DOI:
10.1039/C7RA07095K
(Paper)
RSC Adv., 2017,
7, 40990-40995
Enhancement of bacterial growth with the help of immiscible oxygenated oils
Received
26th June 2017
, Accepted 14th August 2017
First published on 22nd August 2017
Abstract
Bacterial growth in an aqueous medium in the vicinity of the interface with an immiscible oxygenated fluid is a subject of this study. We analyse the impact of fluids with oxygen solubility much higher than that of water, on the growth rate of Escherichia coli in shaken simple culture tubes. The measurements were conducted continuously in an incubator with the use of a custom-designed detector of scattered light. As a result, we show that oxygenated immiscible fluids transfer oxygen across the interfaces to enhance the maximum growth rate of bacteria, in some cases even to double it. In the present study, we probed six fluids, including hexadecane, silicone oil, fluorinated liquids: FC-40, FC-70, and hydrofluoroethers (HFE): HFE-7200 and HFE-7500. The mechanisms of interfacial phenomena of physio-chemical and hydrodynamic nature have been elucidated.
Introduction
The rapid growth of bacteria is now essential in many branches of industry, medicine and pharmacology. The food industry uses bacteria in production and preservation,1 and especially in the proliferation of probiotic bacteria.2 Medicine searches for a rapid means of pathogen detection and fitting an appropriate antibiotic at a proper concentration to provide to a patient.3,4 Currently, the latter is even more significant than identification of the particular microorganisms. Pharmacology produces specific proteins and antibodies utilizing genetically modified bacteria.5,6
Oxygen supply to bacterial growth is a well-known and investigated topic during the last, at least, 50 years.7–11 Special incubators providing enhanced oxygenation of a culture medium are employed to improve the growth of bacteria and the biomass. Oxygen is the main substrate whose transport is crucial in the aerobic culture.12,13 For this purpose, one applies liquid/liquid10,14,15 or gas/liquid10,16,17 bioreactors to increase the oxygen transfer capabilities. Recently, the use of organic solvents,18 membranes,19,20 surfactants21 or particles22 to improve oxygen transfer between phases in the complex systems have also been reported. The most popular groups of compounds used in these applications are hydrocarbon oils, fluorinated fluids, and hydrofluoroethers. These fluids characterise themselves with high oxygen solubility and biocompatibility.23,24
On the other hand, we have to remember that the rapid stochastic growth of bacteria is limited by general relationship concerning heat, self-organization and durability of bacteria.25 It means that thermodynamics of a bacterial division process imposes certain constraints on self-replication. From this point of view, the limitation of the doubling time of Escherichia coli to ca. 20 minutes in ideal conditions is justified. Other strains, such as thermophilic anaerobic bacteria, show the doubling time of ca. 10 minutes.26 Similarly, Vibrio natriegens has dividing twice as fast as Escherichia coli.27 So, the doubling time and the amount of biomass of bacteria depend on the strain of bacteria as well as on the environment and conditions under which microorganisms are growing.
A need for another approach
The primary objective of this research has been to find the best oil as a continuous phase to apply in microfluidics.28–30 In these applications, the continuous phase can be supplied to a microfluidic chip from a container which is under pressure of, e.g., pure oxygen.31 In addition, the growth of bacterial culture in microfluidic droplets is fast.32 Reported growth rates are among the most optimal for a given strain.31,33 These phenomena have not yet been thoroughly investigated. Therefore, the approach presented herein does not optimise the mixing, sparkling or bubbling gas or oil, nor considering the destructive action of free radicals, which is increased as a result of excess oxygen.34 We focus on the simplest gas exchange at the free interface between the bacteria medium and the tested oil to find the best continuous phase for applications in microfluidics.
Results
The growth of bacteria in oxygenated immiscible fluids
For investigations, we chose fluids that are biocompatible and exhibit high oxygen solubility. These are: hexadecane, silicone oil, FC-40, FC-70, HFE-7200 and HFE-7500. Prior to the experiment, each of the fluids is aerated twelve hours with the use of pure oxygen. Next, we pour 20 mL of inoculated Escherichia coli culture medium and 20 mL of one of the tested liquids to the culture tubes. Hexadecane and silicone oil are less dense than water; as a result, the culture media are afloat (Fig. 1b). In turn, FC-40, FC-70, HFE-7200 and HFE-7500 have density almost double the density of water, so the culture media float on them (Fig. 1c). A control tube used as the reference contained only 20 mL of inoculated culture medium. In each of the ten repetitions of the experiment, seven vessels were placed on an orbital shaker (200 rpm) in a thermostatic incubator (37 °C). The light scattered by bacteria in the culture media was measured using the custom-made system every 10 minutes within 12 hours.
