DOI:
10.1039/C7RA06822K
(Paper)
RSC Adv., 2017,
7, 38452-38457
Low-cost solution-processed digenite Cu9S5 counter electrode for dye-sensitized solar cells†
Received
19th June 2017
, Accepted 31st July 2017
First published on 7th August 2017
Abstract
The development of low-cost alternatives to the commonly used but expensive platinum (Pt) catalyst in dye-sensitized solar cells (DSSCs) is important from a commercial point of view. In this work, Cu9S5 nanocrystalline film is fabricated directly onto a F-doped SnO2 (FTO) substrate by a solution-processed spin-coating method with low temperature post-treatment at 250 °C and it is further explored as a counter electrode (CE) material in DSSCs. The results from cyclic voltammetry (CV) and electrochemical impedance spectroscopy (EIS) disclose that Cu9S5 film exhibits a higher catalytic ability for the state-of-the-art cobalt(II/III) tris(bipyridyl) ([Co(bpy)3]2+/3+) redox system as compared to the widely used iodine-based electrolyte. Consequently, the DSSC devices based on the cobalt complex redox shuttles show a power conversion efficiency (PCE) of 5.7% measured at 100 mW cm−2 illumination (AM 1.5G), which is substantially higher than that of the iodine-based counterpart (3.9%). This has been the first presentation for the application of digenite copper sulfides as an electrocatalyst for the [Co(bpy)3]2+/3+ redox system in DSSCs. The present finding represents a promising solution for the development of alternative cost-effective CE materials for DSSCs in the future.
1. Introduction
Dye-sensitized solar cells (DSSCs) have been widely studied as promising next-generation solar cells since 1991, owing to their low production cost, ease of manufacture, and comparatively high PCE.1 A DSSC device typically consists of three major parts: a mesoporous TiO2 photoanode loaded with a dye molecule, a liquid electrolyte typically involving an iodide/triiodide (I−/I3−) redox couple and a CE. After light absorption, the oxidized dye transfers electrons into the TiO2 semiconductor. The dye molecule cations are then regenerated by iodide, while the CE reduces the triiodide back to iodide at the CE/electrolyte interface.
Through intense investigations over the past twenty years, remarkable improvements have been achieved in the research field of DSSCs. A big breakthrough was made by replacing the I−/I3− redox system with a [Co(bpy)3]2+/3+ redox couple, which possesses a more positive redox potential, thus giving a much higher open-circuit voltage (Voc) and higher PCEs.2,3 The highest PCE of DSSCs has reached ∼13% by using [Co(bpy)3]2+/3+ system in combination with panchromatic co-photosensitizers.4
CE acts as a catalyst for the regeneration of the redox couple, which is an indispensable part in DSSCs and is the key for determining the overall performance.5 Noble metal Pt was extensively applied as CE catalyst material since the very beginning of DSSC research, because of the excellent catalytic ability for the I3− reduction and good electrical conductivity. Nevertheless, Pt is rarely reserved in the earth and the fabrication process typically involves high temperature, which limit its large-scale application in the future. Searching for a low-cost alternative CE catalyst is therefore absolutely necessary.
To replace Pt CE, a number of alternative materials has been studied, for instance, carbon-based materials,6 conducting polymers7 as well as metal complexes.8 Metal sulfides, such as CoS, NiS, FeS2, WS2, and MoS2, have also been developed as alternative CEs, showing comparable or even higher PCEs relative to the Pt-based counterparts.9–17 Among the family of metal sulfides, several copper sulfide compounds, which showed good catalytic property, high conductivity, as well as economical fabrication, have attracted much attention in quantum dot-sensitized solar cells and thin film solar cells.18,19 However, the use of copper sulfide as a CE material in DSSCs has been rarely reported until now. Lam and co-workers reported a copper sulfide-based CE fabricated from precursor decomposition method involving a high temperature (500 °C) sintering process. It showed a 3.79% PCE for the I−/I3− redox system.20
In this work, Cu9S5 nanocrystalline film was fabricated directly onto the FTO substrate by a solution-processed spin-coating technique with a low temperature post-treatment at 250 °C and it was further explored as a CE material in DSSCs. The CV and EIS results revealed that Cu9S5 film exhibited a higher electrocatalytic activity for the cobalt-based system relative to the iodine-based electrolyte. Consequently, the DSSC devices based on the cobalt complex redox shuttles showed a PCE of 5.7% at 100 mW cm−2 illumination (AM 1.5G), which was considerably higher than that of iodine-based electrolyte (3.9%).
