DOI:
10.1039/C7RA07460C
(Paper)
RSC Adv., 2017,
7, 37929-37937
Core@shell Fe3O4@Mn2+-doped NaYF4:Yb/Tm nanoparticles for triple-modality T1/T2-weighted MRI and NIR-to-NIR upconversion luminescence imaging agents
Received
6th July 2017
, Accepted 26th July 2017
First published on 1st August 2017
Abstract
Core@shell structures of Fe3O4@Mn2+-doped NaYF4:Yb/Tm nanoparticles (NPs) with multifunctional properties were prepared using a hydrothermal route with a seed-growth procedure. The effect of Mn2+ ions on the phase, shape, and upconversion luminescence (UCL) of Fe3O4@Mn2+-doped NaYF4:Yb/Tm NPs was explored using X-ray diffraction, transmission electron microscopy, dynamic light scattering, and photoluminescence spectroscopy. Furthermore, hydrophobic to hydrophilic surface modification of the Fe3O4@Mn2+-doped NaYF4:Yb/Tm NPs was achieved by coating the NPs with an amphiphilic polymer (poly(maleic anhydride-alt-1-octadecene) (C18PMH)) modified with amine-functionalized methyl ether poly(ethylene glycol). Then, the NIR-to-NIR UCL ascribed to the Tm3+ ions, T1-weighted MRI ascribed to the Mn2+ ions, and T2-weighted MRI ascribed to the Fe3O4 core of the hydrophilic Fe3O4@Mn2+-doped NaYF4:Yb/Tm NPs were evaluated. The results indicate that Mn2+ doping is an effective method to control the size/shape and improve the UCL intensity of Fe3O4@Mn2+-doped NaYF4:Yb/Tm NPs, which are promising as imaging agents for NIR-to-NIR UCL and T1/T2-weighted MRI in biomedical research.
1. Introduction
Multimodal imaging using functional nanoparticles (NPs) is receiving considerable attention because it can combine the advantages of different imaging modes and improve the efficiency of diagnosis and research.1–3 Bifunctional magnetic and luminescent nanomaterials have received considerable attention for multimodal imaging because they provide high sensitivity/resolution fluorescence imaging, and non-invasive and high spatial resolution magnetic resonance imaging (MRI) for real-time monitoring.4–7
Among the various luminescent materials, lanthanide-doped upconversion nanoparticles (UCNPs), which can convert long wavelength near-infrared (NIR) radiation into a visible or NIR emission through an upconversion process, have received a growing amount of interest. Lanthanide-doped UCNPs exhibit higher signal-to-noise ratios, deeper tissue penetration, and lower photodamage than conventional luminescent probes, rendering them ideal for use as luminescent probes in bioimaging.8–14 UCNPs based on NIR-to-NIR emission are more suitable for upconversion optical bioimaging because the biological transparent window is located at 700–1000 nm.3,15–17 Tm-Doped UCNPs have attracted particular interest because they can emit NIR light at approximately 800 nm under 980 nm excitation.3,15–17 However, the application of UCNPs is still restricted because of their low upconversion luminescence (UCL) efficiency. Until now, co-doping with non-lanthanide ions to tailor the local crystal field of the lanthanide ions has been regarded as an effective strategy to improve the UCL efficiency.17–19 Mn2+ ions have been regarded as promising doping ions for UCNPs because they provide a simultaneous enhancement of the upconversion emission and control of the crystal phase/size.20–27
Based on the different roles that they play in imaging, MRI contrast agents can be divided into two categories: T1-weighted contrast agents and T2-weighted contrast agents.28–30 T1-weighted MRI contrast agents make the tissue brighter than the surrounding environment,28,31,32 whereas T2-weighted MRI contrast agents make the contrast parts darker.28,33,34 Traditional MRI using T1- or T2-weighted contrast agents has certain limitations related to the refinement of signals that originate from artifacts (such as fat, calcification, hemorrhages, air) other than diseased lesions.29,35 These agents complicate the image and, in most cases, make it difficult to map the area of disease spread. One of the solutions for this problem is to design a T1/T2 weighted dual mode contrast agent that can provide more detailed pathological information for the diagnosis and monitoring of cancer treatment.28–30 Usually, paramagnetic compounds, such as Mn-based coordination complexes and NPs, can be used as T1-weighted contrast agents.36,37 Gu and co-workers confirmed that Mn2+-doped NaYF4:Yb/Er UCNPs can be used as T1 mode contrast agents.21 Generally speaking, T2-weighted MRI contrast agents are based on superparamagnetic nanomaterials (e.g., Fe3O4), which have large magnetization values.38,39 Consequently, the combination of Fe3O4 with the Mn2+-doped NaYF4:Yb/Tm system can provide a simple strategy to construct the NIR-to-NIR UCL and a binary T1/T2-weighted triple-modality imaging probe.
In the precious work, we applied a seed-growth procedure to synthesize hydrophobic Fe3O4@Mn2+-doped NaYF4:Yb/Er core@shell-structured NPs using oleic acid (OA)-coated Fe3O4 NPs as the seeds and core, these NPs can emit red UCL.8 In that work, we proved that these core@shell-structured NPs can be obtained by hydrothermal method.8 However, the luminescence intensity of these Fe3O4@Mn2+-doped NaYF4:Yb/Er NPs in water was significantly reduced compared to the original hydrophobic NPs when using a ligand exchange method, the quenching effect is mainly caused by the nonradiative decay of the electronically excited states of the dopant lanthanide ions caused by surface ligands and water molecules.8,40,41 It was found that compared with the ligand exchange method, the amphiphilic polymer coating method can maintain better UCL intensity ascribed to the presence of hydrophobic OA ligands in the later case, which could hinder at least partly the direct access of water molecules to the particle surface.40,41 Herein, we applied our reported method to synthesize new hydrophobic Fe3O4@Mn2+-doped NaYF4:Yb/Tm core@shell-structured NPs. The overall synthetic procedure is illustrated in Scheme 1. The crystal phase and morphology of these UCNPs were tuned by doping Mn2+. Then, the hydrophobic Fe3O4@Mn2+-doped NaYF4:Yb/Tm NPs could be transferred into water after coating them with a layer of amphiphilic poly(maleic anhydride-alt-1-octadecene) (C18PMH) modified with amine-functionalized methyl ether poly(ethylene glycol) (C18PMH–mPEG). Finally, the obtained hydrophilic UCNPs were used as contrast agents for NIR-to-NIR luminescent imaging and T1/T2-weighted MRI.
