1) as a function of pH after incubation for 1 min. In the case of PBM-b-ND solutions, fluorescence spectra with maximum emission at 590 nm were observed, and fluorescence intensities were independent of pH. On the other hand, fluorescence intensities in PDP-b-ND micelles were decreasing and undergoing a small red shift (pH 7.4
:
584 nm, pH 6.4
:
591 nm, and pH 5.4
:
647 nm) from pH 7.4 to pH 5.4. Because Nile red is a solvatochromic fluorescent dye,42 which shows a red shift with increasing polarity, these results indicated that Nile red was quickly (within 1 min) released with the burst of PDP-b-ND with lowering pH. In the case of stimuli-sensitive cleavage of covalent bond types, drug release times were rather long (up to hours).23–29 This result of PDP-b-ND micelles is favorable to endosomal escape.
The temperature-dependent cellular uptake of dual temperature- and pH-responsive polymeric micelle
The temperature-dependent intracellular uptake of DOX-loaded PDP-b-ND and PBM-b-ND micelles was evaluated using human cervical cancer HeLa cells at 37 °C and at 42 °C, the temperature at which local heating cancer therapy is conducted. Red fluorescence derived from DOX was clearly observed upon incubation at 42 °C, compared with incubation at 37 °C with both micelles, as shown in the fluorescence microscopy images in Fig. 4. In our previous work,37 temperature-responsive polymeric nanoparticles with a P(NIPAAm-co-DMAAm) polymer brush attached on its surface showed a temperature-dependent cellular uptake, with its LCST as boundary. In accordance to our previous study, the temperature-responsive PDP-b-ND and PBM-b-ND micelles, which encapsulate DOX, were internalized into cells in a temperature-dependent manner, as they also have a P(NIPAAm-co-DMAAm) corona.
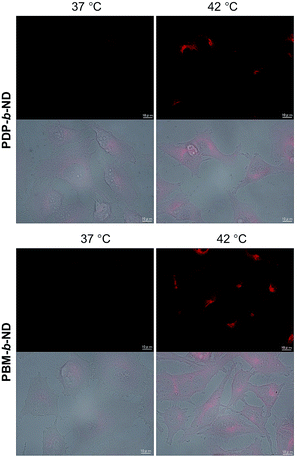 |
| Fig. 4 Fluorescence microscopy images (upper panels) and overlays of fluorescence and phase contrast images (lower panels) of HeLa cells incubated with DOX-loaded PDP-b-ND, and PBM-b-ND for 2 h at 37 °C and 42 °C. Scale bar: 10 μm. | |
The localization of DOX-loaded PDP-b-ND and PBM-b-ND was visualized by co-staining with lysosome-specific LysoTracker green, and cell nuclei staining Hoechst 33342 as shown in Fig. 5. In the case of DOX-loaded PBM-b-ND, the red fluorescence derived from DOX was predominantly merged with the green dot fluorescence corresponding to LysoTracker green. On the other hand, in the case of DOX-loaded PDP-b-ND, the red fluorescence was rarely merged with the green dot. These results indicated that the endosomal escape of DOX-loaded PDP-b-ND was accelerated with the disruption by endosomal acidic pH, which triggered rapid DOX release to cytoplasm.