2. Experimental
2.1. Materials
Chemicals and solvents, including tert-butanol (96%), 4-tert-butylpyridine (TBP, 96%), li-bis(trifluoromethanesulfonyl)imide (Li-TFSI), copper chloride (CuCl2), thioacetamide (TAA), and N,N-dimethylformamide (DMF) were purchased from Sigma-Aldrich. Sensitizer LEG4 was purchased from Dyenamo AB, Sweden. Mesoporous TiO2 paste and scatting layer TPP200 paste were provided by Heptachroma, China.
2.2. Preparation of CEs
FTO conducting glass substrates were washed by detergent, deionized water, and ethanol. Then, the substrates were further treated by UVO-Cleaner for 30 min. The preparation of the precursor solutions as follows:19 an equiv. of CuCl2 was dispersed in DMF by ultrasound to dissolve. Then 4 equiv. of TAA was added and stirred for 2 hours until a blood red precursor solution was obtained. The solution was filtered before use. The digenite Cu9S5 CE was prepared by spin-coating of the precursor solution on a freshly cleaned FTO substrate at a speed of 2000 rpm for 30 s. After being sintered at 250 °C on hot plate for 1 min, Cu9S5 counter electrode was obtained.
2.3. Fabrication of DSSC devices
The DSSC devices were fabricated as reported previously.21 The well cleaned FTO substrate was soaked in 40 mM TiCl4 aqueous solution at 70 °C for 30 min, and then sintered at 500 °C for 30 min to fabricate the compact TiO2 blocking layer. The mesoporous layer and scattering layer were fabricated via screen-printing of TiO2 18NR-T paste (three times, every time dried at 130 °C for 5 min) and TPP200 paste, respectively. After sintering (500 °C, 30 min), the films were dipped into TiCl4 aqueous solution again. The final TiO2 film was soaked in a LEG4 dye solution (1 × 10−4 M) in acetonitrile and tert-butanol mixed solvent (1
:
1, v/v) overnight. DSSCs with the Cu9S5 counter electrode and the photoanode TiO2 electrode were assembled using a surlyn film (25 μm) as a spacer. Different electrolytes were injected into interlayer through a pinhole. The symmetrical dummy cells were fabricated by two identical counter electrodes (CE/electrolytes/CE), and the electrolytes were the same as used for the DSSCs devices. The iodine-based electrolyte was the same as previously reported.21 The iodine-based electrolyte consisted of 0.60 M 1,2-dimethyl-3-propylimidazolium iodide (DMPII), 0.02 M I2, 0.06 M LiI, and 0.40 M TBP in acetonitrile. The [Co(bpy)3]2+/3+ electrolyte was made of 0.22 M [Co(bpy)3](PF6)2, 0.05 M [Co(bpy)3](PF6)3, 0.10 M Li-TFSI, and 0.50 M TBP in acetonitrile.22
2.4. Characterizations and measurements
XRD was measured with an automatic X-ray powder diffractometer (D/Max 2400, Rigaku, Japan). X-ray photon spectroscopy (XPS, Escalab 250Xi, Thermo Fisher) was implemented to measure the binding energies of digenite Cu9S5 film. The morphology and structure of the Cu9S5 CE was obtained by field-emission scanning electron microscopy (FE-SEM, Nova Nano SEM 450, USA) and atomic force microscopy (AFM, XE-70, Korea). The electrochemical characterizations of Cu9S5 CE were performed by electrochemical workstation (CHI660E, Chenhua, Shanghai). It was conducted using a