DOI:
10.1039/C7RA08427G
(Paper)
RSC Adv., 2017,
7, 50035-50039
Highly sensitive and selective fluorescent probe for Fe3+ and hazardous phenol compounds based on a water-stable Zn-based metal–organic framework in aqueous media†
Received
31st July 2017
, Accepted 11th October 2017
First published on 26th October 2017
Abstract
A novel two-fold interpenetrating network, namely, {[Zn(DPTMIA)]·(H2O)2(DMF)0.5}n (HPU-1), with rectangular channels was synthesized and structurally characterized. HPU-1 was shown to be potentially applicable as a multi-functional fluorescent probe. It was shown to specifically detect Fe3+, through a cation exchange mechanism, and hazardous phenol compounds, using a mechanism involving a decrease in the efficiency of the transfer of electrons and energy from the ligands to the metal ions. The detection limits of HPU-1 for Fe3+ and the phenol compounds were found to be lower than those of MOFs reported in the literature. This HPU-1 probe was shown to be functional in aqueous solution and to be affected neither by the type of solvent nor the pH.
Introduction
Fe3+ is ubiquitous in cells, and thus plays a crucial role in the human body and other biological tissues. A deficiency and excess of Fe3+ from the normal permissible limit may induce serious disorders.1 Therefore, the detection of iron has gained increasing interest in recent years.2 Recently, the design of fluorophore-based Fe3+-sensing materials has been actively pursued because of the safety, operational simplicity, humanitarian implications and non-destructive character of these materials.3 However, these materials do show some problems, such as low sensitivity and poor stability. Therefore, synthesizing novel fluorescent probes for the detection of metal ions or small organic molecules is a challenging yet important task.
Fluorescent probes based on the metal–organic framework (MOF) have been widely investigated for the selective sensing of metal ions or toxic organic complexes on account of their visible luminescence and designable architectures, which have allowed for improved host–guest interactions and pre-concentrators for target analytes.4 Major research has focused on the lanthanide MOFs due to their high luminous efficiency, long-wavelength emission and lifetime.5 However, there are some defects existing in the synthesis process of Ln-MOFs, such as: expensive raw materials, secondary pollution and so on. Therefore, MOFs based on transition metal ions, particularly Zn2+, have captured our interest because of their advantages, including their environmental compatibility, low cost, accessibility and unimodal emission.6 In addition, transition metal-based MOFs could provide facile Lewis acid active sites and potential space within the MOF channel for sensing an ion or molecule.7 However, to the best of our knowledge, only a few Fe3+ probes based on transition MOFs have been developed.8
Phenol compounds are important raw materials that are frequently used in synthesizing plastic, adhesives, dyes, medicine, and pesticides, but are also hazardous. Their long-term residual accumulation in the ecosystem has caused serious environmental problems because of their intrinsic high toxicity and non-degradability.9 Thus, the detection of phenol compounds through simple and inexpensive methods is an international concern. However, less effort has been focused on the detection of these compounds than has been expended on the detection of other hazardous substances. MOFs, due to their intrinsic traits including their tunable luminescence and exposed active sites, generally sense nitro-aromatic compounds more efficiently than do conventional sensors.10 The combination of advantages of MOFs enable them to be promising candidates as probes for phenol compounds. Furthermore, such a probe that can operate in an aqueous medium is highly desirable for in-field selective detection of phenol compounds present in soil and ground water. To develop fluorescent MOFs that work in aqueous media for the selective detection of Fe3+ and phenol compounds, a water-stable Zn-based MOF, {[Zn(DPTMIA)]·(H2O)2(DMF)0.5}n (HPU-1) with strong luminescence intensity and rectangle-type tubular channels was synthesized. HPU-1 was shown to be able to rapidly detect Fe3+ from a mixture of metal ions through an ion exchange mechanism and to exhibit high sensitivity for phenol compounds in aqueous media through an energy transfer mechanism.
Experimental
Materials and physical measurements
All chemicals used in our work were commercially available and used as purchased without further purification. The other reagents and solvents referred to were also commercially available and were employed without further purification. Elemental analyses (C, H, and N) were carried out on a FLASH EA 1112 elemental analyzer. Powder X-ray diffraction (PXRD) experiments were recorded using Cu Kα1 radiation on a PANalytical X'Pert PRO diffractometer. Thermal analyses were performed on a Netzsch STA 449C thermal analyzer from room temperature at a heating rate of 10 °C min−1 in air. The luminescence spectra for the powdered solid samples were acquired at room temperature on a Hitachi F-4500 fluorescence spectrophotometer. The widths of the excitation and emission slits were each 5 nm. The UV spectra were recorded on a Purkinje General TU-1800 spectrophotometer.
