DOI:
10.1039/C7RA08818C
(Paper)
RSC Adv., 2017,
7, 46208-46214
Integrated production of furfural and levulinic acid from corncob in a one-pot batch reaction incorporating distillation using step temperature profiling†
Received
9th August 2017
, Accepted 25th September 2017
First published on 28th September 2017
Abstract
Furfural and levulinic acid (LA) are potential major renewable platform chemicals for producing biochemicals and biofuels. In this study, integrated conversion of corncob to furfural and LA was carried out in a one-pot system using p-toluenesulfonic acid (p-TsOH) and step temperature profiling. A batch reaction incorporating distillation (BRD) resulted in a good furfural production yield of 61.6% based on the corncob xylan content for the hydrolysis conditions tested. Distillation not only reduced furfural loss due to side reactions to humins, but also resulted in a fairly pure and concentrated furfural solution. At the end of the first distillation step, the reaction temperature was increased to convert hexoses into LA with a maximal production yield of 61.3 ± 7.8% based on corncob glucan content. LA was extracted with good yield as LA–ester using low-cost alcohols and then phase separated to recycle alcohols and p-TsOH. Good recyclability and reusability of p-TsOH and alcohols were demonstrated.
Introduction
Lignocellulosic biomass as a renewable feedstock has received considerable attention for sustainable production of fuels and chemicals.1 Furfural and levulinic acid (LA) are two attractive sugar-based building blocks that can be obtained from lignocellulosic biomass. These two molecules have versatile pathways for producing a variety of value-added chemicals and biofuels.2–5 For example, they can be used to produce relatively high energy density and high octane number drop-in biofuels such as gasoline, diesel or jet fuel.6–9 High-yield production of furfural and LA from lignocelluloses and efficient separation of these two chemicals are the keys to achieve commercial success.
Several methods for improving furfural and LA yield from pentosan-containing materials have been developed in recent years. Conventional batch production of furfural is always accompanied by the side degradation reactions such as condensation with itself and intermediates (glucose, xylose, 5-hydroxyl methylfurfural (HMF)) to form black, resinous products called humins,10 and therefore is not ideal for large scale applications. Various approaches were applied to address this problem. For example, a biphasic system was employed to reduce humins formation by transferring furfural into the organic phase.10 Using a large amount of organic solution is of course not suitable for commercial furfural production. Two-stage processes were also employed.10–12 These processes hydrolyze pentosans to monomeric pentoses, with subsequent acid-catalyzed dehydration into furfural. The additional stage will inevitably increase furfural production cost. The second stage can be eliminated when using batch production together with immediate separation of furfural after formation. Furfural yield in excess of 85% theoretical was achieved by condensing the vapor fraction from the reactor.13 This approach not only improved furfural yield by protecting furfural from degradation, but also facilitated furfural recovery by avoiding costly distillation from a dilute solution to obtain a highly concentrated furfural condensate.
Many researchers have used one-pot catalytic conversion of cellulose and lignocellulosic biomass to LA.14–17 Catalysts can improve LA yield from lignocellulosic biomass. However, the one-pot processes cannot avoid parasitic pathway reactions from furfural, glucose, and HMF to humins,18 in addition to the inefficient utilization of hemicelluloses. Two-stage acid-catalyzed conversion of lignocelluloses into LA was developed to address this problem.1,19 Pentose was extracted in the first stage prior to LA production, which may improve LA yield. However, the additional stage increases the cost of LA production. Another study reported the production of furfural along with HMF in the first stage using a plug-flow reactor followed by continued degradation of glucose and intermediates to produce LA in the second stage using a stirred-tank reactor.20,21 This process used two reactors, which increases the capital cost.
Separation of LA through distillation is difficult due to its high boiling point of 245 °C, which hinders the commercialization of LA production from lignocelluloses. Gurbuz et al. developed a biphasic reactor with alkylphenol solvents to selectively partition LA from acidic aqueous solutions.22 Li et al. reported that high yields of LA ester can be obtained directly from aqueous hydrolysis mixtures by using toluene and water as co-solvents with the addition of isopropanol.23 The high cost and toxicity of these solvents were challenges for commercial applications.