three-electrode system with as-prepared Cu9S5 film on FTO as a working electrode. Pt wire electrode and Ag/AgNO3 were used as counter electrode and a reference electrode, respectively. The cobalt complex-based solution is composed of 20 mM [Co(bpy)3](PF6)2, 5 mM [Co(bpy)3](PF6)3, and 100 mM LiClO4 in acetonitrile. The iodine-based solution contained 10 mM LiI, 1 mM I2, and 100 mM LiClO4 in acetonitrile. The active area of working electrode was controlled at 1 cm2 and the scan rate was 50 mV s−1. EIS was obtained by using a Zennium analyzer system (Model: IM6, Zahner, German) with a frequency range from 106 Hz to 0.1 Hz. The photovoltaic performance were determined by photocurrent density–voltage (J–V) curves and incident photon-to-current conversion efficiency (IPCE) spectra. The test instruments include a Keithley 2400 Source-measure with an Oriel Sol3A solar simulator (Model: 94023A, Newport USA) and Hypermono-light (SM-25, Japan).
3. Results and discussion
3.1. Composition and morphology
Fig. 1 displays the XRD patterns of Cu9S5 film on FTO (red line) and a bare FTO (black line) for reference. It indicates that Cu9S5 was successfully in situ grown on the FTO substrate. A sharp diffraction peak at position 46.2° corresponds to the (0120) plane, indicative of the good crystallization of as-prepared Cu9S5 film. The peaks observed at diffraction angles of 27.8°, 32.3° and 54.9° correspond to the (0015), (1010), (1115) crystal planes, respectively, in a good line with the reported data (JCPD card, no. 47-1748) and previous report.19 XPS was carried out to further confirm the structure of Cu9S5 film on the FTO substrate. The peaks at binding energy energies of 931.95 and 932.85 eV in Fig. S1a† are attributed to Cu 2P peaks of the Cu+ and Cu2+. The double peaks at about 162 eV in Fig. S1b† correspond to the S 2P peaks of S2−. These results agree well with characteristics of Cu9S5 as previously reported.19
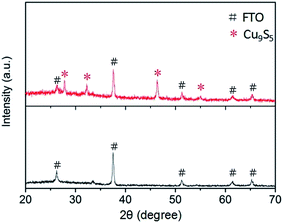 |
| Fig. 1 XRD spectra of Cu9S5 film on FTO (red line) and bare FTO (black line). | |
Fig. 2a and b exhibit the FE-SEM results of Cu9S5 film with different magnifications. A nanoporous network is clearly observed for the as-prepared film. Large specific surface area is expected to deliver more catalytic active sites, and thus enhancing the CE's electrocatalytic ability.9 Moreover, the nanoporous structure is also beneficial for the diffusion of the electrolytes.23 The energy-dispersive X-ray spectroscopy (EDS) elemental mappings show uniform distributions of elements of Cu and S grown within the film,24 as presented in Fig. S2.† AFM was performed to evaluate the surface three-dimensional morphology, as depicted in Fig. 2c. The root-mean-square roughness (RMS) of Cu9S5 film is 74.47 nm, which is much larger than that of Pt (RMS = 8.82 nm) (Fig. S3†). The result indicates that Cu9S5 film has unsmooth surface, which is consistent with the SEM images. Here, we conclude that well-crystallized digenite Cu9S5 film with high surface area was in situ grown on the FTO glass through a low-temperature solution-processable method from a simple precursor solution.