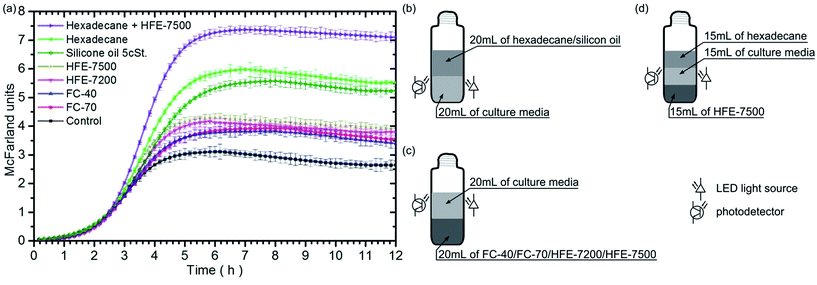 |
| Fig. 1 (a) Graph shows the temporal evolution of scattered light intensity observed during bacterial growth (expressed in McFarland units) in culture media tubes containing different oxygenated oils. The marked standard deviations were calculated for ten independent experiments. (b–d) Schematic illustration of the cultivation of E. coli bacteria in medium: (b) in the vicinity of hexadecane or silicon oil, (c) in the vicinity of fluorinert/hydrofluoroether, (d) between two immiscible fluids. The LED light source and the photodetector were placed in the middle of bacteria medium. | |
Fig. 1 shows the bacterial growth curves for each fluid. The results indicate that the presence of each of the oxygenated immiscible fluids initiates a faster growth of bacteria in the nutrient as compared to the reference sample. The bacteria populations attained a stationary phase at different levels, as evidenced by the steady-state light scattering intensity. Moreover, the maximum rates of bacterial growth (see Table 1) are higher for samples containing fluids under test than that for the control sample.
Table 1 The maximum rate of bacteria growth in experiments performed with different oxygenated immiscible fluids in comparison to the control sample. The standard deviations were calculated from measurements in ten independent experiments. Abbreviation Hex stands for hexadecane
|
Control |
FC-70 |
FC-40 |
HFE-7200 |
HFE-7500 |
Silicon oil 5 cSt. |
Hexadecane |
Hex + HFE-7500 |
Max. growth rate [h−1] |
1.32 ± 0.03 |
1.37 ± 0.02 |
1.49 ± 0.02 |
1.76 ± 0.02 |
1.90 ± 0.02 |
1.76 ± 0.02 |
2.20 ± 0.02 |
3.07 ± 0.01 |
Correlation of the number of colony-forming units with the light scattering of the bacterial medium
To check, that the value of scattering measured in the tubes is proportional to the number of bacteria, we have performed the bacterial culture test using the dilution method for the selected cultures (Fig. 2). The outcomes confirmed that despite the vicinity of various oxygenated fluids, the number of bacteria in the culture continued to grow as indicated by the McFarland units. The result also indicates no changes in bacterial morphology between the various samples – that has been confirmed with the use of a microscope. Larger or smaller bacterial cells may cause a decrease or increase in measured scattering,35 but then the number of colonies for the measured OD should deviate significantly from the straight line.36
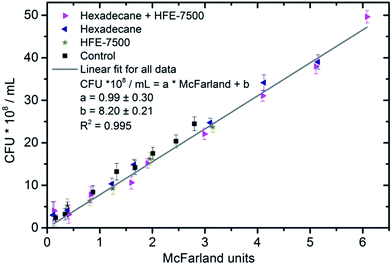 |
| Fig. 2 The correlation between the number of colony-forming units (CFU) per mL and McFarland units for various samples. Colonies were plate-counted after dilutions of bacterial culture media. | |
The growth of bacteria between two free interfaces
The next experiment was performed using four culture tubes which contained: (a) 15 mL of medium (serving as the control/reference sample), (b) 15 mL of medium and 15 mL of hexadecane, (c) 15 mL of medium and 15 mL of HFE-7500, (d) 15 mL of medium plus 15 mL of hexadecane and 15 mL of FHE-7500. From the group of fluorinated and HFE fluids, we have selected HFE-7500. Then, from the group of afloat fluids, we have selected hexadecane. These selections were made due to the highest bacterial growth rates observed during the initial experiments (see Table 1). The last sample consisted of three phases: looking from the bottom, the first was a heavy phase of HFE-7500, then, in the middle, was the bacteria medium, and finally, on top, there was a light phase of hexadecane (Fig. 1d). HFE-7500 and hexadecane do not mix with each other. They form two free interfaces: the medium/hexadecane one and the medium/HFE-7500 one. As before, the hexadecane and HFE-7500 oils were oxygenated for 12 hours before the experiment. The experiment was also repeated 10 times. The signals measured for the first three samples are comparable with those obtained in previous experiments within the limits of statistical errors. Therefore, the result obtained for the remaining three-phase sample is shown in the Fig. 1 and the Table 1 for better comparison.