Here we report integrated production of furfural and LA through acid catalytic conversion of corncob in a one-pot system using a two-step temperature profile as shown in Fig. 1. Furfural was separated with high concentration and purity through steam stripping during the low temperature step. LA was produced during the high temperature step and separated as LA–ester from acidic aqueous solutions by a novel extraction method, using low-cost and non-toxic solvents, which has not been reported in literature. The solvents and acid solution can be reused. Therefore, this study has practical significance to conversion of lignocellulosic biomass to platform chemicals and biofuels.
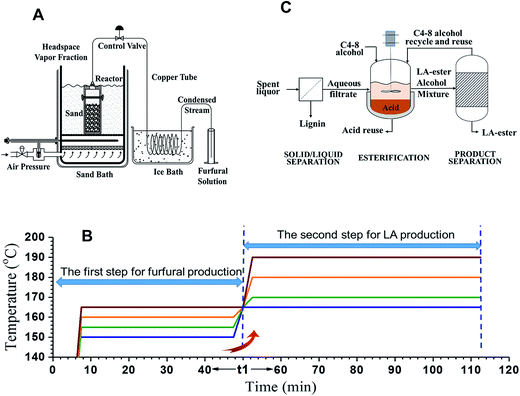 |
| Fig. 1 Schematic diagrams showing the productions of furfural and LA using two step temperature profile and separations. (A) Dehydration experimental apparatus; (B) two-step temperature profiles; (C) LA separation process flow schematics. | |
Results and discussion
Effect of furfural on LA production from a glucose solution
As described above, the effects of furfural and HMF on LA production were first evaluated using a glucose solution spiked with furfural and HMF in batch reactions. Glucose degradation was enhanced with the application of furfural (Fig. 2A). The terminal glucose concentration was 5.75 g L−1 with the application of furfural compared with 6.80 g L−1 without furfural at 60 min. Formic acid (FA) formation was reduced with the application of furfural (Fig. 2A). Furfural degradation was substantial as shown in Fig. 2B. HMF concentration in the liquor increased initially due to formation from glucose and then decreased with reaction time as a result of degradation to LA (Fig. 2C). The application of furfural reduced HMF concentration. The maximal HMF concentration was 0.58 g L−1 and occurred at 20 min into the reaction without the application of furfural compared with 0.53 g L−1 with furfural. This decrease can be attributed to the side reaction between furfural and intermediates.24,25 The application of furfural reduced terminal LA production from 8.76 to 7.27 g L−1 at 120 min. Simultaneous production of furfural and LA was accompanied by parasitic pathway reactions between furfural and either glucose, furfural or HMF, forming black resinous polymeric products, or humins.18,26 The formation of humins was due to degradation of furfural and HMF through aldol addition followed by condensation or polymerization with itself or other reaction intermediates.24,25 Therefore, separating furfural from the process stream, such as using distillation in this study, can improve the LA production.