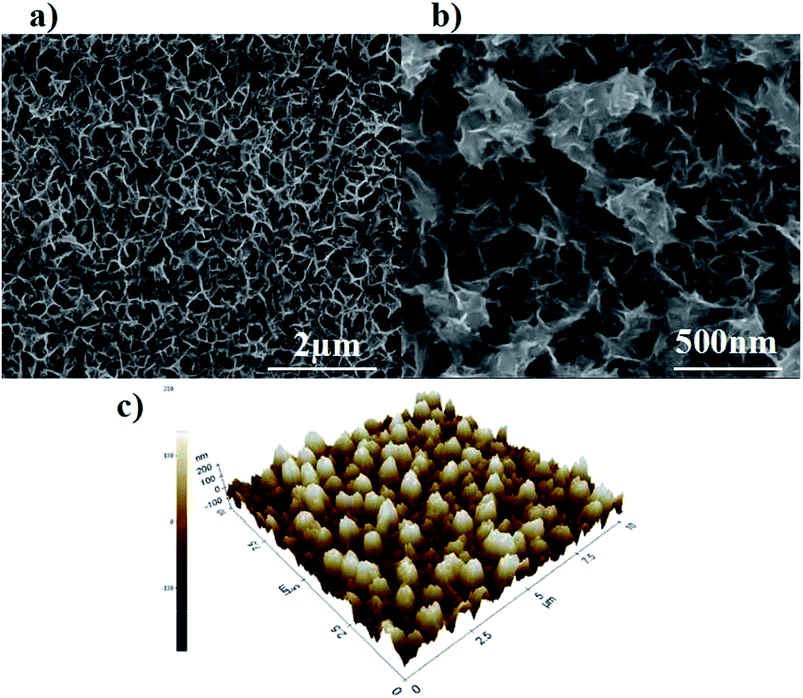 |
| Fig. 2 (a and b) FE-SEM images of Cu9S5 film on FTO substrate at different magnifications; (c) AFM image of Cu9S5 film on FTO substrate. | |
3.2. Electrochemical properties
To explore the electrocatalytic ability of the Cu9S5 CE for both the iodine- and cobalt-based redox electrolytes, CV measurement was performed. It was conducted using a three-electrode system with as-prepared Cu9S5 film on FTO as a working electrode. Pt wire electrode and Ag/AgNO3 were used as counter electrode and a reference electrode, respectively. The cobalt complex-based solution is composed of 20 mM [Co(bpy)3](PF6)2, 5 mM [Co(bpy)3](PF6)3, and 100 mM LiClO4 in acetonitrile. The iodine-based solution contained 10 mM LiI, 1 mM I2, and 100 mM LiClO4 in acetonitrile. Generally, the catalytic activities was determined by the peak current density and the peak distance between the oxidation peak and reduction peak (Epp).25 For a CE, large peak current density and small Epp are two important parameters to determine high electrocatalytic ability of a CE catalyst.24 Fig. 3a depicts the CV of Cu9S5 electrode in cobalt complex-based electrolyte. A redox event is clearly observed in the CV curve (Fig. 3a), which is assigned to the redox reaction of Co3+ + e− ↔ Co2+. In DSSCs, the dye cations are regenerated by Co2+ cations, which are oxidized to Co3+ cations afterwards. The resulting Co3+ cations are reduced back to Co2+ cations at CE/electrolyte interface. Consequently, the reduction peak should be the research emphasis for the CV study.26 In the CV curve, Cu9S5 electrode exhibits a high reduction peak current density (JRE) of 0.85 mA cm−2 and an Epp of 272 mV, which are close to the corresponding value obtained for Pt electrode (JRE 1.0 mA cm−2, Epp 219 mV) (Fig. S4†). This result indicates that the Cu9S5 film is expected to have comparable catalytic activity for the reduction of Co3+ cations complex relative to Pt. The high surface of the nanoporous Cu9S5 electrode most likely provides a more reduction sites, thus enhancing catalytic ability. It is also worth mentioning that the JRE of Cu9S5 CE remained almost unchanged after 50 cycles as shown in Fig. S4,† indicating the good electrochemical stability of Cu9S5 electrode for the reduction of cobalt(III) species. The electrocatalytic ability of the Cu9S5 CE for the iodine-based electrolyte was also studied and the CV curve is depicted in Fig. 3b. By stark contrast, for the iodine-based redox couple, the Cu9S5 CE exhibited two pairs of irreversible redox peaks. In particular, the weak cathodic peak at the left part of the CV curve is attributed to the reduction of the I3− ions (I3− + 2e− → 3I−). This result implies the poor electrocatalytic activity of Cu9S5 electrode towards the reduction of I3− ions.