Synthesis of {[Zn(DPTMIA)]·(H2O)2(DMF)0.5}n (HPU-1)
The colorless block crystal of HPU-1 was easily produced via a hydrothermal reaction of Zn(NO3)2 (14.85 mg, 0.03 mmol) and DPTMIA (5-(3,5-di-pyridin-4-yl-[1,2,4]triazol-1-ylmethyl)-isophthalic acid) (12.03 mg, 0.03 mmol) in a solution of H2O, CH3CN and DMF (2 + 2 + 1 mL) carried out at 80 °C for 72 h. Yield: 47%. Elemental analysis data calcd for C22.50H20.50N5.50O6.50Zn: C 50.29%, H 3.84%, N 14.33%. Found: C 50.44%, H 3.28%, N 14.66%.
Crystal data collection and refinement
The crystallographic diffraction data for HPU-1 were obtained on a Siemens Smart CCD single-crystal X-ray diffractometer with a graphite monochromatic MoKalpha radiation (λ = 0.71073 Å) at 296 K. The structure was solved by using direct methods with the SHELXS-2014 program of the SHELXTL package and refined on F2 by using full-matrix least-squares techniques with SHELXL-2014. All empirical absorption corrections were applied using the SADABS program. All non-hydrogen atoms were refined anisotropically, while hydrogen atoms were located and refined geometrically. A SQUEEZE/PLATON technique was applied to remove disordered solvent that could not be satisfactorily modeled. The crystallographic data and structural refinement parameters of the complex are summarized in Table S1,† and the selected bond lengths and angles are collated in Table S2.†
Results and discussion
Crystal structure of {[Zn(DPTMIA)]·(H2O)2(DMF)0.5}n
The structure of HPU-1 is shown in Fig. 1 and was determined using single-crystal X-ray diffraction (XRD) studies. The HPU-1 framework formed from Zn2+ ions bridged by DPTMIA ligands (Fig. 1). Each ligand was observed to coordinate with four Zn2+ atoms using its two carboxylate groups and two pyridine N atoms. Every Zn2+ ion showed the same tetrahedral coordination environment, with the ion being surrounded by two O atoms and two N atoms. As shown in Fig. S1,† the extension of the structure into a 3D framework was accomplished by the connections of the ligands and metal ions. The vacant space in HPU-1 was calculated using Platon to be approximately 31.1%. Other potential voids were found to be filled through mutual interpenetration of another independent equivalent framework in a normal mode, producing a two-fold interpenetrating network (Fig. 1). Further analysis indicated the presence of long rectangular channels with cross-sectional dimensions of 11.4362 × 8.8427 Å2 (Fig. 1).