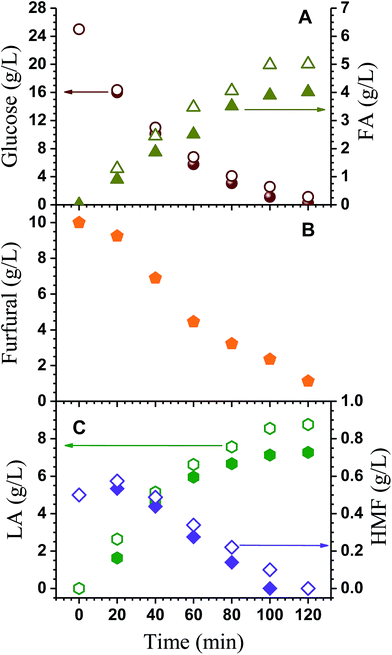 |
| Fig. 2 Effect of furfural and HMF on time-dependent LA production from a glucose solution of 25 g L−1 spiked with furfural of 10 g L−1 and HMF of 0.5 g L−1 using p-TsOH of 1.1 mol L−1 at 150 °C. Solid symbols: with furfural and HMF; open symbols: without furfural and HMF as control. | |
Batch reaction temperature on furfural and LA yield from corncob
To study the effect of reaction temperature on furfural and LA production, batch experiments without steam stripping were conducted in a temperature range between 110 and 190 °C. Furfural production was improved when the reaction temperature was increased from 100 °C to 130 °C and decreased with further increases in temperature as shown in Fig. 3. A similar trend was also observed for LA but with peak yield at 180 °C. HMF is the main conversion intermediate from glucose to LA and can be rapidly converted to LA using acid. This can be seen from the low HMF concentration in the reaction mixture (Fig. 3). The results shown in Fig. 3 clearly indicate that furfural and LA yields are highly dependent on reaction temperature. The fact that maximal furfural yield was obtained at a relatively lower T of 130 °C while the maximal yield of LA was achieved at a higher T of 180 °C, suggests a two-step temperature profile scheme can be employed to achieve production of furfural and LA in a one-pot system, as will be discussed below.
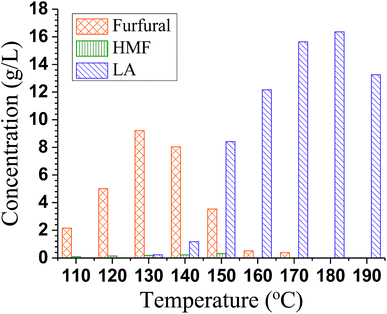 |
| Fig. 3 Effect of reaction temperature on yield of furfural and LA in batch experiments with reaction time of 70 min and catalyst p-TsOH concentration of = 1.1 mol L−1. | |
Furfural and LA production from corncob via steam stripping experiments
In a previous study,27 we reported the optimization of furfural production from corncob through steam stripping experiments using a reaction kinetics based severity, namely the combined hydrolysis factor (CHF).28 Optimal furfural yield can be achieved in a CHF range of 830–1850. Here we extended the previous study to include efficient hexose conversion to LA for a corncob biorefinery operation. A CHF of 2200 was chosen in designing the first-step furfural production in the present study, which is higher than the upper range of CHF of 1850 for optimal furfural production. This is in consideration of our previous study27 that indicated a higher severity can increase HMF production for maximizing LA yield. The elevated CHF did reduce furfural yields approximately 10% compared with the optimal 70% theoretical furfural yields reported previously at a CHF of approximately 1600 using the same p-TsOH concentration and similar temperatures but with longer reaction times. In the present study, four schemes for first-step furfural production were used as listed in Table 1 followed by the variation of reaction temperature in the second-step to maximize LA yield.
Table 1 Furfural yield at similar CHF of approximately 2220
Scheme |
p-TsOH (mol L−1) |
T1 (°C) |
Time (t1) (min) |
CHF (min mol L−1) |
Residual xylose (%) |
Glucose yield (%) |
Furfural concentration (g L−1) |
Furfural production yield (%) |
A |
0.28 |
165 |
60 |
2287 |
2.52 |
39.67 |
20.45 |
55.06 |
B |
0.57 |
160 |
40 |
2189 |
3.19 |
36.31 |
22.15 |
59.63 |
C |
0.87 |
155 |
40 |
2337 |
1.70 |
38.73 |
19.74 |
53.13 |
D |
1.18 |
150 |
40 |
2198 |
0.26 |
36.06 |
22.88 |
61.60 |
In Scheme A, glucose yield from cellulose reached 39.7% theoretical yield at the end of step 1 (or t1 = 60 min) as shown Fig. 4A. The reaction was continued in step 2 where T2 was ramped to a constant value ranging between 165 and 190 °C. A total of four different runs were carried out. T2 had a significant effect on LA production. The LA yield improved with increasing T2 and time in the range studied. Peak LA yield was occurred at T2 = 190 °C in approximately 120 min (or t2 = 60 min) into the reaction. This suggests a high T2 of 190 °C may be necessary to overcome the activation energy of the reaction29 to maximize LA production. Similarly, using Scheme B for step 1, peak LA yield was achieved at T2 = 190 °C in t2 = 60 min in step 2, as shown Fig. 4B. A higher LA peak yield of 64.6% was achieved compared to 61.0% using Scheme A. An even higher LA peak yield of 67.2% was achieved using Scheme C at T2 = 180 °C for t2 = 40 min. The highest LA peak yield of 71.8% was achieved using Scheme D at T2 = 170 °C for t2 = 40 min among all four schemes evaluated for step 1.