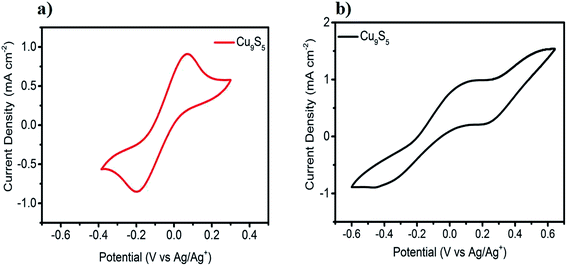 |
| Fig. 3 Cyclic voltammograms for prepared Cu9S5 electrode: (a) cobalt complex-based electrolyte in acetonitrile solution of 20 mM [Co(bpy)3](PF6)2, 5 mM [Co(bpy)3](PF6)3, and 100 mM LiClO4; (b) iodine-based electrolyte in acetonitrile solution of 10 mM LiI, 1 mM I2, and 100 mM LiClO4. | |
EIS measurements were performed with an aim to further examine the electrocatalytic property of Cu9S5 electrode in different electrolytes by using a dummy cell with two identical electrodes (CE/Electrolyte/CE). Fig. 4a presents the Nyquist plots of the [Co(bpy)3]2+/3+ and I−/I3− redox electrolytes measured at a bias voltage of 0.3 V under dark condition. The equivalent circuit model is presented in the inset. For a better comparison, the enlarged part of the Nyquist plots is also given (Fig. 4b). Two semicircles can be observed for these two redox systems. The high-frequency one corresponds to the charge-transfer resistance (RCT) at the CE/electrolyte interface. The semicircle observed in the low-frequency range reflects the Nernst diffusion impedance (ZN) of the redox couples in the electrolyte.16 The RCT of the cobalt-based redox couple is estimated to be 12.3 Ω, which is significantly lower than that of I−/I3− (422 Ω). The smaller RCT further indicates that Cu9S5 electrode shows good electrocatalytic ability towards the reduction of Co3+ species, which is in a good line with the CV curves. The EIS of Pt CE under the same conditions were also tested for comparison as presented in Fig. S5.† For the cobalt-based system, the RCT value of Pt CE is estimated to be 7.2 Ω, which is slightly smaller relative to Cu9S5 based CE. Here, it emphasizes again that Cu9S5 CE has good electrocatalytic activity towards the reduction of the Co3+ species, which can rival the state-of-the-art Pt electrode. To further study the electrocatalytic activities of Cu9S5 CE for different electrolytes, Tafel polarization curves were conducted using symmetric cell as in EIS. Fig. S6† shows the Tafel polarization curves of the Cu9S5 electrode with different electrolytes in the potential range from −0.6 to +0.6 V. For the iodine-based electrolyte, the plot is not a normal smooth line and the exchange current density is much lower than that of cobalt-based electrolyte. These results further verifies that Cu9S5 film is not a suitable catalytic material for the I−/I3− redox system. Overall, from the CV, EIS and Tafel results, we infer that the Cu9S5 CE would be a potential counter electrode for cobalt complex-based electrolytes in DSSCs.