The obtained results show that the growth of bacteria in medium placed between two oil phases is the fastest, with the maximum growth rate attaining 3.08 h−1 which is more than twice the rate in the control sample. Moreover, we observe the greatest increase in biomass volume under these conditions.
Dependence of the bacterial growth on the volume of oxygenated fluids
Next, we checked how the growth of bacteria depends on the amount of the employed immiscible fluid. In this case, we used independently hexadecane and HFE-7500. The volume of media in each culture sample was the same – 20 mL. The tests were performed for four volumes of oxygenated immiscible liquids: 5 mL, 10 mL, 15 mL and 20 mL. Fig. 3 represents the finding of the study.
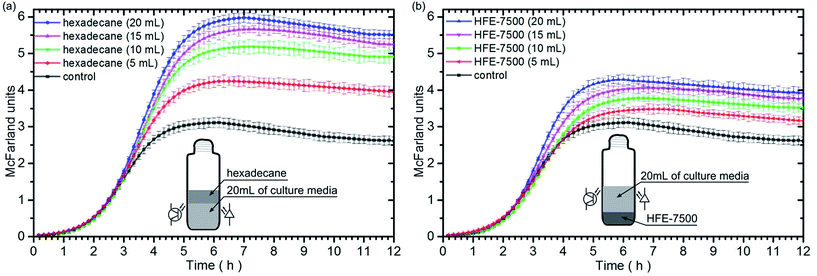 |
| Fig. 3 Temporal evolution of light scattering intensity, expressed in McFarland units, during the bacteria growth in culture media tubes for different volumes of: (a) hexadecane, (b) HFE-7500. The marked standard deviations were calculated from ten independent experiments. The sketches depict the distribution of liquids in culture tubes. The positions of the LED light source and the photodetector were marked in the middle of the bacteria medium. | |
The performed experiments show that 5 mL of the immiscible oxygenated fluid can increase the growth rate of bacteria ca. 21% and 2% while 20 mL of oil can increase the growth rate ca. 67% and 44%, for hexadecane and HFE-7500, respectively. For both liquids, the larger volume of an oxygenated fluid on the bottom or on the top of the culture media, caused an increase of the bacteria population in the stationary phase. Moreover, the growth rate increases with increasing volume of the tested fluids (Table 2).
Table 2 The maximum bacterial growth rates for different volumes of oxygenated HFE-7500 and hexadecane fluids. The standard deviations were calculated from ten independent experiments. Abbreviations: Hex – hexadecane, HFE – HFE-7500
|
Control |
Hex 20 mL |
Hex 15 mL |
Hex 10 mL |
Hex 5 mL |
HFE 20 mL |
HFE 15 mL |
HFE 10 mL |
HFE 5 mL |
Max. growth rate [h−1] |
1.32 ± 0.03 |
2.20 ± 0.02 |
2.11 ± 0.03 |
1.96 ± 0.02 |
1.60 ± 0.03 |
1.90 ± 0.02 |
1.78 ± 0.02 |
1.44 ± 0.03 |
1.35 ± 0.03 |
Discussion
The obtained results show that the presence of an oxygenated immiscible fluid in the vicinity of the culture medium causes a faster bacterial growth and leads to a much larger population of bacteria in the stationary phase. These outcomes can be interpreted with the use of the Fick's and Henry's laws, which say that the transfer of gas, in this case – oxygen, is proportional to the difference in oxygen partial pressures between liquids, and to the exchange rate at the interface. From the point of view of statistical physics, the gas exchange should take place until the balance of chemical potentials of two liquids is attained. Table 3 contains a set of physical data for the tested fluids. One can notice that the highest oxygen solubility is encountered for HFE liquids. In turn, other fluids such as fluorinated oils, hexadecane and silicone oil are comparable to each other.