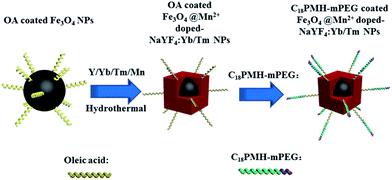 |
| Scheme 1 Schematic representation of the formation of Fe3O4@Mn2+-doped NaYF4:Yb/Tm NPs and their subsequent transfer to water. | |
2. Experimental
2.1. Materials
All chemicals were of analytical grade and used as received without further purification. Deionized water was used throughout. Yttrium nitrate hexahydrate (99.99% trace metals basis), ytterbium nitrate (99.99% trace metals basis), and thulium(III) nitrate hexahydrate (99.9% trace metals basis) were supplied by Best Chemical Reagent Co., Ltd. (Chengdu, China). OA (technical grade, 98.0%), sodium fluoride (reagent grade, 98.0%), sodium hydroxide (reagent grade, 96%), ethanol (reagent grade, 99.7%), ferric trichloride hexahydrate (reagent grade, 99.0%), and manganese dichloride tetrahydrate (reagent grade, 98.0%) were supplied by Kelong Chemical Technology Co., Ltd. (Chengdu, China). Toluene (reagent grade, 99.5%), chloroform (reagent grade, 99.0%), and dichloromethane (reagent grade, 99.0%) were purchased from Ruijinte Chemical Technology Co., Ltd. (Tianjin, China). Ferrous sulfate heptahydrate (reagent grade, 99%) was supplied by Jinshan Chemical Technology Co., Ltd. (Chengdu, China). Ammonia solution (reagent grade, 28%) and triethylamine (TEA) were supplied by Kelong Chemical Technology Co., Ltd. (Chengdu, China). C18PMH was supplied by Sigma-Aldrich Co., LLC. (USA). Methoxypolyethylene glycol amine (mPEG–NH2) was supplied by Aladdin Company (Shanghai, China). 1-Ethyl-3-(3-(dimethylamino)propyl) carbodiimide hydrochloride (EDC·HCl) was obtained from Best Chemical Reagent Co., Ltd. (Chengdu, China).
2.2. Synthesis of C18PMH–mPEG
In a typical procedure,42,43poly(maleic anhydride-alt-1-octadecene) (10 mg, 0.0286 mmol, C18PMH) was reacted with methoxypolyethylene glycol amine (142.85 mg, 0.0286 mmol, mPEG–NH2 5 kDa) in 10 mL of CH2Cl2/TEA (9
:
1, v/v) for 2 h at room temperature. Then, EDC·HCl (10.9 mg, 0.055 mmol) was added, and the solution was stirring for another 24 h. The resulting polymer was dialyzed against ultrapure water through a dialysis membrane (molecular weight cut-off of 8000–14
000) for 2 days. Finally, the C18PMH–mPEG powders were obtained by lyophilization. 1H NMR (400 MHz, CDCl3) δ: 3.7–3.4 (m, br, CH2 of mPEG), 1.3–1.0 (m, CH2 of C18PMH), 1.65 ppm (s, br, CH of C18PMH), 0.88 ppm (m, br, CH3 of C18PMH).
2.3. Synthesis of OA coated Fe3O4@Mn2+-doped NaYF4:Yb/Tm NPs
First, the hydrophobic OA-coated Fe3O4 NPs were prepared according to our previously reported procedure44 and then dispersed in toluene for use in the next step. The as-prepared OA–Fe3O4 NPs were used as seeds and covered with an NIR shell using a facile hydrothermal method, which was similar to the preparation of Mn2+-doped NaYF4:Yb/Er NPs.8 Fe3O4@NaYF4:18% Yb/2% Tm/x Mn (x = 0, 20, 30, 40, and 50 mol%) UCNPs were prepared using a hydrothermal method with OA as a capping ligand. In a typical procedure, Fe3O4@NaYF4:18% Yb/2% Tm/40% Mn NPs (as an example), 3.135 mL of 0.5 M MnCl2, 3.135 mL of 0.5 M Y(NO3)3, 3.53 mL of 0.2 M Yb(NO3)3, and 0.41 mL of a 0.2 M Tm(NO3)3 aqueous solution were added to a mixture of NaOH (1.164 g), 5.88 mL toluene dispersion of OA coated Fe3O4 (5 mg mL−1), OA (19.58 mL), and ethanol (39.16 mL) under stirring. Then, 7.83 mL of deionized water containing 18.5 mmol NaF was added dropwise into the mixture. After vigorous stirring at room temperature for 30 min, the colloidal solution was transferred into a 100 mL Teflon-lined autoclave, which was sealed and heated at 200 °C for 8 h; then, the mixture was cooled to room temperature. The final product was collected using magnetic separation and then washed several times with ethanol and deionized water. Finally, the obtained OA–Fe3O4@NaYF4:18% Yb/2% Tm/40% Mn NPs were dispersed in chloroform.
2.4. Synthesis of C18PMH–mPEG coated Fe3O4@40% Mn2+-doped NaYF4:Yb/Tm NPs
The synthesis of mPEG–Fe3O4@NaYF4:18% Yb/2% Tm/40% Mn NPs was performed according to a previously reported method.43 C18PMH–mPEG (15 mg) and OA coated Fe3O4@40% Mn2+-doped NaYF4:Yb/Tm NPs (15 mg) were dispersed separately in chloroform (10 mL) and then mixed together to obtain a homogeneous phase. The mixture was stirred for 2 h at room temperature. After evaporating the chloroform, the black solid was redispersed in 10 mL of water, and the large aggregates were removed using a 0.22 μm drainage membrane filter and stored at 4 °C.
2.5. Cytotoxicity assay
The cell viability was measured using a 3-(4,5 dimethylthiazol-2-yl)-2,5,-diphenyltetrazolium bromide (MTT) proliferation assay.45,46 Briefly, HeLa cells were seeded into 96-well plates with 200 μL of fresh medium at a density of 1 × 104 cells per well and cultured at 37 °C and 5% CO2 overnight. The cell culture medium in each well was then replaced by 200 μL of cell growth medium containing C18PMH–mPEG coated Fe3O4@40% Mn2+-doped NaYF4:Yb/Tm NPs at concentrations ranging from 0.0625 to 1 mg mL−1. After incubation for 24 h, 200 μL of MTT (0.5 mg mL−1 in a phosphate-buffered saline solution) was added to each well for a further 4 h incubation at 37 °C. The growth medium was removed gently using suction, 300 μL of dimethyl sulfoxide was added to each well as a solubilizing agent, and the microplate was left at room temperature for 2 h.