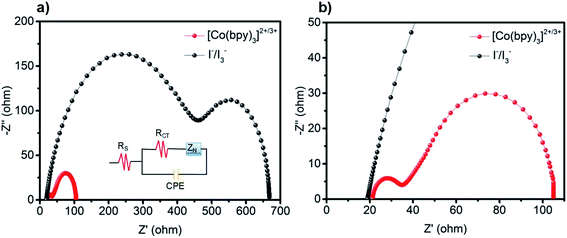 |
| Fig. 4 (a) Nyquist plots of the prepared Cu9S5 electrode in a dummy cell with [Co(bpy)3]2+/3+ and I−/I3− based electrolytes, the inset is the equivalent circuit used to model the impedance spectra; (b) enlarged Nyquist plots of (a). | |
3.3. Photovoltaic performance of DSSCs
We further examined photovoltaic performance of Cu9S5 CEs in DSSCs using cobalt complex- and iodine-based redox electrolytes. The photocurrent density–voltage (J–V) characteristics of the best solar cells measured at 100 mW cm−2 illumination AM 1.5G (active area 0.16 cm2) are displayed in Fig. 5a and the corresponding parameters are summarized in Table 1. The DSSC device based on [Co(bpy)3]2+/3+ system exhibited an open-circuit voltage (Voc) of 0.78 V, a short-circuit current density (Jsc) of 13.7 mA cm−2, and a fill factor (FF) of 0.53, giving a PCE of 5.7%. By comparison, all the photovoltaic parameters for the I−/I3− system are lower than the corresponding values of the cobalt complex counterpart. DSSC device used I−/I3− electrolyte showed a much lower PCE of only 3.9%, with a Voc of 0.69 V, a Jsc of 12.3 mA cm−2, and a FF of 0.46. Strikingly, the cobalt-based electrolyte displayed a comparatively higher FF (0.53) as compared to the I−/I3− redox system (0.46). This should be attributed to a significantly lower RCT observed for the cobalt-based redox couple as confirmed by the EIS measurements discussed above. Generally, Voc is mainly governed by the potential gap between the Fermi energy level of the TiO2 and the redox potential of the redox mediator in the electrolyte. From the previous report, the redox potential of [Co(bpy)3]2+/3+ is 0.56 V vs. NHE, while the corresponding value of the I−/I3− is 0.35 V vs. NHE.21 Thus, the difference for the Voc values observed should be mainly attributed to more positive redox potential of the cobalt complexes. Fig. 5b shows the IPCE spectra of DSSCs based on these two redox electrolytes. The trend of IPCE measurements is in good agreement with Jsc obtained from J–V characterizations. The photovoltaic performance of Pt CE, as a reference, was also tested for the [Co(bpy)3]2+/3+ based electrolyte under identical conditions (Fig. S7†). The solar cell based on Pt CE exhibited a PCE of 8.5% with an improved FF (0.67). The different performances of Pt and Cu9S5 CEs most likely lies in the fact that Cu9S5 CE has a low intrinsic conductivity and thus a larger charge transfer resistance.
