DOI:
10.1039/C7RA10369G
(Paper)
RSC Adv., 2017,
7, 47219-47224
Isostructural functionalization by –OH and –NH2: different contributions to CO2 adsorption†
Received
18th September 2017
, Accepted 29th September 2017
First published on 6th October 2017
Abstract
Two isostructural mfj-type metal–organic frameworks, [Cu2(ABDPB)(H2O)]n (HHU-3, HHU for Hohai University; H4ABDPB for 5-amino-1,3-bis(3,5-dicarboxylphenyl)-benzene) and [Cu2(OBDPB)(H2O)]n (HHU-4; H4OBDPB for 5-hydroxyl-1,3-bis(3,5-dicarboxylphenyl)-benzene) were successfully synthesized by V-shaped tetracarboxylic ligand with amino and hydroxyl groups, respectively. Compared with the prototypical MOF, PCN-306, both MOFs exhibit obviouse decreases in BET surface area and pore volume, with the values of 2354 m2 g−1 and 0.920 cm3 g−1 for HHU-3, and 2353 m2 g−1 and 0.954 cm3 g−1 for HHU-4. Although both functional groups are believed to be effective in strengthen CO2 interactions with the framework, in this type of MOF, amino group works better due to its basic nature. Meanwhile, the decoration of amino groups grafted HHU-3 high CO2 adsorption capacity (25.6 wt% at 1 bar and 273 K) and high CO2 selectivity (SCO2/N2 = 129).
Introduction
Metal–organic frameworks (MOFs), as promising porous materials, have shown great potential in gas storage and separation due to their large surface area and high porosity.1–11 Compared with traditional porous materials, the feasibility of structural tunability is a great advantage of MOFs. Various functional groups can be intentionally introduced into frameworks via either ligand-design or post-synthetic modification to achieve high performance.12–16 Practically, both of them show their own pros and cons. Sharp decrease in pore volume generally happens in the functionalization reactions of post-synthetic process, and therefore leads to the declines of gas adsorption capacity. Besides, the completeness of functionalization cannot be easily attained.17 Pre-design modification shows its advantage in obtaining the totally functionalized MOF. However, due to the chemical/physical activity of some functional groups, it requires a structural-stable prototypical MOF that can resist these groups from interfering the formation of the desired MOF.18,19
Mfj-type MOF is a good platform for functionalization towards high performance in gas adsorption, as illustrated by PCN-306 and its analogues, in which the introduction of different functional groups by pre-synthetic method seldom led to a structural change.20–22 Based upon this type of MOF, in this contribution, we managed to decorate the channels in PCN-306 by two different polar groups, –NH2 and –OH (Scheme 1), both of which are regarded to be effective in strengthening CO2-framework interactions.23–25 The functional groups were selected on the basis of two points: (a) similar size, which avoids the influence of narrow pore effect induced by different pore sizes; (b) different acid-base property makes the comparison of their effect on gas adsorption more specific. Compared with the prototypical MOF PCN-306, the resulted two mfj-type MOF [Cu2(ABDPB)(H2O)2]n (HHU-3, HHU for Hohai University; H4ABDPB for 5-amino-1,3-bis(3,5-dicarboxylphenyl)-benzene) and [Cu2(OBDPB)(H2O)2]n (HHU-4; H4OBDPB for 5-hydroxyl-1,3-bis(3,5-dicarboxylphenyl)-benzene) exhibit slight decreases in BET surface area, with the values of 2354 m2 g−1 and 2353 m2 g−1 respectively. Although both of them are polar functional groups, which are expected to be effective in CO2 adsorption in MOFs, amine groups obviously work better than hydroxyl groups. The decoration of amino groups grafted HHU-3 high CO2 adsorption capacity (25.6 wt% at 1 bar and 273 K) and high CO2 selectivity (SCO2/N2 = 129).