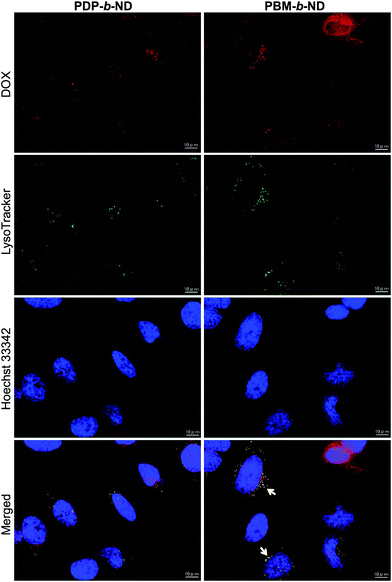 |
| Fig. 5 Fluorescence microscopy images of HeLa cells incubated with DOX-loaded PDP-b-ND, and PBM-b-ND (red) for 2 h at 42 °C, endosome-specific LysoTracker (green), nuclei staining Hoechst 33342, and merged images. Scale bar: 10 μm. | |
The temperature-dependent antitumor effect of DOX loaded dual temperature- and pH-responsive polymeric micelle
The temperature-dependent cytotoxicity of DOX-loaded and unloaded PDP-b-ND and PBM-b-ND micelles was evaluated using the MTT assay. As shown in Fig. 6a, significant greater cytotoxicity was observed with exposure to DOX-loaded PDP-b-ND at 42 °C compared to exposure at 37 °C. These results indicated that intracellular uptake of DOX encapsulated in PDP-b-ND was enhanced above LCST of PDP-b-ND, and correlated with the results of fluorescence microscopy imaging (Fig. 4). On the other hand, no significant temperature-dependent cytotoxicity was observed with exposure to DOX-loaded PBM-b-ND, regardless of temperature (Fig. 6b), although significant difference of intracellular uptake between exposure temperatures at 37 and 42 °C was observed with fluorescence microscopy imaging. The different cytotoxicity between PDP-b-ND, and PBM-b-ND micelles was due to the pH-responsive core moiety. The endocytosed PDP-b-ND micelles were disrupted with endosomal acidic pH, which triggered rapid DOX release, whereas the drug release from PBM-b-ND depended only on diffusion from the hydrophobic PBMA core. The blank polymeric micelles (∼400 μg mL−1) showed no significant cytotoxicity with a HeLa cell viability of >94% even when combined with temperature treatment, indicating the biocompatibility of micelles (Fig. S1 in the ESI†). It was inferred that DOX-loaded PDP-b-ND micelles with local heating treatment have the potential to achieve efficient anti-tumor effect with regression of side effect.
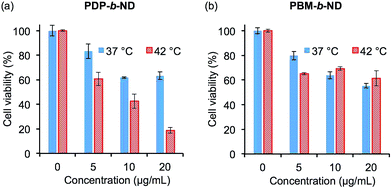 |
| Fig. 6 Concentration-dependent viability of HeLa cells after incubation DOX-loaded (a) PDP-b-ND, and (b) PBM-b-ND for 2 h at 37 °C, and 42 °C. Cell viabilities were measured after 46 h treatment. Error bars represent mean values ±1σ (n = 3). | |
Conclusions
In summary, we have developed dual temperature- and pH-responsive polymeric micelles, PDP-b-ND encapsulating DOX for selective and efficient cancer treatment. The hydrophilic/hydrophobic phase transition temperature of PDP-b-ND micelles with physiological pH was controlled to slightly higher than body temperature by the P(NIPAAm-co-DMAAm) copolymer composition ratio of the temperature-responsive segment in the amphiphilic diblock copolymer. This characteristic enabled the suppression of intracellular uptake with body temperature, and the enhancement of intracellular uptake with local heating. In addition, PDP-b-ND micelles, internalized into the cell via endocytosis, were disrupted with acidic endosomal pH, which led to an effective release of the encapsulated DOX into the cell. Such dual responsive polymeric micelles, based on an equilibrium-changing stimuli-responsive system have not been generated previously, and are believed to achieve simultaneously solid tumor selective uptake and rapid release of encapsulated drug. Significant enhanced intracellular uptake of DOX at 42 °C was observed, while limited uptake was observed at body temperature with fluorescence microscopy. In addition, significant cytotoxicity of DOX-loaded PDP-b-ND at 42 °C was observed, compared to 37 °C. On the other hand, the cytotoxicity of DOX-loaded PBM-b-ND was independent of temperature, and lower than the cytotoxicity of DOX-loaded PDP-b-ND. These results indicated that the synergistic two-step delivery system with the enhancement of intracellular uptake with external thermal stimulation, and rapid release of DOX with internal acidic endosomal pH was effective to tumor treatment. This two-step drug delivery system demonstrates potential for tumor-specific chemotherapy with the combination of local hyperthermia.
Acknowledgements
This study was supported by Grant-in-Aid for Young Scientists (B) (Grant No. 15K18846) to YH from Japan Society for the Promotion of Science (JSPS), and The Research Foundation for Pharmaceutical Sciences to YH.
Notes and references
- Y. Matsumura and H. Maeda, Cancer Res., 1986, 46, 6387 CAS.
- H. Maeda, J. Wu, T. Sawa, Y. Matsumura and K. Hori, J. Controlled Release, 2000, 65, 271–284 CrossRef CAS PubMed.