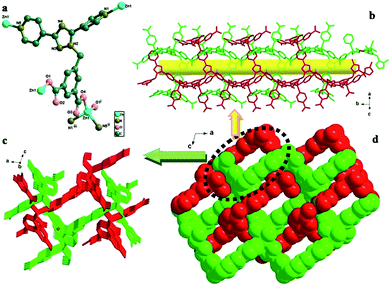 |
| Fig. 1 (a) Linking of the DPTMIA ligand to four Zn2+ atoms in the title complex. (b) The rectangular channel. (c) The 2-fold interpenetrating framework. (d) 3D coordination framework with rectangle-type tubular channels. | |
Sensing of metal ions
As shown in Fig. S2,† thermal analyses indicated HPU-1 to have high thermal stability. The water stability of HPU-1 was also investigated, as shown in Fig. S3.† Powder XRD patterns of the as-synthesized and water-treated samples (HPU-1 samples were immersed in water for one month) both agreed with the simulated pattern from single-crystal analysis, indicating the water stability of the framework of HPU-1, and hence showing its potential to function in an aqueous environment. To investigate the potential ion recognition of HPU-1, the samples of HPU-1 were ground in various 0.01 mol L−1 aqueous solutions containing different metal ions (Ag+, Al3+, Ba2+, Ca2+, Co2+, Cr3+, Cu2+, Fe3+, Mn2+, Ni2+, Pb2+, Cd2+, K+, Li+, Mg2+, Na+, Eu3+ and Tb3+). The suspension-state photoluminescence properties of the various Mn+@HPU-1 samples were determined, and are compared in Fig. 2. The emission intensity of HPU-1 was nearly unchanged upon being combined with nearly any of the metal ions other than Fe3+. In contrast, Fe3+ completely quenched the emission of HPU-1 (Fig. S4†). The ability of HPU-1 to sensitively detect Fe3+ in an aqueous solution inspired us to further examine the effects of other metal ions on its Fe3+-sensing functions. For this purpose, the same number of equivalents of Fe3+ and each of the other metals ions were mixed with HPU-1. Here, in each case, the fluorescence was completely quenched (Fig. 3). This result showed that the interference from conventional metal ions can be neglected, confirming the superior selectivity of HPU-1 for Fe3+. Therefore, Fe3+ can be considered as a recognizable ion for studying its ability to quench the fluorescence of HPU-1 in suspension. The quenching of HPU-1 luminescence was examined for Fe3+ concentrations between 0 and 1000 μM. As shown in Fig. 4, the luminescence intensity of HPU-1 was essentially quenched upon the gradual addition of 100 μL Fe3+. The detection limit of HPU-1 for Fe3+ was calculated to be 1.09 × 10−3 mol L−1 (Fig. S5†), lower than those of most of the reported Fe3+-sensing MOFs.11 In addition, the quenching effect can be quantitatively explained by the Stern–Volmer equation,12 written as
where I0 and I are the luminescence intensities of the before-sensing and after-sensing products, respectively, [M] is the Fe3+ concentration, and KSV is the coefficient of quenching. The KSV value was calculated from the luminescence data to be 1.0 × 104 L mol−1 (Fig. S6†), indicating that Fe3+ had particularly strong quenching effect on the luminescence of HPU-1. Compared with the other reported Fe3+-sensing MOFs, HPU-1 was found to be a highly selective and sensitive probe for Fe3+ in aqueous solution (Table S3†).13
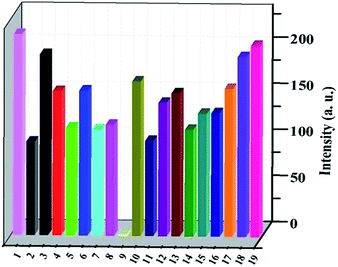 |
| Fig. 2 Fluorescence spectra of HPU-1 (2 mg) in aqueous solutions of various metal ions (3 mL, 10 mM). (1) HPU-1, (2) Ag+, (3) Al3+, (4) Ba2+, (5) Ca2+, (6) Co2+, (7) Cr3+, (8) Cu2+, (9) Fe3+, (10) Mn2+, (11) Ni2+, (12) Pb2+, (13) Cd2+, (14) K+, (15) Li+, (16) Mg2+, (17) Na+, (18) Eu3+, (19) Tb3+. | |
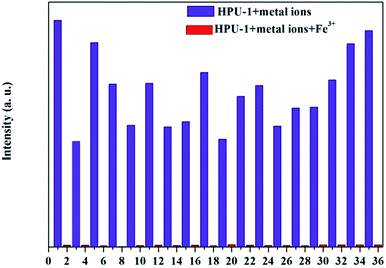 |
| Fig. 3 Fluorescence response of HPU-1 (2 mg) at a wavelength of 430 nm in the absence and presence of various metal ions ((1) HPU-1, (2) Fe3+, (3) Ag+, (5) Al3+, (7) Ba2+, (9) Ca2+, (11) Co2+, (13) Cr3+, (15) Cu2+, (17) Mn2+, (19) Ni2+, (21) Pb2+, (23) Cd2+, (25) K+, (27) Li+, (29) Mg2+, (31) Na+, (33) Eu3+, (35) Tb3+). Purple bars: a free sensor or a sensor treated with the marked ions. Red bars: a sensor treated with the marked metal ion followed by an equivalent of Fe3+. | |