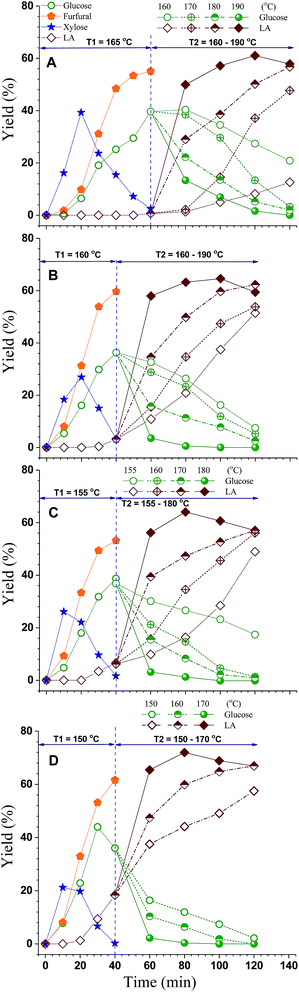 |
| Fig. 4 Effect of step 2 temperature on LA production under four step 1 furfural production conditions of similar CHF approximately 2200 as listed in Table 1. | |
Several observations can be made from the results shown in Fig. 4A–D: (1) for all the schemes for step 1, the production yield of furfural almost reached a maximum value; (2) the maximal LA yield from each scheme was the peak yield obtained at the highest T2 for step 2; (3) the maximal LA yield of 71.8%, from all the 16 runs and among all schemes, was achieved at the highest T2 (170 °C) under Scheme D that had the highest p-TsOH catalyst concentration. These observations indicate a high T2 may be necessary to overcome activation energy for the reaction to form LA. A longer reaction time may also help to improve LA yield. The activation energy for the conversion of intermediates, such as glucose and furan to humins, is reported to be the highest among all the kinetics of conversion;18,30–32 LA degradation to humins, therefore, becomes important only after LA reached maximum. Future studies using higher temperature T2 or longer t2 are needed to verify this argument. It appears that a high catalyst concentration and low temperature such as Scheme D may be beneficial to improve LA yield. A lower severity, i.e., CHF < 1600, in step 1 can be used to improve furfural yield as furfural yield was decreased at higher CHF as listed in Table 1.
Table 2 shows the comparison of furfural and LA yield between the conventional dehydration process carried out in two steps without steam stripping in a batch reactor (BR) and the two-step process in batch reactor with distillation (BRD) under the exact same reaction conditions, i.e., at p-TsOH 1.18 mol L−1 and 150 °C for 40 min for the step 1 and at 170 °C for 40 min for the step 2 (Scheme D at T2 = 170 °C). LA yield using BRD was 61.3 ± 7.8%, greater than that using BR of 52.4%. Distillation in BRD substantially improved furfural yield to 67.1 ± 4.3% from 2.6% using BR without distillation. Furthermore, BRD recovered most furfural through distillation, i.e., distillation efficiency of 74.2% (Table 3, fresh run), or a total recovered furfural yield of approximately 50% without optimization. Batch distillation also resulted in a relatively pure furfural as well as pure LA solution as shown from chromatographic analysis (Fig. S1 and S2,† respectively).