- A. Kolate, D. Baradia, S. Patil, I. Vhora, G. Kore and A. Misra, J. Controlled Release, 2014, 192, 67–81 CrossRef CAS PubMed.
- K. Kataoka, A. Harada and Y. Nagasaki, Adv. Drug Delivery Rev., 2001, 47, 113–131 CrossRef CAS PubMed.
- H. Hatakeyama, H. Akita and H. Harashima, Adv. Drug Delivery Rev., 2011, 63, 152–160 CrossRef CAS PubMed.
- M. Wang and M. Thanou, Pharmacol. Res., 2010, 62, 90–99 CrossRef CAS PubMed.
- Y. Bae, W. D. Jang, N. Nishiyama, S. Fukushima and K. Kataoka, Mol. BioSyst., 2005, 1, 242–250 RSC.
- Y. Miura, T. Takenaka, K. Toh, S. Wu, H. Nishihara, M. R. Kano, Y. Ino, T. Nomoto, Y. Matsumoto, H. Koyama, H. Cabral, N. Nishiyama and K. Kataoka, ACS Nano, 2013, 7, 8583–8592 CrossRef CAS PubMed.
- J. Ahn, Y. Miura, N. Yamada, T. Chida, X. Liu, A. Kim, R. Sato, R. Tsumura, Y. Koga, M. Yasunaga, N. Nishiyama, Y. Matsumura, H. Cabral and K. Kataoka, Biomaterials, 2015, 39, 23–30 CrossRef CAS PubMed.
- F. D. Jochum and P. Theato, Chem. Soc. Rev., 2013, 42, 7468–7483 RSC.
- Y. Hiruta, Y. Nagumo, Y. Suzuki, T. Funatsu, Y. Ishikawa and H. Kanazawa, Colloids Surf., B, 2015, 132, 299–304 CrossRef CAS PubMed.
- H. I. Lee, W. Wu, J. K. Oh, L. Mueller, G. Sherwood, L. Peteanu, T. Kowalewski and K. Matyjaszewski, Angew. Chem., Int. Ed., 2007, 46, 2453–2457 CrossRef CAS PubMed.
- Y. Hiruta, T. Funatsu, M. Matsuura, J. Wang, E. Ayano and H. Kanazawa, Sens. Actuators, B, 2015, 207, 724–731 CrossRef CAS.
- H. Fu, D. M. Policarpio, J. D. Batteas and D. E. Bergbreiter, Polym. Chem., 2010, 1, 631–633 RSC.
- P. Bawa, V. Pillay, Y. E. Choonara and L. C. d. Toit, Biomed. Mater., 2009, 4, 022001 CrossRef PubMed.
- P. Wust, B. Hildebrandt, G. Sreenivasa, B. Rau, J. Gellermann, H. Riess, R. Felix and P. M. Schlag, Lancet Oncol., 2002, 3, 487–497 CrossRef CAS PubMed.
- J. Akimoto, M. Nakayama, K. Sakai and T. Okano, Biomacromolecules, 2009, 10, 1331–1336 CrossRef CAS PubMed.
- F. Kohori, K. Sakai, T. Aoyagi, M. Yokoyama, M. Yamato, Y. Sakurai and T. Okano, Colloids Surf., B, 1999, 16, 195–205 CrossRef CAS.
- D. E. Meyer, B. C. Shin, G. A. Kong, M. W. Dewhirst and A. Chilkoti, J. Controlled Release, 2001, 74, 213–224 CrossRef CAS PubMed.
- M. Stubbs, P. M. J. McSheehy, J. R. Griffiths and C. L. Bashford, Mol. Med. Today, 2000, 6, 15–19 CrossRef CAS PubMed.
- A. K. Varkouhi, M. Scholte, G. Storm and H. J. Haisma, J. Controlled Release, 2011, 151, 220–228 CrossRef CAS PubMed.
- J. Liu, Y. Huang, A. Kumar, A. Tan, S. Jin, A. Mozhi and X. J. Liang, Biotechnol. Adv., 2014, 32, 693–710 CrossRef CAS PubMed.
- Y. Dong, J. Yang, H. Liu, T. Wang, S. Tang, J. Zhang and X. Zhang, Theranostics, 2015, 5, 890–904 CrossRef CAS PubMed.