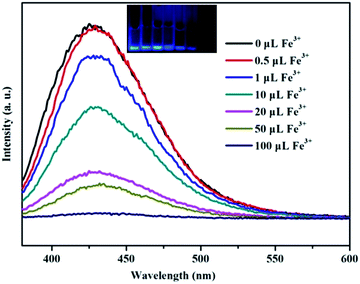 |
| Fig. 4 Fluorescence emission spectra of HPU-1 (2 mg) in aqueous solutions of various concentrations of Fe3+. | |
The quenching of the luminescence of HPU-1 by Fe3+ may have been due to (a) interactions between the sensed metal ions and organic ligands, (b) the collapse of the crystal structure, and/or (c) cation exchange of the central metal ions and the sensed metal ions.14 Therefore, to elucidate the possible mechanism for such photoluminescence quenching by Fe3+, we acquired powder XRD patterns of the samples of HPU-1 immersed in a 0.1 mol L−1 Fe3+ solution for 30 min. As shown in Fig. S7,† the PXRD patterns differed little from the original one, illustrating the remarkable quenching effect results from the transformation of the parent HPU-1 framework to Fe-MOF through the cation exchange of Zn2+ with Fe3+. To further test this hypothesis, ground powder samples of HPU-1 were immersed in a 0.01 mol L−1 Fe3+ aqueous solution. After carrying out this immersion for 0.5, 1, 2, 5, 16, and 24 h, the resulting filtrates were introduced for ICP determination. Table S4† shows the concentrations of Zn2+ and Fe3+ in the filtrate. The concentration of Zn2+ in the filtrated solution gradually increased as the level of Fe3+ was decreased. In addition, as shown in Fig. S8,† the PXRD patterns of the products of immersion for various amounts of time indicated that the structures gradually changed as the concentration of Fe3+ was changed in the products. Moreover, the colors of the products gradually deepened as the immersion time was increased. The SEM and EDS results showed the presence of Fe3+ in the products of 30 minutes of immersion (Fig. S9 and S10†). Combining these observations with the above experimental results, we proposed that the fluorescence quenching resulted from the cation exchange mechanism, where Fe3+ entered into the HPU-1 framework and replaced Zn2+, which lowered the energy transfer from the ligands to Zn2+, and thus led to the quenching effect (Fig. 5). Moreover, when Fe3+ was added into the aqueous solution of DPTMIA containing Zn2+, the fluorescence intensity was entirely quenched, which also verified that Fe3+ can exchange with Zn2+ (Fig. S11†).
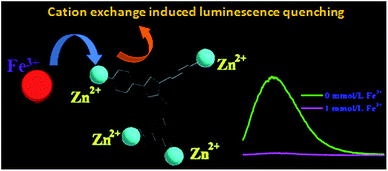 |
| Fig. 5 Schematic representation of the sensing mechanism. | |
Sensing for hazardous organic analytes
To determine the ability of HPU-1 to sense toxic organics and explosives, the HPU-1 powder was immersed in 0.01 mol L−1 aqueous solutions of 4-NP, 2,4-DNP, 1,3-DNB, 3-NP, 2,4-DNT,4-NB, 1,4-DMB, MB, 1,3,5-TMB, and NB for luminescence studies. As shown in Fig. 6 and S12,† 4-NP, 2, 4-DNP, and 3-NP showed large quenching effects compared with the other organic analytes, which indicated that HPU-1 selectively sensed phenolic compounds. In addition, the responses were not disturbed by other organic analytes (Fig. S13 and S14†). To examine the sensing sensitivity toward 4-NP or 2,4-DNP, a batch of suspensions of HPU-1 dispersed in aqueous solution with gradually increasing contents of 4-NP or 2,4-DNP was prepared and their emission spectra were recorded (Fig. S15 and S16†). The quenching constants calculated from the Stem–Volmer (SV) equation for 4-NP and 2,4-DNP were 1.45 × 105 and 1.074 × 104 L mol−1 (Fig. S17 and S18†), better than the values in the limited literature. In addition, the detection limits of HPU-1 for 4-NP and 2,4-DNP were calculated to be 6.31 × 10−4 and 9.86 × 10−4 mol L−1 (and about 1.67 × 10−3 mol L−1 in the literature) (Fig. S19 and S20†).15,9b As mentioned above, HPU-1 was observed to have large rectangular