DOI:
10.1039/C7RA08707A
(Paper)
RSC Adv., 2017,
7, 46148-46154
A novel fluorescence turn-on probe for the selective detection of thiophenols by caged benzooxazolidinoindocyanine†
Received
7th August 2017
, Accepted 23rd September 2017
First published on 28th September 2017
Abstract
Based on a strategy involving 2,4-dinitrophenyl ether and functionalized caged benzooxazolidinoindocyanine, a fluorescence turn-on probe for the selective detection of thiophenols was developed. Using the chemical properties of thiophenols, which are able to induce aromatic nucleophilic substitution (SNAr), and the principle of chemical-reaction-induced skeletal rearrangement, the probe (PBO) exhibits excellent selectivity for thiophenols with a detection limit of 7 nM. This probe features dramatic color changes and a significant fluorescence turn-on response (120 fold) after being treated with thiophenol. The applications of this new probe were demonstrated by the detection of thiophenol in real water samples and by fluorescence imaging of thiophenol in BEL-7402 cells.
Introduction
Thiophenols are extensively used in organic synthesis for preparing various products in the agrochemical, pharmaceutical and dye industries.1–4 Although aliphatic thiols, such as cysteine, homocysteine and glutathione, are important biological molecules with a range of biological functions,5–9 thiophenols are a class of highly toxic and polluting compounds. The studies of their toxicity in fish and mouse reveal that the median lethal dose (LC50) values range from 0.01 to 0.4 mM or 46.2 mg kg−1.10,11 Prolonged exposure to thiophenol liquid and vapor in water or soil is highly detrimental to human health, and triggers a series of serious systemic injuries, including damage to the central nervous system, muscle weakness and even death.12,13 Considering their high toxicity and the emerging concerns about the environment, easy, simple, rapid and highly sensitive and selective methods for the detection of thiophenols are therefore of great importance in both environmental and biological sciences.
Recently, fluorescent probes have attracted great attention due to their easy operation, high sensitivity, high selectivity and low cost.14 Though there are many fluorescent probes for thiols have been developed in the past few decades,15–18 Wang and co-workers reported the first fluorescent probe for selective detection of thiophenols over aliphatic thiols, which was based on sulfonamide that was cleaved by a thiolate anion through an SNAr process.19 Since then, a variety of fluorescent probes have been developed to selectively discriminate thiophenols from aliphatic thiols and been demonstrated in some applications.20–31 However, most of these probes are based on nucleophilic reactions with sulfonamide, sulfonate, or the ether group with thiolate anions of aromatic thiols, resulting in colorimetric or fluorometric responses. But the probes containing sulfonamide and sulfonate groups always release toxic SO2 gas after the reaction with thiophenols and have low selectivity and sensitivity for thiophenols. However, the group of 2, 4-dinitrophenyl ether has several virtues for the development of fluorescent probes for thiophenols, such as good selectivity, low detection limit, easy synthesis and low-cost.20,32–35 On the other hand, selective fluorescent probes for thiophenols over aliphatic thiols are possible because the pKa value of thiophenol is about 6.5, which is lower than that of aliphatic thiols (pKa of ca. 8.5).24,32,36 Thus, cleavage of the groups of sulphonamide or sulfonate or dinitrophenyl ether is more fast for thiophenols than aliphatic thiols under pH 7–8.34,37
To our best knowledge, most of the fluorescent probes for the detection of thiophenols were designed on the basis of intramolecular charge transfer (ICT), photoinduced electron transfer (PET), through-bond energy transfer (TBET) and fluorescence resonance energy transfer (FRET) mechanism. In this work, we describe a selective probe PBO for thiophenols based on benzooxazolidinoindocyanine-conjugated phenol. And the phenol moiety is caged with a 2,4-dinitrophenyl group for the selective recognition of thiophenols. After the addition of thiophenols, probe PBO exhibited rapid and dramatic fluorescence turn-on responses and obvious color changes. It features high selectivity for thiophenols over aliphatic thiols and NaHS by benzooxa-zolidinoindocyanine-to-hydroxyindolium transformation (Scheme 1). This chemical-reaction-induced skeletal rearrangement system (Scheme 1a) is contrast to the functional group transformation system developed by Chang et al. (Scheme 1b),38–40 and the light or thermal driven transformation system pioneered by Raymo et al. (Scheme 1c).41–43 Through the skeletal rearrangement of probe PBO, dramatic color changes and strong fluorescence responses (120 fold) have been exhibited in the presence of chemical inducer thiophen-ols, along with excellent selectivity for thiophenols and a low detection limit of 7 nM. The probe PBO was also successfully applied to the detection of thiophenol in water samples and fluorescent imaging of thiophenol in living cells.