- J. Z. Du, X. J. Du, C. Q. Mao and J. Wang, J. Am. Chem. Soc., 2011, 133, 17560–17563 CrossRef CAS PubMed.
- M. Huang, K. Zhao, L. Wang, S. Lin, J. Li, J. Chen, C. Zhao and Z. Ge, ACS Appl. Mater. Interfaces, 2016, 8, 11226–11236 CAS.
- J. Li, Y. Han, Q. Chen, H. Shi, S. u. Rehman, M. Siddiq, Z. Ge and S. Liu, J. Mater. Chem. B, 2014, 2, 1813–1824 RSC.
- S. Kashyap, N. Singh, B. Surnar and M. Jayakannan, Biomacromolecules, 2016, 17, 384–398 CrossRef CAS PubMed.
- Q. Jin, T. Cai, H. Han, H. Wang, Y. Wang and J. Ji, Macromol. Rapid Commun., 2014, 35, 1372–1378 CrossRef CAS PubMed.
- L. Meng, W. Huang, D. Wang, X. Huang, X. Zhu and D. Yan, Biomacromolecules, 2013, 14, 2601–2610 CrossRef CAS PubMed.
- R. Cheng, F. Meng, C. Deng, H. A. Klok and Z. Zhong, Biomaterials, 2013, 34, 3647–3657 CrossRef CAS PubMed.
- Y. Zhang, C. Wang, C. Xu, C. Yang, Z. Zhang, H. Yan and K. Liu, Chem. Commun., 2013, 49, 7286–7288 RSC.
- K. Zhou, Y. Wang, X. Huang, K. Luby-Phelps, B. D. Sumer and J. Gao, Angew. Chem., Int. Ed., 2011, 50, 6109–6114 CrossRef CAS PubMed.
- S. Perrier, P. Takolpuckdee and C. A. Mars, Macromolecules, 2005, 38, 2033–2036 CrossRef CAS.
- M. Heskins and J. E. Guillet, J. Macromol. Sci., Chem., 1968, 2, 1441–1455 CrossRef CAS.
- Y. Hiruta, M. Shimamura, M. Matsuura, Y. Maekawa, T. Funatsu, Y. Suzuki, E. Ayano, T. Okano and H. Kanazawa, ACS Macro Lett., 2014, 3, 281–285 CrossRef CAS.
- A. Yamada, Y. Hiruta, J. Wang, E. Ayano and H. Kanazawa, Biomacromolecules, 2015, 16, 2356–2362 CrossRef CAS PubMed.
- Y. Hiruta, R. Nemoto and H. Kanazawa, Colloids Surf., B, 2017, 153, 2–9 CrossRef CAS PubMed.
- Y. Hiruta, Y. Nagumo, A. Miki, T. Okano and H. Kanazawa, RSC Adv., 2015, 5, 73217–73224 RSC.
- M. Wilhelm, C. L. Zhao, Y. Wang, R. Xu, M. A. Winnik, J. L. Mura, G. Riess and M. D. Croucher, Macromolecules, 1991, 24, 1033–1040 CrossRef CAS.
- S. Tang, Q. Yin, Z. Zhang, W. Gu, L. Chen, H. Yu, Y. Huang, X. Chen, M. Xu and Y. Li, Biomaterials, 2014, 35, 6047–6059 CrossRef CAS PubMed.
- J.-H. Ryu, R. T. Chacko, S. Jiwpanich, S. Bickerton, R. P. Babu and S. Thayumanavan, J. Am. Chem. Soc., 2010, 132, 17227–17235 CrossRef CAS PubMed.
- O. A. Kucherak, S. Oncul, Z. Darwich, D. A. Yushchenko, Y. Arntz, P. Didier, Y. Mély and A. S. Klymchenko, J. Am. Chem. Soc., 2010, 132, 4907–4916 CrossRef CAS PubMed.
Footnotes |
† Electronic supplementary information (ESI) available: Cell viabilities, and 1H NMR spectra of polymers. See DOI: 10.1039/c7ra03579a |
‡ Present address: Department of Applied Chemistry, Faculty of Science and Technology, Keio University, 3-14-1, Hiyoshi, Kohoku-ku, Yokohama 223-8522, Japan. |
|
This journal is © The Royal Society of Chemistry 2017 |
Click here to see how this site uses Cookies. View our privacy policy here.