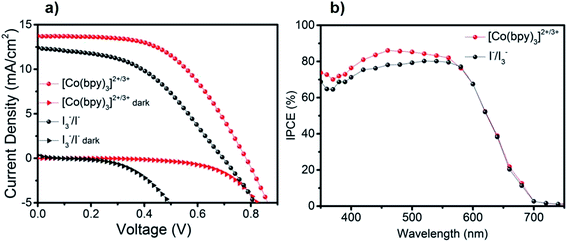 |
| Fig. 5 (a) Photocurrent density–voltage (J–V) curves of DSSCs using Cu9S5 CE with [Co(bpy)3]2+/3+ and I−/I3− based electrolytes measured under AM 1.5G simulated sunlight (100 mW cm−2) and in dark condition; (b) the incident photon-to-current conversion efficiency (IPCE) spectra of DSSCs using Cu9S5 CE with [Co(bpy)3]2+/3+ and I−/I3− based electrolytes. | |
Table 1 Photovoltaic parameters of DSSCs based on Cu9S5 counter electrode using [Co(bpy)3]2+/3+ and I−/I3− based electrolytes measured under AM 1.5G simulated sunlight (100 mW cm−2)
Redox couples |
Voc (V) |
Jsc (mA cm−2) |
FF |
PCE (%) |
[Co(bpy)3]2+/3+ |
0.78 |
13.7 |
0.53 |
5.7 |
I−/I3− |
0.69 |
12.3 |
0.46 |
3.9 |
4. Conclusions
In conclusion, we developed a Cu9S5 nanocrystalline film, which was fabricated directly onto the FTO substrate by a low temperature solution-processed method, as a low-cost CE material in DSSCs. The results from CV and EIS measurements demonstrated that Cu9S5 film displayed a higher electrocatalytic ability for the state-of-the-art [Co(bpy)3]2+/3+ redox system in comparison to the widely used iodine-based electrolyte. Consequently, DSSC devices incorporating cobalt complex redox shuttles showed a considerably higher PCE, which is attributed mainly to the low RCT at the CE/electrolyte interface and good catalytic ability of Cu9S5 electrode towards reduction of the cobalt(III) species. There is still a potential room for further enhancement of the efficiency of Cu9S5 CE. One possible strategy would be to couple it with highly conductive carbon materials, as demonstrated by several successful examples reported previously.24,27 Nevertheless, the present work will shed interesting light on identifying alternative low-cost and efficient CE materials, in particular for non-iodine based electrolytes in DSSCs in the future.
Acknowledgements
We acknowledge the financial support from the National Natural Science Foundation of China (21606039, 21673028, 51661135021, 91233201), the Fundamental Research Funds for the Central Universities, Swedish Foundation for Strategic Research (SSF), the Swedish Energy Agency, as well as the Knut and Alice Wallenberg Foundation.
References
- B. O'regan and M. Grätzel, Nature, 1991, 353, 737–740 CrossRef.
- S. M. Feldt, E. A. Gibson, E. Gabrielsson, L. Sun, G. Boschloo and A. Hagfeldt, J. Am. Chem. Soc., 2010, 132, 16714–16724 CrossRef CAS PubMed.
- A. Yella, H. W. Lee, H. N. Tsao, C. Yi, A. K. Chandiran, M. K. Nazeeruddin, E. W.-G. Diau, C.-Y. Yeh, S. M. Zakeeruddin and M. Grätzel, Science, 2011, 334, 629–633 CrossRef CAS PubMed.
- S. Mathew, A. Yella, P. Gao, R. Humphry-Baker, B. F. E. Curchod, N. Ashari-Astani, I. Tavernelli, U. Rothlisberger, M. K. Nazeeruddin and M. Grätzel, Nat. Chem., 2014, 6, 242–247 CrossRef CAS PubMed.
- M. Wu and T. Ma, J. Phys. Chem. C, 2014, 118, 16727–16742 CAS.
- M. Wu, X. Lin, T. Wang, J. Qiu and T. Ma, Energy Environ. Sci., 2011, 4, 2308–2315 CAS.
- H. N. Tsao, J. Burschka, C. Yi, F. Kessler, M. K. Nazeeruddin and M. Grätzel, Energy Environ. Sci., 2011, 4, 4921–4924 CAS.
- J. Briscoe and S. Dunn, Adv. Mater., 2016, 28, 3802–3813 CrossRef CAS PubMed.
- M. Wang, A. Anghel, B. Marsan, N. Ha, N. Pootrakulchote, S. Zakeeruddin and M. Grätzel, J. Am. Chem. Soc., 2009, 131, 15976–15977 CrossRef CAS PubMed.
- C. Kung, H. Chen, C. Lin, K. Huang, R. Vittal and K. Ho, ACS Nano, 2012, 6, 7016–7025 CrossRef CAS PubMed.