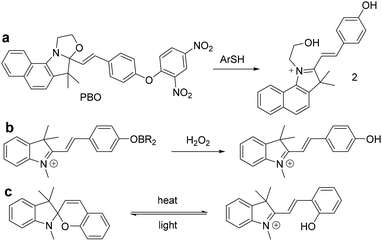 |
| Scheme 1 Strategic approaches to the optical probes. | |
Experimental
Materials and instrumentation
The chemicals were purchased from Aladdin (Shanghai, China) and used without further purification. Solvents used were purified by standard methods prior to use. Double-distilled water was used for all experiments. UV-vis absorption spectra were collected on a UV-2450 spectrophotometer (Shimadzu Co., Japan). Fluorescence spectra were recorded on TECAN-M1000 spectrophotometer (Tecan, Switzerland). The 1H NMR and 13C NMR spectra were obtained with tetramethyl silane (TMS) as the internal standard on a BRUKER AVANCE-500 spectrometer and the chemical shifts (δ) were expressed in ppm and coupling constants (J) in hertz. IR spectrum was recorded on NEXUS as KBr pellets and were reported in cm−1. High resolution mass spectrometric (HRMS) was performed using an AB TripleTOF 5600+ mass spectrometer (U.S AB SCIEX, CH3OH was used for the HRMS). Thin layer chromatography (TLC) analysis was performed on silica gel plates and column chromatography was conducted over silica gel (mesh 200–300). All pH measurements were carried out on a Model pHS-3C pH meter (Shanghai, China). The probe PBO was characterized by 1H NMR, 13C NMR, IR and HRMS (Fig. S12–14 in ESI†). The fluorescence imaging of cells was conducted on an inverted fluorescence microscopy (NIKON Eclipse Ti-S, Japan).
Synthesis of 3-(2-hydroxyethyl)-1,1,2-trimethyl-1H-benzo[e]indol-3-ium bromide (1)
2,3,3-Trimethylbenzoindolenine (10.00 g, 47.78 mmol) and 2-bromoethanol (7.17 g, 57.34 mmol) were dissolved in acetonitrile (40 mL). The mixture was stirred and refluxed for 24 h until no starting materials were indicated by TLC. The reaction mixture was cooled to room temperature and needle-like crystals were formed. Then the solid was filtered, washed with ether, and dried under vacuum to give the target compound (10.1 g, 63.2%). 1H NMR (500 MHz, DMSO-d6) δ 8.38 (d, J = 8.4 Hz, 1H), 8.28 (d, J = 8.9 Hz, 1H), 8.21 (d, J = 8.2 Hz, 1H), 8.17 (d, J = 8.9 Hz, 1H), 7.78 (m, 1H), 7.72 (m, 1H), 4.74 (t, J = 4.9 Hz, 2H), 3.94 (t, J = 5.1 Hz, 2H), 2.95 (s, 3H), 1.78 (s, 6H). 13C NMR (126 MHz, DMSO-d6) δ 198.14, 139.12, 137.29, 133.45, 130.95, 130.18, 128.84, 127.67, 123.87, 114.03, 58.44, 56.05, 51.02, 22.10, 14.83.
Synthesis of (E)-3-(2-hydroxyethyl)-2-(4-hydroxystyryl)-1,1-dimethyl-1H-benzo[e]indol-3-ium bromide (2)
To a solution of compound 3-(2-hydroxyethyl)-1,1,2-trimethyl-1H-benzo[e]indol-3-ium bromide (3.00 g, 8.98 mmol) and 4-hydroxybenzaldehyde (1.32 g, 10.77 mmol) in ethanol (25 mL) was added a few drops of piperidine. The mixture was stirred for overnight at room temperature until all of the starting compounds were consumed. Then the residue was filtered and washed with cold ethanol three times. The solid was dried under vacuum to give the orange product (3.1 g, 78.8%). 1H NMR (500 MHz, DMSO-d6) δ 10.78 (s, 1H), 8.49 (d, J = 16.2 Hz, 1H), 8.41 (d, J = 8.5 Hz, 1H), 8.25 (d, J = 8.9 Hz, 1H), 8.20 (d, J = 8.2 Hz, 1H), 8.12 (d, J = 8.4 Hz, 2H), 8.07 (d, J = 8.9 Hz, 1H), 7.80 (t, J = 7.7 Hz, 1H), 7.70 (t, J = 7.5 Hz, 1H), 7.57 (d, J = 16.2 Hz, 1H), 6.98 (d, J = 8.4 Hz, 2H), 5.24 (s, 1H), 4.87 (t, J = 5.0 Hz, 2H), 3.94 (t, J = 5.0 Hz, 2H), 2.03 (s, 6H). 13C NMR (126 MHz, DMSO-d6) δ 183.49, 163.04, 152.62, 138.76, 137.67, 133.37, 132.90, 130.62, 129.97, 128.30, 126.89, 126.70, 126.07, 122.95, 116.40, 113.50, 109.40, 58.82, 53.48, 49.04, 25.94.