Table 3 Physical properties of fluids used in this study. Where there are no references, the data were taken from http://www.guidechem.com
Fluid |
Density (kg m−3) at 25 °C |
Oxygen solubility (mL of gas/1 L of fluid) at 1 bar of air |
Vapour pressure (Torr) at 25 °C |
Diffusion coefficient of oxygen 10−9 (m2 s−1) |
Water |
1000 |
4.775 (36 °C)37 |
23.7 |
2.11 (22 °C)38 |
Hexadecane |
773 |
49.5 (22 °C)38 |
<0.1 |
2.49 (22 °C)38 |
Silicon oil 5 cSt. |
913 |
51.9 (38 °C)39 |
5 |
No data |
FC-40 |
1870 |
76.8 (25 °C)40 |
3 |
8.3 (22 °C)15 |
FC-70 |
1940 |
64.4 (22 °C) |
<0.1 |
3.17 (22 °C) |
HFE-7200 |
1416 |
128.4 (25 °C)41 |
102 42 |
>5 (25 °C)43 |
HFE-7500 |
1559 |
>100 44 |
14 |
>5 (22 °C)43 |
However, in the first experiment, the highest bacteria growth rate was observed for hexadecane and HFE-7500. As a result of the depletion of oxygen in the medium, a difference in the partial pressure of dissolved oxygen between the immiscible liquid and the medium develops that is larger than the initial difference characterising the oxygen-saturated medium. Therefore, oxygen begins to flow from the immiscible oxygenated fluid to the medium. In addition, hexadecane and silicone oil are afloat so they can absorb atmospheric oxygen. The culture tubes are all the time in an orbital shaker, so we cannot base our model on the diffusion process alone due to the large contribution of convective mass transport.
In the case of fluorinated and HFE fluids, they do not have free access to the air due to their density. Similarly, when bacteria begin consuming oxygen, the medium can transport it from high-density fluids – due to the difference in the partial pressure. Now, the medium can also absorb oxygen from the air. We notice that the vicinity of fluorinated oils, which have a lower oxygen solubility than HFE fluids, can cause a lower growth of bacteria. Nevertheless, the measured difference in growth rate of bacteria is not solely dependent on the oxygen solubility in liquids. The key factor is the oxygen exchange rate at the interface,45 but the quantitative description of the oxygen transfer parameters are not well-known yet.10,46
The above description is also consistent with the second experiment with two free interfaces: culture medium/hexadecane and culture medium/HFE-7500. In that case, we notice the highest maximum growth rate of bacteria among all measurements. The depletion of oxygen in the culture medium is quickly replenished through the oxygen transport from hexadecane and HFE-7500, and bacteria do not feel drastic decline of oxygen level.
The last experiment shows the impact of the quantity of immiscible fluid on the growth of bacteria. When we pour more fluid, it is equivalent with supplying more oxygen. In other words, the equilibrium of partial pressure of oxygen distribution between culture medium and the supplying fluid will be reached later. For this reason, bacteria had larger growth rate, when we poured more oxygenated fluid.
The results indicate clearly that the use of oxygenated oils enables a rapid growth of a bacterial culture which leads to the high concentration of cells. The high-density cultures are recommended for a recombinant protein production, especially for those with low expression rate.47 The high concentration of fast-growing bacterial culture reduces the time of interactions of microorganisms with toxic proteins produced. Hence, it contributes to gain a higher protein expression.47 The presence of oil does not alter the behavioural or genomic characteristics of the bacteria due to the oil–water immiscibility. The bacteria grow exclusively in the aqueous phase while the oil phase serves only as a means of high-content oxygen supply and fast interfacial oxygen transfer medium. Therefore, the cultivation of bacteria in bioreactors using oxygenated oils as an oxygen source for bacterial colonies offers a promising alternative to the current technologies used in the industry.