2.6. In vivo UCL imaging
Firstly, 100 μL of C18PMH–mPEG coated Fe3O4@40% Mn2+-doped NaYF4:Yb/Tm NPs (0.8 mg mL−1) was injected intradermally into the subcutaneous tissue of nude mice. In this system, two external adjustable CW 980 nm lasers (0–5 W) (Shanghai Connet Fiber Optics Co., China) were used as the excitation source, and an Andor DU897 EMCCD was used as the signal collector. Images of luminescent signals were analyzed using the Kodak Molecular Imaging software.
2.7. In vitro T1/T2-weighted MRI
Initially, 2 mL of the C18PMH–mPEG coated Fe3O4@40% Mn2+-doped NaYF4:Yb/Tm NPs solution at Mn concentrations of 0, 0.0346, 0.0691, 0.1382, 0.2763, and 0.5526 mM or Fe concentrations of 0, 0.0973, 0.2434, 0.4868, 0.9737, and 1.9474 mM were prepared before MRI. Both T1-weighted and T2-weighted magnetic resonance (MR) images were acquired using a 0.5 T MesoMR23-060H NMR analyzing and imaging system (Shanghai Niumag Corporation, Shanghai, China). The parameters were set as follows: Q-IR sequence, field of view (FOV) read = 100 mm, FOV phase = 100 mm, TR = 300 ms, TE = 9.5 ms, slices = 1, slice width = 5 mm, averages = 10, k-space size = 192 × 256 for T1-weighted MRI, and Q-CPMG sequence, FOV read = 100 mm, FOV phase = 100 mm, TR = 3000 ms, TE = 80 ms, slices = 1, slice width = 5 mm, averages = 10, k-space size = 192 × 256 for T2-weighted MRI. The relaxivities (r1 and r2) were calculated from a linear fitting of the inverse relaxation time as a function of the Fe or Mn2+ concentration.
2.8. Characterization
The morphology, microstructure, and magnetic properties of the as-prepared NPs were characterized using transmission electron microscopy (TEM; Tecnai F20). The structure was studied using X-ray diffraction (XRD) measurements (Rigaku D/max-2500 V/PC) with Cu Kα radiation (λ = 0.154 nm). The magnetic properties were assessed using a vibrating sample magnetometer (HH-15, China) at 25 °C under an applied magnetic field. The UCL spectra were recorded using a Hitachi F-4600 fluorescent spectrometer with a 980 nm diode laser. Fourier transform infrared (FTIR) spectra were recorded using a Spectrum One instrument (PerkinElmer) and a KBr pellet in the spectral range of 4000 to 500 cm−1. The 1H NMR spectra of methoxy poly(ethylene glycol) phosphoric acid were recorded on an Agilent Technologies 400/54 Annual Refill (400 MR) NMR instrument using CDCl3 as a solvent with a small amount of tetramethylsilane as an internal standard. An inductively coupled plasma mass spectrometry (ICP-MS) system (Shimadzu Corp., Japan) was used to analyze the element concentration of the solution. The T1/T2 relaxation times of the particle suspensions with different Fe concentrations (0.0973–1.9474 mM) or Mn2+ concentrations (0.0346–0.5526 mM) in water were measured using a 0.5 T MesoMR23-060H NMR analyzing and imaging system (Shanghai Niumag Corporation, Shanghai, China).
3. Results and discussion
3.1. Effect of Mn2+ ion doping on the morphology, nanostructure, and UCL of Fe3O4@Mn2+-doped NaYF4:Yb/Tm NPs
Fe3O4@Mn2+-doped NaYF4:Yb/Tm NPs were synthesized by the nucleation and growth of Mn2+-doped NaYF4:Yb/Tm on preformed Fe3O4 NPs. Previous studies showed that the doping of Mn2+ ions could tune the crystal structure, shape/size, and UCL of the UCNPs.20–27 Five samples were synthesized, each doped with 18 mol% Yb3+, 2 mol.
% Tm3+, and 0–50 mol% Mn2+ ions, to investigate the effect of Mn2+ doping on the preparation of Fe3O4@Mn2+-doped NaYF4:Yb/Tm NPs. Then, the crystal structure and phase purity of the five samples were examined using XRD, as shown in Fig. 1. Mn-free UCNPs exhibit both cubic (Joint Committee on Powder Diffraction Standards (JCPDS file number 77-2042) and hexagonal (JCPDS file number 16-0334)) structures (Fig. 1a). Furthermore, the phase transformation from the coexistence of α- and β-phases to the pure α-phase is completed by increasing the Mn2+ content; the pure α-phase can be obtained when the Mn2+ content reaches 20 mol% (Fig. 1b), and no other diffraction peaks are observed even when the Mn2+ content is increased up to 40 mol% (Fig. 1c and d). Nevertheless, extra NaMn3F10 peaks begin to appear when the Mn2+ content reaches 50 mol% (Fig. 1e). In contrast, diffraction peaks relevant to Fe3O4 are not observed in any of the XRD curves, perhaps because the NPs are composed of smaller Fe3O4 NPs coated by a higher percentage of NaYF4:Yb/Tm layer.47 These findings reveal that Mn2+ doping can also promote the hexagonal to cubic phase transformation in the presence of Fe3O4 NPs.
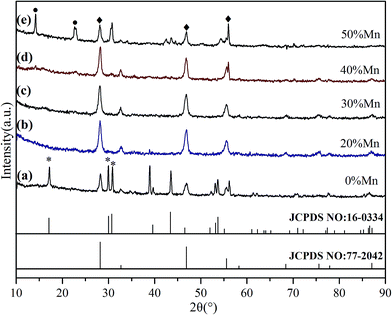 |
| Fig. 1 XRD patterns of OA coated Fe3O4@NaYF4:Yb/Tm NPs co-doped with 0, 20, 30, 40, and 50 mol% Mn2+. The diffraction peaks of the cubic phase are marked with ◆, and peaks related to the hexagonal phase are marked with *. The black dots in curve e indicate a possible extra phase of NaMn3F10. | |
To further reveal the phase and size control, Fe3O4@Mn2+-doped NaYF4:Yb/Tm NPs (doped with different amounts of Mn2+) were characterized by TEM (Fig. 2). The TEM images show that the sample prepared without Mn2+ doping is composed of hexagonal micro rods (β-phase) and small cubic NPs (α-phase) (Fig. 2a and b).48 The addition of Mn2+, as demonstrated in Fig. 2c–f, forms high-quality uniform OA–Fe3O4@Mn2+-doped NaYF4:Yb/Tm NPs with the pure α-phase structure. The inset in Fig. 2e demonstrates the excellent dispersity of the OA coated Fe3O4@40% Mn2+-doped NaYF4:Yb/Tm NPs, which could potentially exist as a magnetic fluid. Fig. 2g shows the typical selected area electron diffraction (SAED) pattern of the Fe3O4@Mn2+-doped NaYF4:Yb/Tm NPs in Fig. 2e, in which the formation of the pure α-phase structure is evident.