Table 2 Comparisons of furfural and LA production yields between BR and BRD. Reaction condition: p-TsOH = 1.18 mol L−1 at 150 °C for 40 min in the first step, and at 170 °C for 40 min in the second step
|
Furfural (%) |
LA (%) |
BR |
2.6 |
52.4 |
BRD |
67.1 ± 4.3 |
61.3 ± 7.8 |
Table 3 Comparisons of recovery yields of furfural and LA using isobutanol, 1-pentanol, 1-octanol extraction and evaluation of the reusability and efficacy of p-TsOH and the three alcohols for furfural and LA production and recovery
Run no. |
Furfural production yield (%) |
Furfural distillation efficiency (%) |
LA production yield (%) |
LA extraction efficiency (%) |
1-Pentanol (C5H10OH) |
Fresh |
67.1 ± 4.3 |
74.2 ± 1.3 |
61.3 ± 7.8 |
77.8 ± 4.2 |
1 |
63.4 |
74.8 |
43.4 |
67.8 |
2 |
48.1 |
67.4 |
38.4 |
62.6 |
3 |
45.2 |
60.4 |
43.3 |
56.2 |
![[thin space (1/6-em)]](https://www.rsc.org/images/entities/char_2009.gif) |
Isobutanol (C4H8OH) |
Fresh |
67.1 ± 4.3 |
74.2 ± 1.3 |
61.3 ± 7.8 |
78.2 |
![[thin space (1/6-em)]](https://www.rsc.org/images/entities/char_2009.gif) |
1-Octanol (C8H16OH) |
Fresh |
67.1 ± 4.3 |
74.2 ± 1.3 |
61.3 ± 7.8 |
75.5 ± 1.9 |
1 |
64.0 |
77.3 |
47.2 |
66.1 |
2 |
66.1 |
77.0 |
46.6 |
64.0 |
3 |
62.1 |
78.2 |
52.2 |
50.0 |
Extraction of LA and reusability of p-TsOH
LA extraction efficiency from the spent liquor, obtained from Scheme D at T2 = 170 °C, were compared using different alcohols such as isobutanol, 1-pentanol, 1-octanol. The benefits of LA extraction using these alcohols are high extraction efficiency and the recyclability of acid and alcohols. As described in Fig. 1C, the spent liquor was mixed with an alcohol. The LA in the liquor was then transferred to an alcohol phase as a soluble LA–ester (upper tank). The results in Table 3 (labelled as fresh) indicated that isobutanol produced the highest LA extraction efficiency of 78.2% (based on the amount of LA produced), followed by 1-pentanol at 77.8 ± 4.2%, while 1-octanol resulted in the lowest extraction efficiency of 75.5 ± 1.9%.
The water soluble p-TsOH was phase separated from the alcohol–ester solution and subsequently reused for three times to evaluate its efficacy for LA production. The results using 1-pentanol and 1-octanol as extraction solvents are listed in Table 3. Efficiencies of furfural distillation (60.4%) and LA extraction (56.2%) decreased substantially for the third reuse of p-TsOH and pentanol–ester solution, perhaps due to the slight solubility of 1-pentanol in water. Using 1-octanol, a water-insoluble solvent, eliminated the problem associated with low furfural recovery. Distillation efficiency of furfural was maintained near 80%, while both LA production yield and LA extraction efficiency remained lower in the recycling runs than those from using fresh chemicals. Higher concentration of p-TsOH contributed to higher LA production yield with same reaction severity (Fig. 4D) as discussed early. The slight loss of p-TsOH may have contributed into LA production loss. Saturation in alcohol extraction of LA may resulted in efficiency loss in extraction.
The advantages of the present process is that esterification and separation of the ester from the aqueous reaction mixture are combined in a single reactive extraction step without the need for additional solvents. The water-immiscible alcohol that acts as an esterifying agent also serves as the solvent for the extraction of LA ester. Since most of the furfural has been distilled off in the step 1, the formation of undesired, tar-like furfural by-products is minimized in the acid reaction mixture. Therefore, the aqueous mixture does not need to be treated before recycling. Furthermore, the reactive extraction strategy can be carried out directly for the aqueous reaction mixture from acid hydrolysis of biomass without the need to remove the acid catalyst. The same acid catalyst used in acid hydrolysis for the production of LA can be used for esterification between LA and C4–C8 alcohol in the reactive extraction process. The aqueous phase in bottom of the tank (Fig. 2C) can be recycled for reuse.