channels filled with guest molecules. Thus, the sensing mechanism likely involved guest molecules included in the channels exchanging with 4-NP or 2,4-DNP, resulting in the luminescence response. To test this assumption, samples of HPU-1 were soaked in 1 mg mL−1 ethanol solution of 4-NP or 2,4-DNP for 0, 1, 2, 3, 4, 5, 6, 7, 12, 24 and 72 h. Then, UV/vis absorption spectra of the filtrates were acquired and analyzed. Results showed that the absorption peaks of 4-NP or 2,4-DNP were nearly unchanged, demonstrating that 4-NP or 2,4-DNP was not absorbed in the cavity of HPU-1 (Fig. S21 and S22†). Thus, the quenching of the luminescence of Zn2+ may have been due to the hydrogen-bonding interactions between NP and the organic ligands resulting in a decrease in the energy transferred from the ligand to Zn2+ ions.16
 |
| Fig. 6 Fluorescence intensities of HPU-1 (2 mg) in aqueous solutions of various organic analytes (3 mL, 0.01 mol L−1). | |
Finally, the luminescence properties of HPU-1 in various solvents were investigated for examining the practicality of using HPU-1 in environmental systems. Well-ground samples of HPU-1 (2 mg) were dispersed in 2 mL of different solvents, and the emission spectra of HPU-1 excited at 350 nm revealed strong luminescence (Fig. S23†); these results showed these common solvents to have no effect on the luminescence of HPU-1. In addition, we also investigated the effect of pH on the fluorescence intensity of HPU-1. As shown in Fig. S24,† the fluorescence intensity was observed to be nearly the same in relatively acidic and alkaline environments. Therefore, the highly sensitive and selective sensing of Fe3+ and phenol compounds in aqueous solutions has provided the foundation for an efficient, straightforward, and real-time detection of trace amounts of this metal ion and hazardous class of compounds in environmental systems.
Conclusions
In conclusion, a water-stable two-fold interpenetrating network (HPU-1) with rectangular channels was synthesized and characterized. HPU-1 was found to be a highly selective and sensitive probe of Fe3+ in aqueous solution. Based on our observations and experimental results, we proposed a cation exchange mechanism for the fluorescence quenching. HPU-1 also showed highly selective and sensitive detection of phenol compounds in aqueous solution. Quenching of the fluorescence was proposed to result from a decrease in the efficiency of the transfer of electrons and energy from the ligands to the metal ions. This work demonstrated the potential application of a fluorescent Zn-MOF as a multi-responsive probe for the detection of Fe3+ and hazardous phenol compounds in aqueous media.
Conflicts of interest
There are no conflicts to declare.
Acknowledgements
This work was supported by the National Natural Science Foundation of China (Nos. 21601050), NSFC – Henan region mutual funds (U1604124), the key scientific research project of Henan higher education (16A150010), Science and technology research project of Henan province (152102210314).
References
-
(a) J. Wang, M. Jiang, L. Yan, R. Peng, J. Meng, F. Huang, X. X. X. Guo, Y. Li and P. Y. Wu, Inorg. Chem., 2016, 55, 12660–12668 CrossRef CAS PubMed;
(b) L. W. He, X. L. Yang, K. X. Xu and W. Y. Lin, Chem. Commun., 2017, 53, 4080–4083 RSC;
(c) L. Y. Wang, Y. Tian, L. M. Ding, B. Zhao, X. Y. He, B. Song and S. F. Liu, RSC Adv., 2017, 7, 16916–16923 RSC;
(d) S. C. Zhou, M. Zhang, F. Y. Yang, F. Wang and C. Y. Wang, J. Mater. Chem. C, 2017, 5, 2466–2473 RSC;
(e) B. D. Wang, J. Hai, Z. C. Liu, Q. Wang, Z. Y. Yang and S. H. Sun, Angew. Chem., Int. Ed., 2010, 49, 4576–4579 CrossRef CAS PubMed;
(f) Q. H. Tan, Y. Q. Wang, X. Y. Guo, H. T. Liu and Z. L. Liu, RSC Adv., 2016, 6, 61725–61731 RSC.