Synthesis of (E)-10a-(4-(2,4-dinitrophenoxy)styryl)-11,11-dimeth-yl-8,9,10a,11-tetrahydrobenzo[e]oxazolo[3,2-a]indole (probe PBO)
To a solution of compound (E)-3-(2-hydroxyethyl)-2-(4-hydroxystyryl)-1,1-dimethyl-1H-benzo[e]indol-3-ium bromide (2) (428 mg, 0.97 mmol), 2,4-dinitrofluorobenzene (200 mg, 1.07 mmol) and anhydrous sodium carbonate (207 mg 1.95 mmol) in acetone (25 mL) was added a few drops of triethylamine. Then the mixture was heated to reflux for 5 h. After cooled to room temperature, the residue was filtered and the filtrate was concentrated to afford the crude product, which was purified by flash column chromatography (PE
:
EA = 10
:
1, v/v) to afford the yellow probe PBO (300 mg, 58.7%). 1H NMR (500 MHz, CDCl3) δ 8.86 (d, J = 2.7 Hz, 1H), 8.32 (dd, J = 9.3, 2.8 Hz, 1H), 8.02 (d, J = 8.5 Hz, 1H), 7.83 (dd, J = 8.2, 1.2 Hz, 1H), 7.75 (d, J = 8.6 Hz, 1H), 7.59 (m, 2H), 7.46 (ddd, J = 8.4, 6.7, 1.3 Hz, 1H), 7.31 (ddd, J = 8.0, 6.8, 1.1 Hz, 1H), 7.14 (dd, J = 8.5, 5.8 Hz, 3H), 7.07 (d, J = 9.2 Hz, 1H), 6.96 (d, J = 15.9 Hz, 1H), 6.41 (d, J = 15.9 Hz, 1H), 3.86 (m, 2H), 3.63 (q, J = 7.8, 7.8, 7.2 Hz, 1H), 3.53 (dt, J = 12.2, 8.3 Hz, 1H), 1.82 (s, 3H), 1.41 (s, 3H). 13C NMR (126 MHz, CDCl3) δ 156.24, 153.25, 147.55, 135.29, 131.19, 131.04, 130.41, 129.98, 129.60, 129.39, 129.11, 128.94, 127.52, 126.55, 123.05, 122.32, 122.27, 120.93, 118.70, 114.08, 110.30, 63.69, 50.36, 49.91, 26.63, 21.80. IR (KBr pellet, cm−1): 3274, 2971, 2883, 1619, 1535, 1470, 1342, 1263, 1193, 926, 821, 746. HRMS: m/z calcd for C30H25N3O6 [M + H]+ 524.1821; found 524.1821.
General procedure for UV-visible and fluorescence measurements
UV-vis absorption and fluorescence spectra studies were conducted in a mixture solution of CH3CN/PBS (10 mM, pH = 7.4, 5
:
5, v/v). Probe PBO was dissolved in dimethyl sulfoxide (DMSO) to get the stock solution (1 × 10−3 M). Deionized water was used to prepare stock solutions (1 × 10−2 M) of NaF, NaCl, NaBr, NaNO3, NaNO2, NaOAc, Na2CO3, NaHCO3, Na2SO4, Na2SO3, NaHSO4, NaHSO3, Na2S2O3, Na2S2O8, NaCN, NaHS, NaOCl, Na3PO4, Cys and GSH. DMSO was used to prepare stock solution (1 × 10−2 M) of p-nitrophenol, 4-chlorothiophenol, 2-aminothiophenol, p-toluenethiol, aniline and thiophenol. These stock solutions were further diluted to required concentration for measurement. Test solution was prepared by placing 20 μL of the stock solution and an appropriate aliquot of each specie solution into a 2 mL sample tube, and the solution was diluted to 2 mL with CH3CN containing 50% PBS buffer. Spectra data were recorded in an indicated time (20 min) after the addition of analytes.
Cell culture and fluorescence imaging
The BEL-7402 cells obtained from China Center for Type Culture Collection (Wuhan, China) were cultured in a humid atmosphere of 5% CO2 at 37 °C in Dulbecco's modified eagle medium (DMEM) supplemented with 10% heat-inactivated fetal bovine serum (FBS) and streptomycin/penicillin (100 μg mL−1). Cell cytotoxicity was evaluated by MTT assay. Cells were cultivated in a 96-well plate until 50–70% confluence, and then incubated with different concentrations of PBO (0–10 μM) for 24 h. Then 20 μL 3-(4,5-dimethyl-2-thiazolyl)-2,5-diphenyltetrazolium bromide (MTT, 0.5 mg mL−1) was added for 4 h at 37 °C. After removing the MTT, 150 μL DMSO was added. Absorbance was measured at 492 nm with a Varioskan Flash (Thermo Fisher Scientific, Waltham, MA, USA). All experiments were repeated three times, and the data are presented as the percentages of the experimental viability of the treated cells compared with that of the control cells. 70–80% confluent cell monolayers in 24-well plate were exposed to 10 μM probe PBO for 30 min at 37 °C. For another group of cells, after cells were exposed to probe PBO for 30 min, thiophenol (0.05 mM) was added and incubated for another 30 min at 37 °C, then washed with phosphate-buffered saline (PBS) for three times.