Conclusions
Integrated conversion of corncob to furfural and LA in a one-pot system was developed using a batch reactor with distillation and a two-step temperature profile. The vapor fraction from the reactor was condensed to produce furfural with a production yield of 61.6% of the theoretical yield based on total xylan in the system at the start of the reaction. The distillation of furfural also substantially improved LA production yield as compared to processing without distillation under the same conditions. The maximum LA yield (61.3 ± 7.8%) was obtained. Furthermore, phase separation of LA using organic solvent was effective in terms of production yield and recyclability.
Materials and methods
Materials
Corncob used in this study was kindly provided by Dr Xuejun Pan of University of Wisconsin-Madison. Corncob was Wiley-milled into particles of 20–40 mesh (model no. 2, Arthur Thomas Co, Philadelphia, PA, USA). p-Toluenesulfonic acid (p-TsOH), LA, HMF and calcium carbonate were ACS reagent grade and purchased from Sigma-Aldrich (St. Louis, MO, USA).
Batch experiments using glucose solutions
To study the effects of HMF and furfural on LA production, 50 mL aqueous glucose solution at 25 g L−1 spiked with HMF at 0.5 g L−1 and furfural at 10 g L−1 was dehydrated at 150 °C in a 100 mL stainless steel reactor using p-TsOH as catalyst at 1.1 mol L−1.
Batch hydrolysis and dehydration of corncob
Ground corncob of 6 g in oven dry (OD) weight was mixed with 50 mL p-TsOH solution of 1.1 mol L−1 in a 100 mL stainless steel reactor. Batch reactions, without steam stripping of furfural, were conducted in a temperature range 110–190 °C with a 10 °C increment to investigate the effect of reaction temperature on furfural and LA production from corncob.
One-pot furfural and LA production and separation
Reaction experiments with steam stripping of furfural were conducted in a laboratory apparatus designed to simulate conditions in a batch reactive distillation process as described previously.27 A 100 mL stainless steel reactor was fitted with a valve that can be opened to continuously remove a portion of vapor from the reactor headspace as schematically shown in Fig. 1A. Copper tubing was immersed in an ice bath to condense the vapor extracted from the reactor headspace. The reactor was filled with 80 mL of p-TsOH solution at a given concentration (0.28, 0.57, 0.87, 1.18 mol L−1) and 6 g in oven dry (OD) weight ground corncob. The reactor was heated to a specified temperature using a sand bath (Techne F932D, Techne Inc., USA). The reactions in the first step for furfural production were performed at 150 °C for t1 = 60 min, or at 155, 160 and 165 °C for t1 = 40 min (Fig. 1B). The vapor extraction valve was slightly open after the reactor reached the desired temperature. Heat was continuously supplied to the reactor to offset the cooling effect due to vapor loss to maintain a nearly constant temperature throughout the reaction. For each experiment, a total of 30 mL of furfural solution was extracted from the reactor into a beaker resulted in a concentrated p-TsOH solution of approximately 0.45, 0.93, 1.44, 1.98 mol L−1 for step 1 Scheme A to D, respectively. When the reaction time reached the preset 40 or 60 min, temperature was raised to 150, 155, 160, 170, 180, or 190 °C in less than 5 min and maintained for t2 = 60 min in the second step reaction for LA production (Fig. 1B). At the end of each reaction, the reactor was cooled down using tap water before opening. Water insoluble solids (WIS) and freely drainable spent liquor were separated with a Büchner funnel using filter paper (15 cm, slow, Fisher Scientific Inc., Pittsburgh, PA, USA). The collected spent liquor and distilled furfural solution were analyzed by HPLC.