- H. Sun, D. Qin, S. Huang, X. Guo, D. Li, Y. Luo and Q. Meng, Energy Environ. Sci., 2011, 4, 2630–2637 CAS.
- D. Y. Wang, Y. T. Jiang, C. C. Lin, S. S. Li, Y. T. Wang, C. C. Chen and C. W. Chen, Adv. Mater., 2012, 24, 3415–3420 CrossRef CAS PubMed.
- Y. C. Wang, D. Y. Wang, Y. T. Jiang, H. A. Chen, C. C. Chen, K. C. Ho, H. L. Chou and C. W. Chen, Angew. Chem., Int. Ed., 2013, 52, 6694–6698 CrossRef CAS PubMed.
- Y. Wang, S. Li, Y. Bai, Z. Chen, Q. Jiang, T. Li and W. Zhang, Electrochim. Acta, 2013, 114, 30–34 CrossRef CAS.
- E. Singh, K. S. Kim, G. Y. Yeom and H. S. Nalwa, ACS Appl. Mater. Interfaces, 2017, 9, 3223–3245 CAS.
- N. Huang, G. Li, Z. Xia, F. Zheng, H. Huang, W. Li, C. Xiang, Y. Sun, P. Sun and X. Sun, Electrochim. Acta, 2017, 235, 182–190 CrossRef CAS.
- S. Infant Raj, X. Xu, W. Yang, F. Yang, L. Hou and Y. Li, Electrochim. Acta, 2016, 212, 614–620 CrossRef.
- M. Venkata-Haritha, C. V. V. M. Gopi, Y. S. Lee and H. J. Kim, RSC Adv., 2016, 6, 101185–101197 RSC.
- M.
J. Zhang, Q. Lin, X. Yang, Z. Mei, J. Liang, Y. Lin and F. Pan, Nano Lett., 2016, 16, 1218–1223 CrossRef CAS PubMed.
- H. K. Mulmudi, S. K. Batabyal, M. Rao, R. R. Prabhakar, N. Mathews, Y. M. Lam and S. G. Mhaisalkar, Phys. Chem. Chem. Phys., 2011, 13, 19307–19309 RSC.
- J. Li, X. Yang, Z. Yu, G. G. Gurzadyan, M. Cheng, F. Zhang, J. Cong, W. Wang, H. Wang, X. Li, L. Kloo, M. Wang and L. Sun, RSC Adv., 2017, 7, 4611–4615 RSC.
- H. N. Tsao, C. Yi, T. Moehl, J. H. Yum, S. M. Zakeeruddin, M. K. Nazeeruddin and M. Grätzel, ChemSusChem, 2011, 4, 591–594 CrossRef CAS PubMed.
- M. Wu, X. Lin, Y. Wang, L. Wang, W. Guo, D. Qi, X. Peng, A. Hagfeldt, M. Grätzel and T. Ma, J. Am. Chem. Soc., 2012, 134, 3419–3428 CrossRef CAS PubMed.
- Z. Xie, X. Cui, W. Xu and Y. Wang, Electrochim. Acta, 2017, 229, 361–370 CrossRef CAS.
- H. Li, Y. Xiao, G. Han and W. Hou, J. Power Sources, 2017, 342, 709–716 CrossRef CAS.
- X. Yin, F. Wu, N. Fu, J. Han, D. Chen, P. Xu, M. He and Y. Lin, ACS Appl. Mater. Interfaces, 2013, 5, 8423–8429 CAS.
- M. Guo, F. Zhao, Y. Yao, S. Wang and S. Yin, Electrochim. Acta, 2016, 205, 15–19 CrossRef CAS.
Footnote |
† Electronic supplementary information (ESI) available. See DOI: 10.1039/c7ra06822k |
|
This journal is © The Royal Society of Chemistry 2017 |
Click here to see how this site uses Cookies. View our privacy policy here.