-
(a) D. M. Chen, N. N. Zhang, C. S. Liu and M. Du, J. Mater. Chem. C, 2017, 5, 2311–2317 RSC;
(b) J. L. Bricks, A. Kovalchuk, C. Trieflinger, M. Nofz, M. Buschel, A. L. Tolmachev, J. Daub and K. Rurack, J. Am. Chem. Soc., 2005, 127, 13522–13529 CrossRef CAS PubMed;
(c) A. Barba-Bon, A. M. Costero and S. Gil, Chem. Commun., 2012, 48, 3000–3002 RSC;
(d) R. F. Bogale, Y. Z. Chen, J. W. Ye, Y. Y. Yang, A. Rauf, L. Y. Duan, P. Tian and G. L. Ning, Sens. Actuators, B, 2017, 245, 171–178 CrossRef CAS.
-
(a) Q. Tang, S. X. Liu, Y. W. Liu, J. Miao, S. J. Li, L. Zhang, Z. Shi and Z. P. Zheng, Inorg. Chem., 2013, 52, 2799–2801 CrossRef CAS PubMed;
(b) X. Y. Dong, R. Wang, J. Z. Wang, S. Q. Zang and T. C. W. Mak, J. Mater. Chem. A, 2015, 3, 641–647 RSC;
(c) Y. T. Liang, G. P. Yang, B. Liu, Y. T. Yan, Z. P. Xi and Y. Y. Wang, Dalton Trans., 2015, 44, 13325–13330 RSC;
(d) Y. Shi, S. L. Chou, J. Z. Wang, D. Wexler, H. J. Li, H. K. Liu and Y. P. Wang, J. Mater. Chem., 2012, 22, 16920–16926 RSC;
(e) Y. J. Cui, Y. F. Yue, G. D. Qian and B. L. Chen, Chem. Rev., 2012, 112, 1126–1162 CrossRef CAS PubMed.
-
(a) J. M. Zhou, W. Shi, H. M. Li, H. Li and P. Cheng, J. Phys. Chem. C, 2014, 118, 416–426 CrossRef CAS;
(b) X. Feng, Y. Q. Feng, N. Guo, Y. L. Sun, T. Zhang, L. F. Ma and L. Y. Wang, Inorg. Chem., 2017, 56, 1713–1721 CrossRef CAS PubMed;
(c) Y. Q. Xiao, Y. J. Cui, Q. Zheng, S. C. Xiang, G. D. Qian and B. L. Chen, Chem. Commun., 2010, 46, 5503–5505 RSC;
(d) Z. M. Hao, X. Z. Song, M. Zhu, X. Meng, S. N. Zhao, S. Q. Su, W. T. Yang and H. J. Zhang, J. Mater. Chem. A, 2013, 1, 11043–11050 RSC;
(e) S. Mohapatra, B. Rajeswaran, A. Chakraborty, A. Sundaresan and T. K. Maji, Chem. Mater., 2013, 25, 1673–1679 CrossRef CAS;
(f) R. W. Huang, Y. S. Wei, X. Y. Dong, X. H. Wu, C. X. Du, S. Q. Zang and T. C. W. Mark, Nat. Chem., 2017, 9, 689–697 CrossRef CAS PubMed.
-
(a) Y. Zhou, H. H. Chen and B. Yan, J. Mater. Chem. A, 2014, 2, 13691–13697 RSC;
(b) X. Lian and B. Yan, Inorg. Chem., 2016, 55, 11831–11838 CrossRef CAS PubMed;
(c) Y. J. Li, Y. L. Wang and Q. Y. Liu, Inorg. Chem., 2017, 56, 2159–2164 CrossRef CAS PubMed;
(d) J. N. Hao and B. Yan, J. Mater. Chem. A, 2014, 2, 18018–18025 RSC;
(e) W. T. Xu, Y. F. Zhou, D. C. Huang, M. Y. Su, K. Wang and M. C. Hong, Inorg. Chem., 2014, 53, 6497–6499 CrossRef CAS PubMed.
-
(a) K. C. Wang, X. Tian, Y. H. Jin, J. Sun and Q. H. Zhang, Cryst. Growth Des., 2017, 4, 1836–1842 CrossRef;
(b) Y. Rachuri, B. Parmar, K. K. Bisht and E. Suresh, Cryst. Growth Des., 2017, 17, 1363–1372 CrossRef CAS;
(c) X. D. Zhu, K. Zhang, W. X. Zhou, Q. H. Li, Y. Q. Fu, R. M. Liu and G. X. Qian, Inorg. Chem. Commun., 2016, 75, 37–40 Search PubMed.