An organic solvent (either C4, C5, or C8 alcohol), having the same volume as the collected spent liquor from filtration, was added into the spent liquor. The mixture was then heated to 90 °C for 3 h to form LA–esters which is soluble in the alcohol used. Hence, LA was then separated as LA–ester from the aqueous p-TsOH solution using a phase separation funnel. The remaining acid solution was reused again. The C4-8 alcohol can then be separated using a rotary evaporator or CO2 extraction.
Analytical methods
All solid samples were hydrolyzed using sulfuric acid in two steps for carbohydrate analyses by the Analytical Chemistry and Microscopy Lab (ACML) at the Forest Products Lab as described previously.33 Monosaccharides, LA, furfural, HMF, acetic acid, formic acid in the pretreatment spent liquors, and distilled furfural solutions were determined using a Dionex HPLC system (Ultimate 3000, Thermo Scientific) equipped with an RI (RI-101) detector and a UV detector (VWD-3400RS), as described previously.34
Determining furfural and LA production yields and recovery efficiencies
Furfural production yields were determined from the distillate at the end of each step 1 reaction and from the spent liquor at the end of the step 2 reaction. The steam stripping efficiency of furfural was the percentage of furfural distillated as the total amount of furfural measured for the run. LA production yields were calculated using the LA measured from the spent liquor at the end of each step 2 reaction. LA extraction efficiency was define as the ratio of reduced and total LA in spent liquor. The reported yields of furfural and LA were all in percent of theoretical based on xylan and glucan content in the corncob.
Conflicts of interest
The authors have no conflict of interest.
Acknowledgements
This work was partially supported by the Chinese Scholarship Council (CSC). The funding from the programs made the visiting appointment of Ji at the USDA Forest Products Laboratory (FPL) possible. We would like to acknowledge Fred Matt of US Forest Service Forest Products Laboratory for conducting carbohydrate analyses.
References
- T. Runge and C. H. Zhang, Ind. Eng. Chem. Res., 2012, 51, 3265–3270 CrossRef CAS.
- R. Weingarten, W. C. Conner and G. W. Huber, Energy Environ. Sci., 2012, 5, 7559 CAS.
- J. J. Bozell, L. Moens, D. C. Elliott, Y. Wang, G. G. Neuenscwander, S. W. Fitzpatrick, R. J. Bilski and J. L. Jarnefeld, Resour., Conserv. Recycl., 2000, 28, 227–239 CrossRef.
- R. Mariscal, P. Maireles-Torres, M. Ojeda, I. Sádaba and M. López Granados, Energy Environ. Sci., 2016, 9, 1144–1189 CAS.
- K. Yan, Y. Yang, J. Chai and Y. Lu, Appl. Catal., B, 2015, 179, 292–304 CrossRef CAS.
- A. Bohre, S. Dutta, B. Saha and M. M. Abu-Omar, ACS Sustainable Chem. Eng., 2015, 3, 1263–1277 CrossRef CAS.
- G. W. Huber, S. Iborra and A. Corma, Chem. Rev., 2006, 106, 4044–4098 CrossRef CAS PubMed.
- J. P. Lange, E. van der Heide, J. van Buijtenen and R. Price, ChemSusChem, 2012, 5, 150–166 CrossRef CAS PubMed.
- K. Yan and A. Chen, Fuel, 2014, 115, 101–108 CrossRef CAS.
- R. Weingarten, J. Cho, W. C. Conner and G. W. Huber, Green Chem., 2010, 12, 1423–1429 RSC.
- A. J. Deng, J. L. Ren, H. L. Li, F. Peng and R. C. Sun, RSC Adv., 2015, 5, 60264–60272 RSC.
- F. Lopez, M. T. Garcia, M. J. Feria, J. C. Garcia, C. M. de Diego, M. A. M. Zamudio and M. J. Diaz, Chem. Eng. J., 2014, 240, 195–201 CrossRef CAS.