Conclusions
In summary, the much faster growth of bacteria can be obtained with the use of the oxygenated organic oils, fluorinated or HFE fluids. It is important to select the proper immiscible fluid that has a lot of dissolved oxygen. In the microfluidic systems, we can ensure the continuous transport of oxygen from the continuous phase to the bacteria medium located in the microdroplet. By extension, to attention deserve HFE oils and hexadecane. It is further noted that the droplet flowing in a microfluidic channel exhibits intensive mixing as a result of eddy pairs inside the volume.48 Thereby, droplet should have the same concentration of oxygen in the entire volume, as well as at the interface.
Further studies, we are currently conducting with the microfluidic bioreactors, involve synthesis of recombinant proteins using oxygenated oils as the medium supplying oxygen to the growing bacterial culture.
Experimental
Automation and setup
Custom written Lab View (National Instruments, USA) script controlled custom-designed culture tubes and measured the growth of the bacteria in different environments at the same time via National Instruments (USA) card (NI PCIE-6321). Bacteria were grown in 60 mL glass tubes (Rotilabo screw neck ND24 vials (LC94.1), Roth, Germany), similar like in the Toprak's experiment.49 Each tube was placed in a holder containing a LED light source (XPEBAM-L1-0000-00901, http://mouser.com) and a photodiode (TSL257-LF, http://uk.farnell.com). The angle between the optical axis of the LED and photodiode was 135°.49 The holder was set exactly in the middle of the medium fluid with bacteria to monitor the scatter of the light.
The system enables to measure the voltage from photodiodes every 10 minutes. The culture tubes together with holders were placed in the shaking incubator (200 rpm, 37 °C, Grant Bio ES20, Keison International Ltd, UK). Before each measurement, the shaker was turned off for 20 seconds. After the measurement, the shaker was turned back on.
Measurement of light scattering – calibration process
The light scattering was measured using a custom-built sensor applying various McFarland equivalence turbidity standards (R20410, R20411, R20412, R20413, R20414, R20415) purchased from Thermo Fisher Scientific Inc. (USA). As a result, the voltage output of a photodiode could be calibrated and scaled in the McFarland units. To achieve maximum compliance, we have also measured the scattering of the culture medium at different stages of colony growth, both in the bacterial nephelometer (Cn60m/Wgz-2xj, China Medical Devices, China) and with our sensor. As a result, we calculated the Pearson correlation coefficient, which equals 0.983.
Media and reagents
A Luria-Bertani Broth (LB Broth, Biocorp, Poland) was used in all experiments. As a model organism, we used E. coli strain ATCC 25922. The prepared stock solution of cells in LB broth containing 30% glycerol (Sigma-Aldrich, Poland) was stored at −80 °C. Before experiments, cells were streaked on LB agar plates and incubated overnight. We picked individual colonies, used them to inoculate liquid LB broth, cultured the cells at 37 °C with shaking at 200 rpm overnight, and transferred aliquots to the fresh LB media and grew them until the absorbance at 600 nm reached 0.1. The culture was diluted tenfold in fresh medium before transferring suspensions of E. coli into culture tubes. The transfer was performed from the same overnight culture into tubes at the same time. The experiment was repeated ten times for the whole set of fluids.
Oils used in experiments: hexadecane (n-hexadecane, 99%), silicone oil (silicone oil viscosity 5 cSt. (25 °C)), were purchased from Sigma-Aldrich (Poland). In turn, the fluorinert fluids: FC-40 and FC-70, as well as the hydrofluoroether fluids: HFE-7200, HFE-7500, were bought from 3M (USA).
Oxidation process. Before each experiment, the fluids were oxygenated (pure oxygen) for 12 hours at standard air pressure and temperature 25 °C. Oxygen was administered from an air stone (Pawfly Air Stone Bubble for Aquarium, http://amazon.com). The flow rate was 2 L min−1. Unless otherwise noted, the experiments were repeated ten times to verify repeatability and statistics.To avoid any fire hazard while dissolving pure oxygen in oils, the oxygen should be first saturated with water vapours. The use of high-flammability oils, mixed oxygen with small addition (∼1% v/v) of nitrogen is recommended.
Dilution method. The counting of CFUs was repeated three times for each point with the use of LB Agar plates (Biocorp, Poland) after 18 hour incubations at 37 °C.
Conflicts of interest
There are no conflicts to declare.