Results and discussion
Design and synthesis of the probe
From Wang's work,36 we know that 2,4-dinitrophenyl derivatives are essential for the high reactivity and selectivity of the thiolate-mediated SNAr reaction and serve as an effective quencher of the PET or ICT sensor. We hypothesized that the reaction of the nucleophilic oxygen group with an electron-withdrawing 2,4-dinitrophenyl and caged benzooxazolidinoindocyanine would result in a nonfluorescent molecule. The resulting 2,4-dinitrophenyl ether could be readily cleaved by thiophenol and underwent a chemical-reaction-induced skeletal rearrangement process under the physiological pH conditions through SNAr reaction, and a fluorescence turn-on would happen. Moreover, under the advantages of the unique reactivity profile and rational design, the fluorescent probe features of high sensitivity and selectivity for thiophenols, which is based on chemical-reaction-induced skeletal rearrangement. Due to cyanine derivatives' excellent photophysical properties, outstanding biocompatibility, and low toxicity to living systems,44 probe PBO was designed and easily synthesized by using cyanine derivatives as the fluorophore moiety. And the group of 2,4-dinitrophenyl ether was utilized both as the selectivity recognition unit for thiophenols and a fluorescence quencher (Scheme 2).
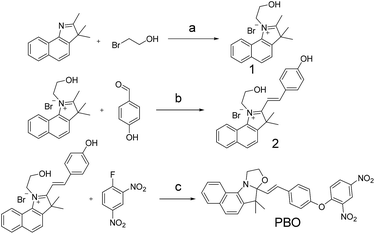 |
| Scheme 2 Synthesis of probe PBO. Reagents and conditions: (a) CH3CN, reflux, 63.2%; (b) piperidine, absolute ethanol, r.t., 78.8%. (c) Na2CO3, triethylamine, acetone, reflux, 58.7%. | |
Spectral properties of probe PBO
With probe PBO in hand, we firstly investigated its absorbance in the absence and presence of thiophenol. The free probe PBO exhibited near zero absorbance at 558 nm, while a well-defined strong absorbance maximum at 558 nm was obtained after treated with 10 equiv. of thiophenol (Fig. 1a). And the color of PBO solution was changed from colorless to red under visible light (inset, Fig. 1a), which could be easily observed by naked eyes. The effect of the ratio of acetonitrile with PBS buffer was also examined, and it was found that 1
:
1 ratio would give the best result (Fig. S1 in ESI†), for the skeleton-rearrangement would be proceeded smoothly under water-contained conditions. Correspondingly, the kinetic studies indicated that probe PBO could be shortly reacted with thiophenol at room temperature in physiological pH conditions (Fig. S2 in ESI†). It would reach to a plateau after 17 min. Therefore, reaction time of 20 min was selected to conduct the following experiments. Notably, probe PBO exhibited almost near zero fluorescence in the absence of thiophenol (λex = 530 nm). While, after the treatment of thiophenol (100 μM, 10.0 equiv.), a significant fluorescence turn-on at 580 nm (λex = 530 nm) (120 fold) was observed after 20 min (Fig. S2 in ESI†). The absorption and fluorescence response of probe PBO to various concentrations of thiophenol was further studied (Fig. S3 in ESI†). Drastic fluorescence increasement was displayed in a concentration from 0 μM to 4 μM, and the linear correlation between the thiophenol concentrations and fluorescence intensities showed the good sensitivity of probe PBO to thiophenol (Fig. 1b). Another linear calibration graph for the response of probe PBO to thiophenol concentrations ranging from 4 μM to 60 μM was also obtained (Fig. 1b), indicating that probe PBO could be potentially applied to quantitatively detect thiophenol with a range from nanomolar to micromolar. The detection limit was calculated to be as low as 7 nM (S/N = 3) (Fig. 1b), which was much lower than some reported probes.23,24,35,45 The comparison of recently reported fluorescent probes for thiophenols is summarized in Table S1 (ESI†). Thus probe PBO showed excellent performance for the sensing of thiophenol.
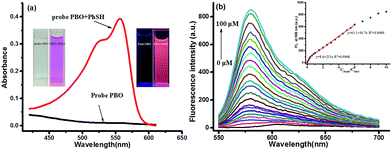 |
| Fig. 1 (a) Absorption spectra of the probe PBO (10 μM) and probe PBO (10 μM) with PhSH (10 equiv.) in CH3CN–PBS (10 mM, pH = 7.4, v/v, 5 : 5) after 20 min at room temperature. (b) Fluorescence spectra of probe PBO (10 μM) in the presence of different concentrations of PhSH in CH3CN–PBS (10 mM, pH = 7.4, v/v, 5 : 5). (λEx = 530 nm, λEm = 580 nm). Slit (nm): 10.0/10.0. Inset: (a) left: visual changes of probe PBO in the presence of PhSH (10 equiv.) by naked eyes. Right: fluorescence color change of probe PBO (10 μM) in 10 equiv. of PhSH under UV lamp. (b) The linear changes of the fluorescence intensity of probe PBO at 580 nm and as a function of PhSH concentration. | |
Effect of pH
To further evaluate the performance of the probe, we studied the fluorescence spectra of probe PBO in the absence and presence of thiophenol in different pH. Encouragingly, we found that almost no fluorescence enhancement was observed for probe PBO under pH ranging from 2.0 to 10.0, indicating that probe PBO was very stable with a wide range of pH. In contrast, bigger fluorescence increasement was observed when pH was 6.0 to 8.0 due to the strong ionization of thiophenol, which favoured the SNAr reaction (Fig. S4 in ESI†). These results showed that probe PBO could be applied to the detection of thiophenol under physiological pH condition.