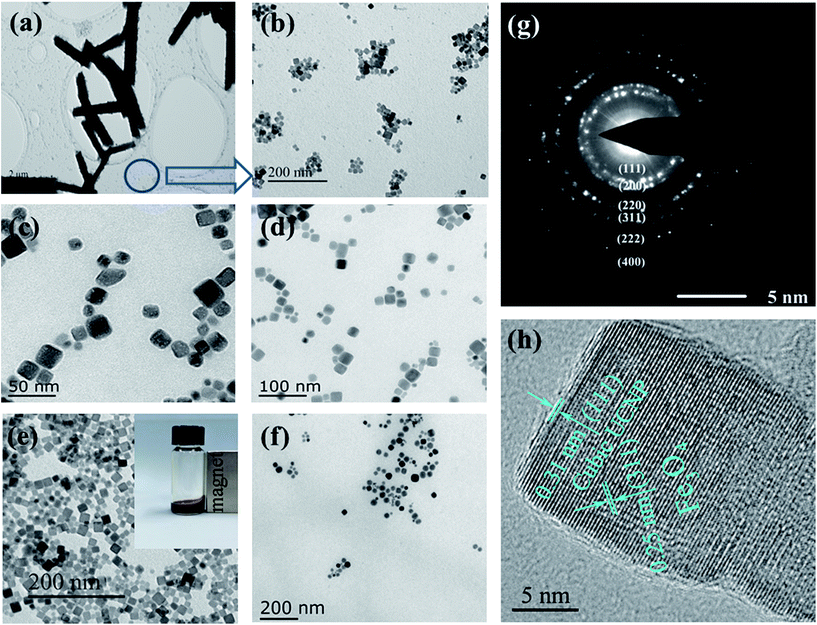 |
| Fig. 2 (a–f) Typical TEM images of OA coated Fe3O4@NaYF4:Yb/Tm NPs doped with different amounts of Mn2+ (0, 20, 30, 40, and 50 mol%, respectively), (g) SAED of NPs in (e), and (h) HRTEM image of a single particle in (e). The inset of (e) shows a photographic image of the chloroform solution of OA coated Fe3O4@40% Mn2+-doped NaYF4:Yb/Tm NPs under a magnet. | |
Fig. 2g also shows a very clear diffraction ring corresponding to the α crystalline NaYF4 (111), (200), (220), (311), (222), and (400) faces, which is consistent with the XRD results. A high-resolution TEM (HRTEM) image (Fig. 2h) of a single particle (shown in Fig. 2e) shows that the measured interplanar spacing of the Fe3O4 cores and Mn2+-doped NaYF4:Yb/Tm shell are approximately 0.25 nm and 0.31 nm, respectively, which agree well with the separation between the (311) lattice planes of the magnetite face-centered cubic structure and the (111) lattice planes of cubic NaYF4. Thus, the HRTEM image shown in Fig. 2h demonstrates successful connection between magnetic seed nanocrystals and the Mn2+-doped NaYF4:Yb/Tm shell.
To reveal the impact of Mn2+ doping on the UCL properties, the UCL spectra of Fe3O4@Mn2+-doped NaYF4:Yb/Tm NPs were studied. Fig. 3 shows the effect of various Mn2+ co-doping contents on the UCL of Fe3O4@Mn2+-doped NaYF4:Yb/Tm NPs. Following the 980 nm laser excitation at room temperature, the UCNPs without Mn2+ doping exhibit an intense NIR emission (∼800 nm) and a weak red emission (∼690 nm). Tm3+ ions are used as activators to generate the emission from the NIR excitation. The Yb3+ emissions can be attributed to transition from the ground state energy level (2F7/2) to the excited state energy level (2F5/2) to Tm3+. Tm3+ is excited from its ground state (3H6) to its excited state (3H5) by absorbing the transition. The NIR emission (∼800 nm) originates from the (3H4) state to the (3H6) ground state. The NIR and red emissions are enhanced as the Mn2+ doping concentrations increase. The overall upconversion emissions reach a maximum value at 40 mol% Mn2+. Nonradiative energy transfer of Tm3+ from the (1D2) and (1G4) states to the (4T1) state of the Mn2+ ions followed by a back energy transfer to the (3F2) state of Tm3+ enhances the fluorescence intensity and possibly improves the transition emission.49 The decrease of the UCL intensity at high Mn2+ contents (50 mol%) could be caused by the exchange interaction between Mn2+ ions, which is depicted in Fig. 3, and significant distortion of the lattice, which induces concentration quenching, thus reducing the UCL intensity.50,51 The result reveals that Mn2+ doping is a general method to tune the crystal structure, size, and shape of UCNPs. According to the above result, the OA coated Fe3O4@40% Mn2+-doped NaYF4:Yb/Tm NPs were chosen as the studying object in the following work.