- A. Mandalika and T. Runge, Green Chem., 2012, 14, 3175–3184 RSC.
- N. Ya'aini, N. A. S. Amin and M. Asmadi, Bioresour. Technol., 2012, 116, 58–65 CrossRef PubMed.
- N. A. S. Ramli and N. A. S. Amin, Energy Convers. Manage., 2015, 95, 10–19 CrossRef CAS.
- G. T. Jeong, Ind. Crops Prod., 2014, 62, 77–83 CrossRef CAS.
- M. Kang, S. W. Kim, J. W. Kim, T. H. Kim and J. S. Kim, Renew. Energ., 2013, 54, 173–179 CrossRef CAS.
- K. Dussan, B. Girisuta, D. Haverty, J. J. Leahy and M. H. Hayes, Bioresour. Technol., 2013, 149, 216–224 CrossRef CAS PubMed.
- Z. G. Yang, H. Y. Kang, Y. F. Guo, G. Q. Zhuang, Z. H. Bai, H. X. Zhang, C. X. Feng and Y. P. Dong, Ind. Crops Prod., 2013, 46, 205–209 CrossRef CAS.
- S. F. D. J. Hayes, M. H. B. Hayes and J. R. H. Ross, in Biorefineries-Industry Processes and Products, Wiley-VCH, Verlag GmbH, 2005, pp. 139–164 Search PubMed.
- W. F. Stephen, in Feedstocks for the Future, American Chemical Society, 2006, pp. 271–287 Search PubMed.
- E. I. Gurbuz, S. G. Wettstein and J. A. Dumesic, ChemSusChem, 2012, 5, 383–387 CrossRef PubMed.
- C. R. Li, D. Q. Ding, Q. N. Xia, X. H. Liu and Y. Q. Wang, ChemSusChem, 2016, 9, 1712–1718 CrossRef CAS PubMed.
- J. Lewkowski, ARKIVOC, 2001, 17–54 Search PubMed.
- S. K. R. Patil and C. R. F. Lund, Energy Fuels, 2011, 25, 4745–4755 CrossRef CAS.
- R. Weingarten, W. C. Conner and G. W. Huber, Energy Environ. Sci., 2012, 5, 7559–7574 CAS.
- H. Ji, L. Chen, J. Y. Zhu, R. Gleisner and X. Zhang, Ind. Eng. Chem. Res., 2016, 55, 11253–11259 CrossRef CAS.
- W. Zhu, C. J. Houtman, J. Y. Zhu, R. Gleisner and K. F. Chen, Process Biochem., 2012, 47, 785–791 CrossRef CAS.
- Y. Chen, G. Li, F. Yang and S. M. Zhang, Polym. Degrad. Stab., 2011, 96, 863–869 CrossRef CAS.
- C. Chang, X. J. Ma and P. L. Cen, Chin. J. Chem. Eng., 2009, 17, 835–839 CrossRef CAS.
- B. Girisuta, K. Dussan, D. Haverty, J. J. Leahy and M. H. B. Hayes, Chem. Eng. J., 2013, 217, 61–70 CrossRef CAS.
- B. Girisuta, L. P. B. M. Janssen and H. J. Heeres, Ind. Eng. Chem. Res., 2007, 46, 1696–1708 CrossRef CAS.
- X. Luo, R. Gleisner, S. Tian, J. Negron, W. Zhu, E. Horn, X. J. Pan and J. Y. Zhu, Ind. Eng. Chem. Res., 2010, 49, 8258–8266 CrossRef CAS.
- J. Y. Zhu, M. S. Chandra, F. Gu, R. Gleisner, R. Reiner, J. Sessions, G. Marrs, J. Gao and D. Anderson, Bioresour. Technol., 2015, 179, 390–397 CrossRef CAS PubMed.
Footnote |
† Electronic supplementary information (ESI) available. See DOI: 10.1039/c7ra08818c |
|
This journal is © The Royal Society of Chemistry 2017 |
Click here to see how this site uses Cookies. View our privacy policy here.