Selectivity and interference
The selectivity of probe PBO is crucial to the sensing of thiophenol in complex environments. Various analytes including thiophenol derivatives (thiophenol, 2-aminothiophenol, p-toluenethiol and 4-chlorothiophenol), aliphatic thiols including cysteine (Cys) and glutathione (GSH), other potential interfering substances such as NaF, NaCl, NaBr, NaNO3, NaNO2, NaOAc, NaHSO3, Na2S2O8, Na2SO4, Na2SO3, NaHSO4, NaHCO3, Na2S2O3, p-nitrophenol, NaOCl, aniline, NaCN, Na3PO4, Na2CO3 and NaHS were investigated. Encouragingly, except thiophenols, addition of other analyates induced no marked fluorescence turn-on (Fig. 2). Notably, aliphatic thiols, such as Cys and GSH, did not trigger any fluorescence response, which indicated that probe PBO was highly selective for thiophenols. It should be noted that PBO showed a small fluorescence increasement to NaHS (25 equiv.) (Fig. 2a), which meant that HS− could cleave the dinitrophenyl ether via thiolysis reaction with low reaction rate and it was also consistent with previous reports,46–48 Moreover, based on these results, the effect of competitive species for the probe PBO with PhSH was also investigated, little fluorescence changes were observed except only small fluorescence decreasement in the presence of NaHS (λex = 530 nm, λem = 580 nm) (Fig. 2b). Therefore, probe PBO possessed high selectivity for the sensing of thiophenols and strong interference immunity to other interfering species. In addition, obvious color changes of the probe PBO solution was also observed only with thiophenols under visible light (Fig. S5 in ESI†). All of these results indicated that probe PBO holds the potential for the selective detection of thiophenols in biology and environment.
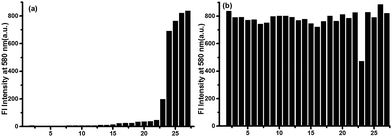 |
| Fig. 2 (a) Histogram representing the fluorescence intensity changes of probe PBO (10 μM) at 580 nm in the presence of different analytes (10.0 equiv.), and (b) probe PBO at 580 nm in the presence of 10.0 equiv. of PhSH upon the addition of different analytes (10.0 equiv.) of (1) black, (2) F−, (3) Cl−, (4) Br−, (5) NO3−, (6) NO2−, (7) OAc−, (8) HSO3−, (9) S2O82−, (10) SO42−, (11) SO32−, (12) HSO4−, (13) HCO3−, (14) S2O32−, (15) p-nitrophenol, (16) OCl−, (17) Cys, (18) aniline, (19) GSH, (20) CN−, (21) PO43−, (22) CO32−, (23) HS−, (24) 2-aminothiophenol, (25) p-toluenethiol, (26) PhSH, (27) 4-chlorothiophenol in CH3CN–PBS (10 mM, pH = 7.4, v/v, 5 : 5) with λex = 530 nm, λem = 580 nm. Slit (nm): 10.0/10.0. | |
Mechanism
In order to verify the mechanism for the probe PBO with thiophenol is indeed due to chemical-reaction-induced skeletal rearrangement and the conversion of probe PBO to compound 2 as depicted in Scheme 3. Firstly, we performed 1H NMR titration studies. Upon addition of excess PhSH to probe PBO, H′a and H′b of probe PBO were changed from multi-peak to triple-peak. H′c and H′d of probe PBO were also changed from two peaks to a single peak, which is consistent with the spectrum of compound 2 (Fig. 3). These results indicated that the thiolysis of dinitrophenyl ether and chemical-reaction-induced skeletal rearrangement would be happened. In addition, the product of the reaction of probe PBO with thiophenol was isolated by column chromatography, and further confirmed by 1H NMR, 13C NMR, high resolution mass spectrometry analysis (Fig. S15–17 in ESI†). Judging from NMR characterizations, it was noted that the isolated product was the intermediate, which could be stable under anhydrous conditions.49 Without water, the intermediate only exhibited a small absorption band at 338 nm in acetonitrile. However, along with the increase of water, a new absorption band centred at 559 nm is observed, and a significant fluorescence is enhanced at 580 nm (Fig. S6 in ESI†). This skeletal rearrangement process was further demonstrated by 1H NMR titration (Fig. S18 in ESI†). Thus, fluorescent probe PBO could be transformed by inducer thiophenol to afford strong fluorescent compound 2 under water contained conditions, along with 120-fold fluorescence enhancement.