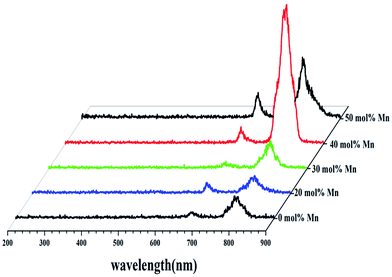 |
| Fig. 3 UCL spectra of OA coated Fe3O4@NaYF4:Yb/Tm NPs co-doped with Mn2+ = 0, 20, 30, 40, and 50 mol% under the excitation of a 980 nm diode laser. | |
3.2. Hydrophobic to hydrophilic transfer of Fe3O4@Mn2+-doped NaYF4:Yb/Tm NPs
Here, an amphiphilic polymer (C18PMH–mPEG) was synthesized by the reaction of C18PMH with amine-functionalized methyl ether poly(ethylene glycol). The as-prepared hydrophobic Fe3O4@40% Mn2+-doped NaYF4:Yb/Tm NPs were then transferred to a hydrophilic phase after coating them with C18PMH–mPEG. The octadecene chains present in the alternating C18PMH–mPEG intercalate with the oleate ligands present on the surface of the Fe3O4@40% Mn2+-doped NaYF4:Yb/Tm NPs because of hydrophobic interactions, while the anhydride rings and the mPEG segments in the polymer are exposed to the solvent. The efficacy of the C18PMH–mPEG coating is probed using several complementary techniques. Firstly, the ligand change was investigated using FTIR. For OA coated Fe3O4@40% Mn2+-doped NaYF4:Yb/Tm NPs, the peaks at 2938 and 2868 cm−1 are assigned to the asymmetric and symmetric stretching vibrations of methylene (–CH2–) in the long alkyl chain of OA, and the band at 1468 cm−1 is attributed to symmetric stretch vibrations of (RCOO–), indicating that OA is coated on the surface of the OA coated Fe3O4@40% Mn2+-doped NaYF4:Yb/Tm NPs (Fig. 4a). For the curve of C18PMH–mPEG coated Fe3O4@40% Mn2+-doped NaYF4:Yb/Tm NPs, the new peak at 1116 cm−1 is attributed to –C–O– in mPEG, and the new peak at 1662 cm−1 corresponds to the absorption of the carbonyl (C
O), which suggests the formation of a C18PMH–mPEG layer on the Fe3O4@40% Mn2+-doped NaYF4:Yb/Tm NPs.
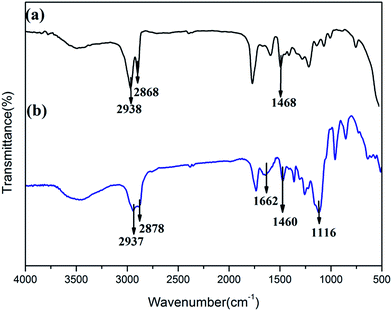 |
| Fig. 4 FTIR spectra of OA (a) and C18PMH–mPEG (b) coated Fe3O4@40% Mn2+-doped NaYF4:Yb/Tm NPs. | |
Fig. 5 shows TEM images of the Fe3O4@40% Mn2+-doped NaYF4:Yb/Tm NPs before and after polymer coating. Compared with the OA coated Fe3O4@40% Mn2+-doped NaYF4:Yb/Tm NPs (Fig. 5a), after modification with C18PMH–mPEG the Fe3O4@40% Mn2+-doped NaYF4:Yb/Tm NPs NPs maintain the cubic phase structure (show in Fig. 5b). In addition, the C18PMH–mPEG coated Fe3O4@40% Mn2+-doped NaYF4:Yb/Tm NPs were separated from each other without observable aggregation, suggesting that they were effectively stabilized in aqueous solution (Fig. 5b).
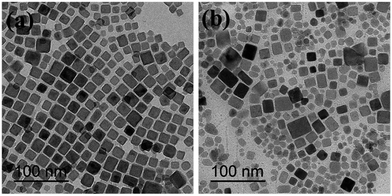 |
| Fig. 5 TEM images of OA (a) and C18PMH–mPEG (b) coated Fe3O4@40% Mn2+-doped NaYF4:Yb/Tm NPs. | |
Fig. 6 shows the magnetic hysteresis measurements of the prepared Fe3O4@40% Mn2+-doped NaYF4:Yb/Tm NPs before and after polymer coating, measured at room temperature. The field-dependent magnetization plots illustrate that OA coated Fe3O4@40% Mn2+-doped NaYF4:Yb/Tm NPs (see Fig. 6a) are superparamagnetic with saturation magnetization values of approximately 3.1 emu g−1 at 25 °C. The C18PMH–mPEG coated Fe3O4@40% Mn2+-doped NaYF4:Yb/Tm NPs (see Fig. 6b) exhibit a saturation magnetization value of 2.4 emu g−1, which is significantly lower than that of OA coated Fe3O4@40% Mn2+-doped NaYF4:Yb/Tm NPs. This may be due to the C18PMH–mPEG polymer coating on the Fe3O4@40% Mn2+-doped NaYF4:Yb/Tm NPs.
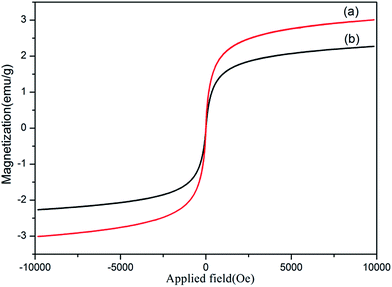 |
| Fig. 6 Room-temperature magnetic hysteresis loop for OA (a) and C18PMH–mPEG (b) coated Fe3O4@40% Mn2+-doped NaYF4:Yb/Tm NPs. | |
Fig. 7 shows the UCL properties of the Fe3O4@40% Mn2+-doped NaYF4:Yb/Tm NPs NPs before and after polymer coating. The UCL intensity of the C18PMH–mPEG coated Fe3O4@40% Mn2+-doped NaYF4:Yb/Tm NPs is slightly reduced compared to the original hydrophobic NPs. This can be attributed to the nonradiative decay of the electronically excited states of the dopant lanthanide ions caused by surface ligands and water molecules.40,41 Even so, the C18PMH–mPEG coated Fe3O4@40% Mn2+-doped NaYF4:Yb/Tm NPs still exhibit good fluorescence properties. The inset in the top left corner shows that complete transfer of the Fe3O4@40% Mn2+-doped NaYF4:Yb/Tm NPs from the bottom chloroform layer to the top water layer is achieved, indicating that Fe3O4@40% Mn2+-doped NaYF4:Yb/Tm NPs are successfully coated by C18PMH–mPEG.