Acknowledgements
This project was supported by the Polish National Science Centre under the grant Opus 8 no. 2014/15/B/ST4/04955.
Notes and references
- E. Caplice and G. F. Fitzgerald, Int. J. Food Microbiol., 1999, 50, 131–149 CrossRef CAS PubMed.
- A. Y. Tamime, Probiotic Dairy Products, John Wiley & Sons, 2008 Search PubMed.
- S. Leekha, C. L. Terrell and R. S. Edson, Mayo Clin. Proc., 2011, 86, 156–167 CrossRef PubMed.
- S. Kalsi, M. Valiadi, M.-N. Tsaloglou, L. Parry-Jones, A. Jacobs, R. Watson, C. Turner, R. Amos, B. Hadwen, J. Buse, C. Brown, M. Sutton and H. Morgan, Lab Chip, 2015, 15, 3065–3075 RSC.
- Y. J. Lee and K. J. Jeong, J. Biosci. Bioeng., 2015, 120, 483–490 CrossRef CAS PubMed.
- S. Xie, S. Tai, H. Song, X. Luo, H. Zhang and X. Li, J. Mater. Chem. B, 2016, 4, 6820–6829 RSC.
- R. Muñoz, A. J. Daugulis, M. Hernández and G. Quijano, Biotechnol. Adv., 2012, 30, 1707–1720 CrossRef PubMed.
- F. Garcia-Ochoa and E. Gomez, Biotechnol. Adv., 2009, 27, 153–176 CrossRef CAS PubMed.
- S. Suresh, V. Srivastava and I. Mishra, J. Chem. Technol. Biotechnol., 2009, 84, 1091–1103 CrossRef CAS.
- E. Dumont and H. Delmas, Chem. Eng. Process. Process Intensif., 2003, 42, 419–438 CrossRef CAS.
- Y. Chisti, in Kirk-Othmer Encyclopedia of Chemical Technology, John Wiley & Sons, 2000 Search PubMed.
- K. C. Lowe, M. R. Davey and J. B. Power, Trends Biotechnol., 1998, 16, 272–277 CrossRef CAS PubMed.
- A. Baez and J. Shiloach, Microb. Cell Fact., 2014, 13, 181 CrossRef PubMed.
- D. Damiano and S. S. Wang, Biotechnol. Lett., 1985, 7, 81–86 CrossRef CAS.
- L.-K. Ju, J. F. Lee and W. B. Armiger, Biotechnol. Bioeng., 1991, 37, 505–511 CrossRef CAS PubMed.
- H. M. Van Sonsbeek, H. H. Beeftink and J. Tramper, Enzyme Microb. Technol., 1993, 15, 722–729 CrossRef CAS PubMed.
- J. V. Littlejohns and A. J. Daugulis, Chem. Eng. J., 2007, 129, 67–74 CrossRef CAS.
- E. Dumont, Y. Andrès and P. Le Cloirec, Biochem. Eng. J., 2006, 30, 245–252 CrossRef CAS.
- I. Ciani, D. P. Burt, S. Daniele and P. R. Unwin, J. Phys. Chem. B, 2004, 108, 3801–3809 CAS.
- S. Cannan, J. Zhang, F. Grunfeld and P. R. Unwin, Langmuir, 2004, 20, 701–707 CrossRef CAS PubMed.
- M. K. Moraveji, M. M. Pasand, R. Davarnejad and Y. Chisti, Can. J. Chem. Eng., 2012, 90, 93–99 CrossRef CAS.
- K. C. Ruthiya, J. van der Schaaf, B. F. M. Kuster and J. C. Schouten, Ind. Eng. Chem. Res., 2005, 44, 6123–6140 CrossRef CAS.
- F. Malchiodi-Albedi, A. Morgillo, G. Formisano, S. Paradisi, R. Perilli, G. C. Scalzo, G. Scorcia and S. Caiazza, J. Biomed. Mater. Res., 2002, 60, 548–555 CrossRef CAS PubMed.
- C. Holtze, A. C. Rowat, J. J. Agresti, J. B. Hutchison, F. E. Angilè, C. H. J. Schmitz, S. Köster, H. Duan, K. J. Humphry, R. A. Scanga, J. S. Johnson, D. Pisignano and D. A. Weitz, Lab Chip, 2008, 8, 1632 RSC.