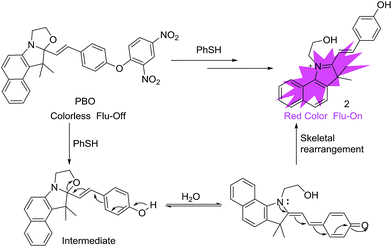 |
| Scheme 3 Proposed sensing mechanism. | |
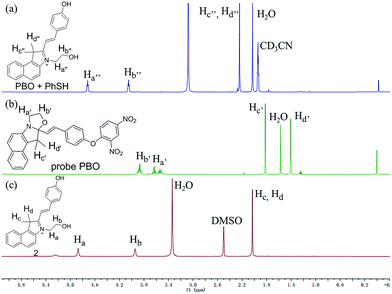 |
| Fig. 3 Partial 1H NMR spectra comparison of (a) probe PBO upon treatment with excess PhSH in CD3CN and D2O (10/1, v/v). (b) Probe PBO in CDCl3 and (c) compound 2 in DMSO-d6. | |
Detection of thiophenol in water samples
In order to evaluate the practicality of this probe, we firstly employed probe PBO to detect thiophenol in water samples from Xiang River and Taozi Lake. The water samples were directly tested, and then spiked with different concentrations of thiophenol (0.05 μM, 0.5 μM and 5 μM). As shown in the Table 1, the recoveries of thiophenol were ranged from 96% to 101%. It was observed that thiophenol in water could be measured with good recovery. And probe PBO could be applied to the quantitative detection of thiolphenol and its analogues in water.
Table 1 Analyses of thiophenol concentrations in real water samples
Sample |
Thiophenol spiked (μM) |
Thiophenol recovered (μM) |
Recovery (%) |
Xiang River |
0 |
Not detected |
|
0.05 |
0.049 ± 0.011 |
98 |
0.5 |
0.505 ± 0.013 |
101 |
5 |
4.88 ± 0.094 |
98 |
Taozi Lake water |
0 |
Not detected |
|
0.05 |
0.048 ± 0.009 |
96 |
0.5 |
0.49 ± 0.019 |
98 |
5 |
4.89 ± 0.14 |
98 |
Cell fluorescence imaging of thiophenol
To further investigate the membrane permeability of probe PBO and its ability to detect thiophenol in living cells, cell imaging experiments were carried out. Firstly, the cytotoxicity of probe PBO for BEL-7402 cells were investigated, and the results from MTT assays showed that the cells remained higher viability upon treatment with probe PBO (0–10.0 μM) as long as 24 h (Fig. S7 in ESI†), indicating that probe PBO has low cytotoxicity to living cells within a short time. Moreover, probe PBO showed little fluorescence response to other sulfur-containing species such as SO2 derivatives, NaHS, Cys and GSH, which promised the probe PBO for specific recognition of thiophenol in live cells. Initially, BEL-7402 cells were treated with probe PBO. As a control, fluorescent images of BEL-7402 cells treated with and without thiophenol were both taken and compared. It clearly showed that the probe displayed non-fluorescence without thiophenol (Fig. 4b), while strong green fluorescence was displayed after treated with thiophenol (Fig. 4d). These results demonstrated that probe PBO has good cell-permeability and could be applied to detect thiophenols in living cells.
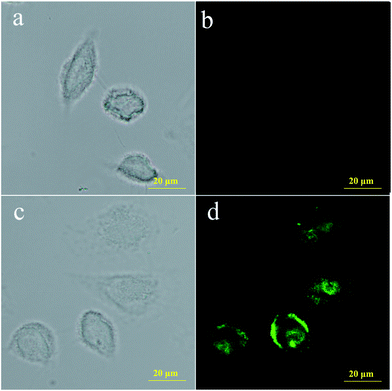 |
| Fig. 4 Fluorescence imaging of thiophenol in BEL-7402 cells using probe PBO. (a) Bright field image of BEL-7402 cells incubated with 10 μM probe PBO for 30 min; (b) fluorescence image of cells treated with 10 μM probe PBO for 30 min; (c) bright flied image of BEL-7402 cells exposed to 10 μM probe PBO for 30 min, subsequently incubated with 0.05 mM thiophenol for 30 min; (d) fluorescence image of BEL-7402 cells pretreated with 10 μM probe PBO for 30 min, subsequently incubated with 0.05 mM thiophenol for 30 min. The fluorescence images were captured with fluorescence microscope (λex = 505 nm, λem = 512–558 nm for green channel). Scale bar: 20 μm. | |
Conclusions
In summary, we have designed and synthesized a novel fluorescence turn-on probe based on 2,4-dinitrophenyl ether and functionalized caged benzooxazolidinoindocyanine for the selective detection of thiophenols. The probe PBO exhibited rapid and significant fluorescence turn-on responses to thiophenols (120 fold) at physiological pH condition via the effective thiolysis of dinitrophenyl ether and chemical-reaction-induced skeletal rearrangement. The probe featured excellent selectivity for thiophenols over aliphatic thiols and high sensitivity with a very low detection limit (LOD = 7 nM). In addition, this probe could be applied to detect thiophenol in real water samples and in living cells. Therefore, this work provides a new fluorescent probe for the rapid detection of toxic thiophenols in environmental and biological samples.