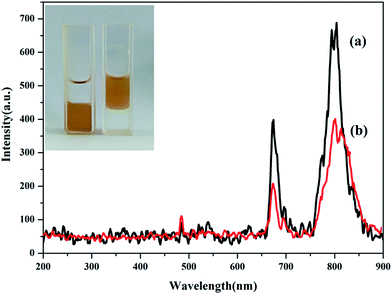 |
| Fig. 7 Upconversion emission spectra of (a) the chloroform solution of OA coated Fe3O4@40% Mn2+-doped NaYF4:Yb/Tm NPs and (b) the water solution of C18PMH–mPEG coated Fe3O4@40% Mn2+-doped NaYF4:Yb/Tm NPs under 980 nm excitation. The insets show photographic images of Fe3O4@40% Mn2+-doped NaYF4:Yb/Tm NPs dispersed in chloroform and water. | |
3.3. Cytotoxicity test
The cytotoxicity of the C18PMH–mPEG coated Fe3O4@40% Mn2+-doped NaYF4:Yb/Tm NPs was determined using HeLa cells and a standard Cell Counting Kit-8 (CCK-8) colorimetric assay. After 24 h of incubation with concentrations varying from 0.0625 to 1 mg mL−1, the C18PMH–mPEG coated Fe3O4@40% Mn2+-doped NaYF4:Yb/Tm NPs exhibit a satisfactory biocompatibility (see Fig. 8). The cell viability is greater than 78%, even at a high-dose concentration of 1 mg mL−1. These results indicate that these C18PMH–mPEG coated Fe3O4@40% Mn2+-doped NaYF4:Yb/Tm NPs are potentially suitable for biomedical applications.
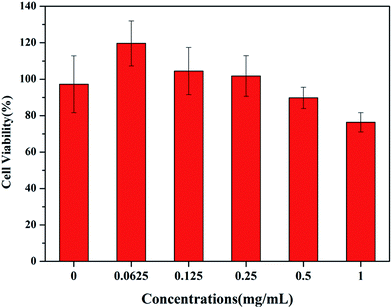 |
| Fig. 8 In vitro cytotoxicity of C18PMH–mPEG coated Fe3O4@40% Mn2+-doped NaYF4:Yb/Tm NPs against HeLa cells at different concentrations after a 24 h incubation. | |
3.4. In vivo UCL imaging
To evaluate the NIR-to-NIR UCL imaging ability of these C18PMH–mPEG coated Fe3O4@40% Mn2+-doped NaYF4:Yb/Tm NPs, a 5 week-old nude mouse (male) was anaesthetized and injected with 100 μL of 0.8 mg mL−1 C18PMH–mPEG coated Fe3O4@40% Mn2+-doped NaYF4:Yb/Tm NPs in the abdomen region for in vivo imaging. The injection depth was about 10 mm (estimated from the needle penetration). As shown in Fig. 9, the UCL imaging could be obtained from the injected sites using an EMCCD camera by collecting NIR emission signal at 800 ± 12 nm. In particular, region of interest (ROI) analysis of the UCL signal (λem = 800 ± 12 nm) reveals a high signal-to-noise ratio (∼26) between the abdomen region and the background. The high contrast UCL image with ultra-low auto-fluorescence interferences can be attribute to the antistokes luminescence feature of UCL as well as the wavelength of the emission light that falls within the NIR window for bioimaging (700–1000 nm).4
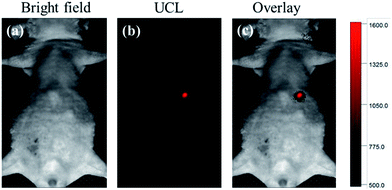 |
| Fig. 9 In vivo UCL imaging. (a) Bright field image; (b) UCL image after injection with 100 μL of C18PMH–mPEG coated Fe3O4@40% Mn2+-doped NaYF4:Yb/Tm NPs (0.8 mg mL−1) in the abdomen (power density of 80 mW cm−2, λexcitation = 980 nm, λemission = 800 ± 12 nm); (c) merged image of bright field image and the UCL signals. | |
3.5. T1/T2-weighted MRI
The elemental composition of the C18PMH–mPEG coated Fe3O4@40% Mn2+-doped NaYF4:Yb/Tm NPs was further analyzed by ICP-MS, where the Fe/Mn molar ratio was estimated to be 3.54
:
1. To explore the T1-weighted imaging performance, MR phantom studies of the C18PMH–mPEG coated Fe3O4@40% Mn2+-doped NaYF4:Yb/Tm NPs dispersed in water at different Mn2+ concentrations were investigated (Fig. 10). As shown in Fig. 10a, the brighter signals in the T1-weighted images increase as a function of the Mn2+ concentration (0–0.5526 mM). The T1 relaxivity coefficient (r1) for the C18PMH–mPEG coated Fe3O4@40% Mn2+-doped NaYF4:Yb/Tm NPs could also be calculated from the curve of 1/T1 versus the Mn2+ concentration (Fig. 10b). The data show that r1 is 4.7 mM−1 s−1 which is close to the relaxivity of commercial Gd-diethylenetriaminepentaacetic acid (4.82 mM−1 s−1).
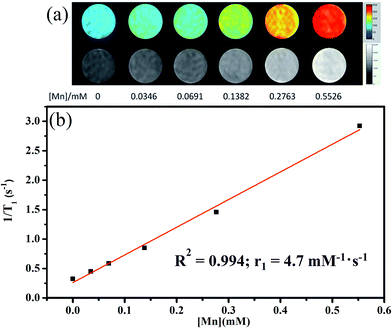 |
| Fig. 10 Color T1-weighted images (a) and linear fitting of 1/T1 (b) of the C18PMH–mPEG coated Fe3O4@40% Mn2+-doped NaYF4:Yb/Tm NPs with different Mn concentrations. The color bar from blue to red indicates the gradual increase of the MR signal intensity. | |
To demonstrate the potential to use the NPs for T2-weighted MRI, MR phantom studies of the NPs as a function of Fe concentration were also performed (Fig. 11). T2-weighted MR images (Fig. 11a) reveal that the brightness decreases with increasing Fe concentrations (0–1.9474 mM). Meanwhile, the T2 relaxation rate (1/T2) increases linearly with respect to the Fe concentration, and the slope (r2 relaxivity) is calculated to be 16.72 mM−1 s−1 (Fig. 11b). Although, the r2 relaxivity of the C18PMH–mPEG coated Fe3O4@40% Mn2+-doped NaYF4:Yb/Tm NPs is lower than that of many MRI contrasts, the content of Fe3O4 here could be tuned to increase the r2 relaxivity.