- J. L. England, J. Chem. Phys., 2013, 139, 121923 CrossRef PubMed.
- L. Elsgaard and D. Prieur, J. Great Lakes Res., 2011, 37, 203–206 CrossRef CAS.
- M. T. Weinstock, E. D. Hesek, C. M. Wilson and D. G. Gibson, Nat. Methods, 2016, 13, 849–851 CrossRef CAS PubMed.
- N. Blow, Nat. Methods, 2009, 6, 683–686 CrossRef CAS.
- E. K. Sackmann, A. L. Fulton and D. J. Beebe, Nature, 2014, 507, 181–189 CrossRef CAS PubMed.
- A. Gupta, H. B. Eral, T. A. Hatton and P. S. Doyle, Soft Matter, 2016, 12, 2826–2841 RSC.
- S. Jakiela, T. S. Kaminski, O. Cybulski, D. B. Weibel and P. Garstecki, Angew. Chem., Int. Ed., 2013, 52, 8908–8911 CrossRef CAS PubMed.
- L. Mahler, M. Tovar, T. Weber, S. Brandes, M. Michael Rudolph, J. Ehgartner, T. Mayr, M. Thilo Figge, M. Roth and E. Zang, RSC Adv., 2015, 5, 101871–101878 RSC.
- M. Horka, S. Sun, A. Ruszczak, P. Garstecki and T. Mayr, Anal. Chem., 2016, 88, 12006–12012 CrossRef CAS PubMed.
- E. Cabiscol, J. Tamarit and J. Ros, Int. Microbiol. Off. J. Span. Soc. Microbiol., 2000, 3, 3–8 CAS.
- A. Katz, A. Alimova, M. Xu, E. Rudolph, M. K. Shah, H. E. Savage, R. B. Rosen, S. A. McCormick and R. R. Alfano, IEEE J. Sel. Top. Quantum Electron., 2003, 9, 277–287 CrossRef CAS.
- K. Stevenson, A. F. McVey, I. B. N. Clark, P. S. Swain and T. Pilizota, Sci. Rep., 2016, 6, 38828 CrossRef CAS PubMed.
- R. F. Weiss, in Deep Sea Research and Oceanographic Abstracts, Elsevier, 1970, vol. 17, pp. 721–735 Search PubMed.
- L.-K. Ju and C. S. Ho, Biotechnol. Bioeng., 1989, 34, 1221–1224 CrossRef CAS PubMed.
- R. Rodnight, Biochem. J., 1954, 57, 661 CrossRef CAS PubMed.
- B. H. Junker, T. A. Hatton and D. I. Wang, Biotechnol. Bioeng., 1990, 35, 578–585 CrossRef CAS PubMed.
- M. G. Costello, R. M. Flynn and J. G. Owens, in Kirk-Othmer Encyclopedia of Chemical Technology, John Wiley & Sons, 2004 Search PubMed.
- J. Murata, S. Yamashita, M. Akiyama, S. Katayama, T. Hiaki and A. Sekiya, J. Chem. Eng. Data, 2002, 47, 911–915 CrossRef CAS.
- J. D. McMillan and D. I. Wang, Ann. N. Y. Acad. Sci., 1990, 589, 283–300 CrossRef CAS PubMed.
- R. Battino, T. R. Rettich and T. Tominaga, J. Phys. Chem. Ref. Data, 1983, 12, 163–178 CAS.
- A. Chavan and Madhusudan, APCBEE Procedia, 2014, 9, 54–58 CAS.
- G. Quijano, M. Hernandez, S. Villaverde, F. Thalasso and R. Muñoz, Appl. Microbiol. Biotechnol., 2010, 85, 543–551 CrossRef CAS PubMed.
- G. L. Rosano and E. A. Ceccarelli, Front. Microbiol., 2014, 5, 172 Search PubMed.
- P. M. Korczyk, M. E. Dolega, S. Jakiela, P. Jankowski, S. Makulska and P. Garstecki, J. Flow Chem., 2015, 5, 110–118 CrossRef.
- E. Toprak, A. Veres, J.-B. Michel, R. Chait, D. L. Hartl and R. Kishony, Nat. Genet., 2011, 44, 101–105 CrossRef PubMed.
|
This journal is © The Royal Society of Chemistry 2017 |
Click here to see how this site uses Cookies. View our privacy policy here.