-
(a) A. K. Chaudhari, S. S. Nagarkar, B. Joarder and S. K. Ghosh, Cryst. Growth Des., 2013, 13, 3716–3721 CrossRef CAS;
(b) L. Wang, Z. Q. Yao, G. J. Ren, S. D. Han, T. L. Hua and X. H. Bu, Inorg. Chem. Commun., 2016, 65, 9–12 CrossRef CAS.
-
(a) L. J. Han, W. Yan, S. G. Chen, Z. Z. Shi and H. G. Zheng, Inorg. Chem., 2017, 56, 2936–2940 CrossRef CAS PubMed;
(b) B. Lv, X. F. Wang, H. M. Hu, Y. F. Zhao, M. L. Yang and G. L. Xue, Inorg. Chim. Acta, 2016, 453, 771–778 CrossRef CAS.
-
(a) J. L. Yu, X. Y. Wang, Q. Kang, J. H. Li, D. Z. Shen and L. X. Chen, Environ. Sci.: Nano, 2017, 4, 493–502 RSC;
(b) Q. H. Tan, Y. Q. Wang, X. Y. Guo, H. T. Liu and Z. L. Liu, RSC Adv., 2016, 6, 61725–61731 RSC.
-
(a) G. Y. Wang, L. L. Yang, Y. Li, H. Song, W. J. Ruan, Z. Chang and X. H. Bu, Dalton Trans., 2013, 42, 12865–12868 RSC;
(b) S. S. Nagarkar, A. V. Desai and S. K. Ghosh, Chem. Commun., 2014, 50, 8915–8918 RSC;
(c) W. Xie, S. R. Zhang, D. Y. Du, J. S. Qin, S. J. Bao, J. Li, Z. M. Su, W. W. He, Q. Fu and Y. Q. Lan, Inorg. Chem., 2015, 54, 3290–3296 CrossRef CAS PubMed.
- M. Zheng, H. Q. Tan, Z. G. Xie, L. G. Zhang, X. B. Jing and Z. C. Sun, Inorg. Chem., 2017, 56, 2936–2940 CrossRef PubMed.
-
(a) S. S. Nagarkar, A. V. Desai and S. K. Ghosh, Chem. Commun., 2014, 50, 8915–8918 RSC;
(b) T. D. Gauthier, E. C. Shane, W. F. Guerin, W. R. Seitz and C. L. Grant, Environ. Sci. Technol., 1986, 20, 1162–1166 CrossRef CAS;
(c) J. Keizer, J. Am. Chem. Soc., 1983, 105, 1494–1498 CrossRef CAS.
-
(a) S. K. Sahoo, D. Sharma, R. K. Bera, G. Crisponi and J. F. Callan, Chem. Soc. Rev., 2012, 41, 7195–7227 RSC;
(b) M. Zheng, H. Q. Tan, Z. G. Xie, L. G. Zhang, X. B. Jing and Z. C. Sun, ACS Appl. Mater. Interfaces, 2013, 5, 1078–1108 CrossRef CAS PubMed;
(c) Y. Zhou, H. H. Chen and B. Yan, J. Mater. Chem. A, 2014, 2, 13691–13697 RSC.
- L. H. Cao, F. Shi, W. M. Zhang, S. Q. Zang and T. C. W. Mak, Chem.–Eur. J., 2015, 21, 15705–15712 CrossRef CAS PubMed.
- Y. Q. Wang, Q. H. Tan, H. T. Liu, W. Sun and Z. L. Liu, RSC Adv., 2015, 5, 86614–86619 RSC.
-
(a) S. N. Zhao, X. Z. Song, M. Zhu, X. Meng, L. L. Wu, S. Y. Song, C. Wang and H. J. Zhang, RSC Adv., 2015, 5, 93–98 RSC;
(b) B. W. Xu, X. F. Wu, H. B. Li, H. Tong and L. X. Wang, Macromolecules, 2011, 44, 5089–5092 CrossRef CAS.
Footnote |
† Electronic supplementary information (ESI) available: Selected bond lengths and bond angles, additional figures, powder X-ray patterns for the complex. CCDC 1542239 for HPU-1. For ESI and crystallographic data in CIF or other electronic format see DOI: 10.1039/c7ra08427g |
|
This journal is © The Royal Society of Chemistry 2017 |
Click here to see how this site uses Cookies. View our privacy policy here.