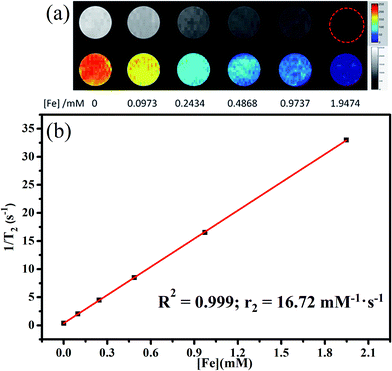 |
| Fig. 11 Color T2-weighted images (a) and linear fitting of 1/T2 (b) of the C18PMH–mPEG coated Fe3O4@40% Mn2+-doped NaYF4:Yb/Tm NPs with different Fe concentrations. The color bar from blue to red indicates the gradual increase of the MR signal intensity. | |
4. Conclusions
In summary, we synthesized core@shell OA-coated Fe3O4@Mn2+-doped NaYF4:Yb/Tm NPs using a hydrothermal method. Besides the enhancement of the NIR UCL (UCL band at ∼800 nm), the control of the phase and size of these NPs was achieved by Mn2+ doping. Furthermore, the water-dispersible Fe3O4@40% Mn2+-doped NaYF4:Yb/Tm NPs were obtained by coating with an amphiphilic polymer (C18PMH–mPEG), which exhibited good biocompatibility. Moreover, the prepared C18PMH–mPEG coated Fe3O4@40% Mn2+-doped NaYF4:Yb/Tm NPs could be used for in vivo NIR to NIR imaging. The developed NPs could also be used as dual-mode T1/T2-weighted MRI because of the co-existence of Fe3O4 and Mn2+ in the NPs. We expect that such multifunctional NPs that combine the advantages of magnetic drug delivery, NIR-to-NIR UCL, and MRI contrast, will find applications in anticancer therapy.
Acknowledgements
This work was supported by the National Natural Science Foundation of China (51273220), the Fundamental Research Funds for the Central Universities (2017NZYQN17). The authors thank Prof. Fuyou Li, Prof. Wei Feng and Cong Cao from FuDan University, who gave many instructions on UCL imaging.
References
- B. R. Smith and S. S. Gambhir, Chem. Rev., 2017, 117, 901 CrossRef CAS PubMed.
- O. S. Wolfbeis, Chem. Soc. Rev., 2015, 44, 4743 RSC.
- A. Xia, Y. Gao, J. Zhou, C. Y. Li, T. S. Yang, D. M. Wu, L. M. Wu and F. Y. Li, Biomaterials, 2011, 32, 7200 CrossRef CAS PubMed.
- B. Shen, Y. L. Gao, Q. Y. Liu, S. M. Cheng, W. Feng and F. Y. Li, RSC Adv., 2017, 7, 21625 RSC.
- X. Wang, M. Tu, K. Yan, P. Li, L. Pang, Y. Gong, Q. Li, R. Liu, Z. Xu, H. Xu and P. K. Chu, ACS Appl. Mater. Interfaces, 2015, 7, 24523 CAS.
- L. Cheng, K. Yang, Y. Li, J. Chen, C. Wang, M. Shao, S. T. Lee and Z. Liu, Angew. Chem., Int. Ed., 2011, 7385 CrossRef CAS PubMed.
- L. Zhang, Y. S. Wang, Y. Yang, F. Zhang, W. F. Dong, S. Y. Zhou, W. H. Pei, H. D. Chen and H. B. Sun, Chem. Commun., 2012, 48, 11238 RSC.
- Z. L. Qin, S. N. Du, Y. Luo, Z. J. Liao, F. Zuo, J. B. Luo and D. Liu, Appl. Surf. Sci., 2016, 378, 174 CrossRef CAS.
- X. J. Zhu, W. Feng, J. Chang, Y. W. Tan, J. C. Li, M. Chen, Y. Sun and F. Y. Li, Nat. Commun., 2016, 7, 10437 CrossRef CAS PubMed.
- L. Zhou, R. Wang, C. Yao, X. Li, C. Wang, X. Zhang, C. Xu, A. Zeng, D. Zhao and F. Zhang, Nat. Commun., 2015, 6, 6938 CrossRef CAS PubMed.
- N. M. Idris, M. K. Gnanasammandhan, J. Zhang, P. C. Ho, R. Mahendran and Y. Zhang, Nat. Med., 2012, 18, 1580 CrossRef CAS PubMed.
- J. Tang, L. Chen, J. Li, Z. Wang, J. Zhang, L. Zhang, Y. Luo and X. Wang, Nanoscale, 2015, 7, 14752 RSC.
- Q. Liu, W. Feng, T. Yang, T. Yi and F. Li, Nat. Protoc., 2013, 8, 2033 CrossRef CAS PubMed.
- B. Shen, Y. L. Gao, Q. Y. Liu, S. M. Cheng, W. Feng and F. Y. Li, RSC Adv., 2017, 7, 21625 RSC.
- N. N. Dong, M. Pedroni, F. Piccinelli, G. Conti, A. Sbarbati, J. E. Ramírez Hernández, L. M. Maestro, M. C. I. Cruz, F. SanzRodriguez, A. Juarranz, F. Chen, F. Vetrone, J. A. Capobianco, J. G. S. MarcoBettinelli, D. Jaque and A. Speghini, ACS Nano, 2011, 5, 8665 CrossRef CAS PubMed.
- M. Nyk, R. Kumar, T. Y. Ohulchanskyy, E. J. Bergey and P. N. Prasad, Nano Lett., 2008, 8, 3834 CrossRef CAS PubMed.
- M. Hu, D. D. Ma, Y. Z. Cheng, C. C. Liu, Z. P. Zhang, Y. J. Cai, S. Wu and R. F. Wang, J. Mater. Chem. B, 2017, 5, 2662 RSC.
- Y. T. Zhang, Y. L. Shen, M. Liu, Y. Han, X. L. Mo, R. B. Jiang, Z. B. Lei, Z. H. Liu, F. Shi and W. P. Qin, CrystEngComm, 2017, 19, 1304 RSC.
- D. Yin, C. Wang, J. Ouyang, K. Song, B. Liu, X. Cao, L. Zhang, Y. Han, X. Long and M. Wu, Dalton Trans., 2014, 43, 12037 RSC.
- J. Wang, F. Wang, C. Wang, Z. Liu and X. G. Liu, Angew. Chem., Int. Ed., 2011, 50, 10369 CrossRef CAS PubMed.
- G. Tian, Z. J. Gu, L. J. Zhou, W. Y. Yin, X. X. Liu, L. Yan, S. Jin, W. L. Ren, G. M. Xing, S. J. Li and Y. L. Zhao, Adv. Mater., 2012, 24, 1226 CrossRef CAS PubMed.