Conflicts of interest
There are no conflicts to declare.
Acknowledgements
This work was supported by the funding from the National Natural Science Foundation of China (Grant No. 21405043, 21675051, 21375037 and 81370532) and K. C. Wong Magna Fund in Ningbo University.
Notes and references
- I. Rahman and W. MacNee, Free Radical Biol. Med., 2000, 28, 1405–1420 CrossRef CAS PubMed.
- J. C. Love, L. A. Estroff, J. K. Kriebel, R. G. Nuzzo and G. M. Whitesides, Chem. Rev., 2005, 105, 1103–1169 CrossRef CAS PubMed.
- K. Shimada and K. Mitamura, J. Chromatogr. B: Biomed. Sci. Appl., 1994, 659, 227–241 CrossRef CAS PubMed.
- A. Eychmuller and A. L. Rogach, Pure Appl. Chem., 2000, 72, 179–188 CrossRef CAS.
- M. H. Lee, J. H. Han, J. H. Lee, H. G. Choi, C. Kang and J. S. Kim, J. Am. Chem. Soc., 2012, 134, 17314–17319 CrossRef CAS PubMed.
- S. Shahrokhian, Anal. Chem., 2001, 73, 5972–5978 CrossRef CAS PubMed.
- C. Yin, F. Huo, J. Zhang, R. Martinez-Manez, Y. Yang, H. Lv and S. Li, Chem. Soc. Rev., 2013, 42, 6032–6059 RSC.
- S. Y. Lim, K. H. Hong, D. I. Kim, H. Kwon and H. J. Kim, J. Am. Chem. Soc., 2014, 136, 7018–7025 CrossRef CAS PubMed.
- C. Y. Kim, H. J. Kang, S. J. Chung, H.-K. Kim, S.-Y. Na and H.-J. Kim, Anal. Chem., 2016, 88, 7178–7182 CrossRef CAS PubMed.
- T. P. Hell and R. C. Lindsay, J. Environ. Sci. Health, Part B, 1989, 24, 349–360 CrossRef.
- R. Munday, Free Radical Biol. Med., 1989, 7, 659–673 CrossRef CAS PubMed.
- P. Amrolia, S. G. Sullivan, A. Stern and R. Munday, J. Appl. Toxicol., 1989, 9, 113–118 CrossRef CAS PubMed.
- R. Munday and E. Manns, J. Appl. Toxicol., 1985, 5, 414–417 CrossRef CAS PubMed.
- A. P. de Silva, H. Q. N. Gunaratne, T. Gunnlaugsson, A. J. M. Huxley, C. P. McCoy, J. T. Rademacher and T. E. Rice, Chem. Rev., 1997, 97, 1515–1566 CrossRef CAS PubMed.
- Y. Yang, Q. Zhao, W. Feng and F. Li, Chem. Rev., 2013, 113, 192–270 CrossRef CAS PubMed.
- L.-Y. Niu, Y.-Z. Chen, H.-R. Zheng, L.-Z. Wu, C.-H. Tung and Q.-Z. Yang, Chem. Soc. Rev., 2015, 44, 6143–6160 RSC.
- Y. Zhou and J. Yoon, Chem. Soc. Rev., 2012, 41, 52–67 RSC.
- H. S. Jung, X. Chen, J. S. Kim and J. Yoon, Chem. Soc. Rev., 2013, 42, 6019–6031 RSC.
- W. Jiang, Q. Fu, H. Fan, J. Ho and W. Wang, Angew. Chem., Int. Ed., 2007, 46, 8445–8448 CrossRef CAS PubMed.
- W. Lin, L. Long and W. Tan, Chem. Commun., 2010, 46, 1503–1505 RSC.
- C. Zhao, Y. Zhou, Q. Lin, L. Zhu, P. Feng, Y. Zhang and J. Cao, J. Phys. Chem. B, 2011, 115, 642–647 CrossRef CAS PubMed.
- R. Zhang, Z. Ye, Y. Yin, G. Wang, D. Jin, J. Yuan and J. A. Piper, Bioconjugate Chem., 2012, 23, 725–733 CrossRef CAS PubMed.
- D. Kand, P. K. Mishra, T. Saha, M. Lahiri and P. Talukdar, Analyst, 2012, 137, 3921–3924 RSC.
- J. Li, C. F. Zhang, S. H. Yang, W. C. Yang and G. F. Yang, Anal. Chem., 2014, 86, 3037–3042 CrossRef CAS PubMed.