- S. J. Zeng, Z. G. Yi, W. Lu, C. Qian, H. B. Wang, L. Rao, T. M. Zeng, H. R. Liu, H. J. Liu, B. Fei and J. H. Hao, Adv. Funct. Mater., 2014, 24, 4051 CrossRef CAS.
- C. Wang, L. Cheng, Y. M. Liu, X. J. Wang, X. X. Ma, Z. Y. Deng, Y. G. Li and Z. Liu, Adv. Funct. Mater., 2013, 23, 3077 CrossRef CAS.
- X. Y. Li, X. W. Liu, D. M. Chevrier, X. Qin, X. J. Xie, S. Y. Song, H. J. Zhang, P. Zhang and X. G. Liu, Angew. Chem., 2015, 127, 13510 CrossRef.
- G. Tian, W. Ren, L. Yan, S. Jian, Z. J. Gu, L. Zhou, S. Jin, W. Yin, S. Li and Y. Zhao, Small, 2013, 9, 1929 CrossRef CAS PubMed.
- Y. B. Li, X. L. Li, Z. L. Xue, M. M. Jiang, S. J. Zeng and J. H. Hao, Adv. Healthcare Mater., 2017, 6, 1601231 CrossRef PubMed.
- Z. Y. Huang, H. P. Gao and Y. L. Mao, RSC Adv., 2016, 6, 83321 RSC.
- J. Zhou, Z. G. Lu, G. G. Shan, S. H. Wang and Y. Liao, Biomaterials, 2014, 35, 368 CrossRef CAS PubMed.
- E. Shah, P. Upadhyay, M. Singh, M. S. Mansuri, R. Begum, N. Sheth and H. P. Soni, New J. Chem., 2016, 40, 9507 RSC.
- N. D. Thorat, R. A. Bohara, H. M. Yadav and S. A. M. Tofail, RSC Adv., 2016, 6, 94967 RSC.
- Y. Chen, H. Chen, S. Zhang, F. Chen, S. Sun and Q. He, Biomaterials, 2012, 33, 2388 CrossRef CAS PubMed.
- H. Yang, Y. Zhuang, H. Hu, X. Du, C. Zhang and X. Shi, Adv. Funct. Mater., 2010, 20, 1733 CrossRef CAS.
- L. Cheng, K. Yang, Y. G. Li, J. H. Chen, C. Wang and M. W. Shao, Angew. Chem., Int. Ed., 2011, 50, 7385 CrossRef CAS PubMed.
- H. Yang, C. Zhang, X. Shi, H. Hu, X. Du and Y. Fang, Biomaterials, 2010, 31, 3667 CrossRef CAS PubMed.
- J. W. M. Bulte and D. L. Kraitchman, NMR Biomed., 2004, 17, 484 CrossRef CAS PubMed.
- L. Turyanska, F. Moro, A. Patanè, J. Barr, W. Köckenberger, A. Taylor, H. M. Faas, M. Fowler, P. Wigmore, R. C. Trueman, H. E. L. Williams and N. R. Thomas, J. Mater. Chem. B, 2016, 4, 6797 RSC.
- J. C. Li, Y. Hu, W. J. Sun, Y. Luo, X. Y. Shi and M. W. Shen, RSC Adv., 2016, 6, 35295 RSC.
- Z. J. Zhou, L. R. Wang, X. Q. Chi, J. F. Bao, L. J. Yang, W. X. Zhao, Z. Chen, X. M. Wang, X. Y. Chen and J. H. Gao, ACS Nano, 2013, 7, 3287 CrossRef CAS PubMed.
- M. Borges, S. Yu, A. Laromaine, A. Roig, S. Súarez-Garcia, J. Lorenzo, D. Ruiz-Molinaa and F. Novio, RSC Adv., 2015, 5, 86779 RSC.
- O. Plohl, S. Kralj, B. Majaron, E. Fröhlich, M. Ponikvar-Svet, D. Makovec and D. Lisjak, Dalton Trans., 2017, 46, 6975 RSC.
- R. Arppe, I. Hyppanen, N. Perala, R. Peltomaa, M. Kaiser, C. Wurth, S. Christ, U. Resch-Genger, M. Schaferling and T. Soukka, Nanoscale, 2015, 7, 11746 RSC.
- G. Prencipe, S. M. Tabakman, K. Welsher, Z. Liu, A. P. Goodwin, L. Zhang, J. Henry and H. J. Dai, J. Am. Chem. Soc., 2009, 9, 4787 Search PubMed.
- R. Y. Wei, Z. W. Wei, L. N. Sun, J. Z. Zhang, J. L. Liu, X. Q. Ge and L. Y. Shi, ACS Appl. Mater. Interfaces, 2016, 8, 400 CAS.
- P. An, F. Zuo, Y. P. Wu, J. H. Zhang, Z. H. Zheng, X. B. Ding and Y. X. Peng, Chin. Chem. Lett., 2012, 23, 1099 CrossRef CAS.
- Q. Ju, D. T. Tu, Y. S. Liu, R. F. Li, H. M. Zhu, J. C. Chen, Z. Chen, M. D. Huang and X. Y. Chen, J. Am. Chem. Soc., 2012, 134, 1323 CrossRef CAS PubMed.
- D. D. Yin, C. C. Wang, J. Ouyang, K. L. Song, B. Liu, X. Z. Cao, L. Zhang, Y. L. Han, X. Long and M. H. Wu, Dalton Trans., 2014, 43, 12037 RSC.
- C. C. Mi, J. P. Zhang, H. Y. Gao, X. L. Wu, M. Wang, Y. F. Wu, Y. Q. Di, Z. R. Xu, C. B. Mao and S. K. Xu, Nanoscale, 2010, 2, 1141 RSC.
- X. Wang, Y. Bu and Y. Xiao, J. Mater. Chem. C, 2013, 1, 3158 RSC.
- S. Z. Wang, J. G. Zhang, H. Q. Chen and L. Wang, J. Lumin., 2015, 168, 82 CrossRef CAS.
- P. Ramasamy, P. Chandra, S. W. Rhee and J. Kim, Nanoscale, 2013, 5, 8711 RSC.
- J. Tang, L. Chen, J. Li, Z. Wang, J. H. Zhang, L. G. Zhang, Y. S. Luo and X. J. Wang, Nanoscale, 2015, 7, 14752 RSC.
|
This journal is © The Royal Society of Chemistry 2017 |
Click here to see how this site uses Cookies. View our privacy policy here.