- D. Kand, P. S. Mandal, T. Saha and P. Talukdar, RSC Adv., 2014, 4, 59579–59586 RSC.
- Y. Yue, F. Huo, Y. Zhang, J. Chao, R. Martinez-Manez and C. Yin, Anal. Chem., 2016, 88, 10499–10503 CrossRef CAS PubMed.
- X. Xie, M. Li, F. Tang, Y. Li, L. Zhang, X. Jiao, X. Wang and B. Tang, Anal. Chem., 2017, 89, 3015–3020 CrossRef CAS PubMed.
- H. Shang, H. Chen, Y. Tang, Y. Ma and W. Lin, Biosens. Bioelectron., 2017, 95, 81–86 CrossRef CAS PubMed.
- D. Yu, F. Huang, S. Ding and G. Feng, Anal. Chem., 2014, 86, 8835–8841 CrossRef CAS PubMed.
- X. Shao, R. Kang, Y. Zhang, Z. Huang, F. Peng, J. Zhang, Y. Wang, F. Pan, W. Zhang and W. Zhao, Anal. Chem., 2015, 87, 399–405 CrossRef CAS PubMed.
- D. Yu, Q. Zhai, S. Yang and G. Feng, Anal. Methods, 2015, 7, 7534–7539 RSC.
- X. L. Liu, X. Y. Duan, X. J. Du and Q. H. Song, Chem.– Asian J., 2012, 7, 2696–2702 CrossRef CAS PubMed.
- L. Lin, X. Zeng, Y. Shen, H. Zhu and Y. Qian, Talanta, 2017, 165, 321–325 CrossRef CAS PubMed.
- M. Yuan, X. Ma, T. Jiang, C. Zhang, H. Chen, Y. Gao, X. Yang, L. Du and M. Li, Org. Biomol. Chem., 2016, 14, 10267–10274 CAS.
- Q. Zhai, S. Yang, Y. Fang, H. Zhang and G. Feng, RSC Adv., 2015, 5, 94216–94221 RSC.
- W. Jiang, Y. Cao, Y. Liu and W. Wang, Chem. Commun., 2010, 46, 1944–1946 RSC.
- X. Liu, L. Yang, L. Gao, W. Chen, F. Qi and X. Song, Tetrahedron, 2015, 71, 8285–8289 CrossRef CAS.
- E. W. Miller, A. E. Albers, A. Pralle, E. Y. Isacoff and C. J. Chang, J. Am. Chem. Soc., 2005, 127, 16652–16659 CrossRef CAS PubMed.
- X. Q. Zhan, B. Y. Su, H. Zheng and J. H. Yan, Anal. Chim. Acta, 2010, 658, 175–179 CrossRef CAS PubMed.
- Y. Wen, K. Liu, H. Yang, Y. Liu, L. Chen, Z. Liu, C. Huang and T. Yi, Anal. Chem., 2015, 87, 10579–10584 CrossRef CAS PubMed.
- F. M. Raymo and S. Giordani, J. Am. Chem. Soc., 2001, 123, 4651–4652 CrossRef CAS PubMed.
- G. Copley, J. G. Gillmore, J. Crisman, G. Kodis, C. L. Gray, B. R. Cherry, B. D. Sherman, P. A. Liddell, M. M. Paquette, L. Kelbauskas, N. L. Frank, A. L. Moore, T. A. Moore and D. Gust, J. Am. Chem. Soc., 2014, 136, 11994–12003 CrossRef CAS PubMed.
- G. Szaloki and L. Sanguinet, J. Org. Chem., 2015, 80, 3949–3956 CrossRef CAS PubMed.
- W. Sun, S. Guo, C. Hu, J. Fan and X. Peng, Chem. Rev., 2016, 116, 7768–7817 CrossRef CAS PubMed.
- W. J. Zhang, F. J. Huo, T. Liu, Y. Wen and C. X. Yin, Dyes Pigm., 2016, 133, 248–254 CrossRef CAS.
- Y. Liu and G. Feng, Org. Biomol. Chem., 2014, 12, 438–445 CAS.
- X. Cao, W. Lin, K. Zheng and L. He, Chem. Commun., 2012, 48, 10529–10531 RSC.
- J. Wang, W. Lin and W. Li, Biomaterials, 2013, 34, 7429–7436 CrossRef CAS PubMed.
- L. Sheng, M. Li, S. Zhu, H. Li, G. Xi, Y. G. Li, Y. Wang, Q. Li, S. Liang, K. Zhong and S. X. Zhang, Nat. Commun., 2014, 5, 3044 Search PubMed.
Footnote |
† Electronic supplementary information (ESI) available: Calculation of the detection limit, absorption and fluorescence spectra, 1H NMR, 13C NMR, and HRMS. See DOI: 10.1039/c7ra08707a |
|
This journal is © The Royal Society of Chemistry 2017 |
Click here to see how this site uses Cookies. View our privacy policy here.