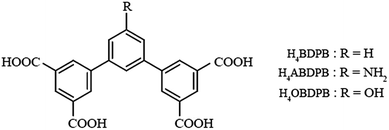 |
| Scheme 1 Ligands-functionalization by hydroxyl and amino groups. | |
Experimental
Materials and methods
All chemical reagents were obtained from commercial sources and, unless otherwise noted, were used as received without further purification. Elemental analyses (C, H, and N) were performed on a Perkin-Elmer 240 analyzer. The IR spectra were recorded in the 400–4000 cm−1 on a Bruker VERTEX 80V spectrometer using KBr pellets. 1H NMR spectra were recorded on a Bruker DRX-500 spectrometer with tetramethylsilane as an internal reference. Thermal gravimetric analyses (TGA) were performed under N2 atmosphere (100 mL min−1) with a heating rate of 5 °C min−1 using a 2960 SDT thermogravimetric analyzer. Powder X-ray diffraction (PXRD) data were collected on a Bruker D8 ADVANCE X-ray diffractometer with Cu/Kα radiation.
Gas sorption measurements
Low-pressure gas sorption measurements were carried out using a Micromeritics ASAP 2020 surface area and pore size analyzer up to saturated pressure at different temperatures. Before the gas sorption measurement, more than 200 mg as-synthesized sample (PCN-306/HHU-3/HHU-4) was washed with DMF and methanol (acetone for PCN-306), respectively. Fresh anhydrous methanol (acetone for PCN-306) was then added, and the sample was allowed to soak for 3 days for solvent-exchange. During this period, methanol (acetone for PCN-306) was refreshed every 8 hours. After then, the sample was charged into a sample tube and activated at certain temperature (120 °C for PCN-306, 80 °C for HHU-3, and 90 °C for HHU-4) for 12 hours under vacuum.
X-ray collection and structure determination
Single crystal suitable for X-ray structure determination were selected and sealed in a capillary under a microscope. The X-ray diffraction intensity data were measured on a BRUKER D8 VENTURE PHOTON diffractometer at room temperature using graphite monochromated Mo/Kα radiation (λ = 0.71073 Å). Data reduction was made with the Bruker Saint program. The structures were solved by direct methods and refined with full-matrix least squares technique using the SHELXTL package. Non-hydrogen atoms were refined with anisotropic displacement parameters during the final cycles. Hydrogen atoms were placed in calculated positions with isotropic displacement parameters set to 1.2 × Ueq of the attached atom. The unit cell includes a large region of disordered solvent molecules, which could not be modeled as discrete atomic sites. We employed PLATON/SQUEEZE to calculate the diffraction contribution of the solvent molecules and, thereby, to produce a set of solvent-free diffraction intensities; structures were then refined again using the data generated. Crystal data and refinement conditions are shown in Table S1.† The crystal data for HHU-3 and HHU-4 have been deposited in CSD database, and labeled as 1566591 and 1566592, respectively.
Synthesis and characterization
Syntheses of organic linker 1,3-bis(3,5-dicarboxylphenyl)-benzene (H4BDPB), 5-amino-1,3-bis(3,5-dicarboxylphenyl)-benzene (H4ABDPB), and 5-hydroxyl-1,3-bis(3,5-dicarboxylphenyl)-benzene (H4OBDPB) (Scheme 1). Details of their synthetic procedure are given in the ESI.†
H4ABDPB. 1H NMR (500 MHz, DMSO-d6, δ ppm): 13.37 (brs, 4H, COO
), 8.47 (s, 2H, Ar
), 8.42 (s, 4H, Ar
), 7.19 (s, 1H, Ar
), 7.04 (s, 2H, Ar
), 5.76 (brs, 2H, N
). Selected FTIR (neat, cm−1): 3399, 2921, 1702, 1599, 1380, 1236, 909, 866, 760, 675, 639.
HHU-3. A mixture of H4ABDPB (5.05 mg, 0.012 mmol) and CuCl2·2H2O (13.6 mg, 0.08 mmol) was dissolved in DMF/H2O (2 mL, 5: 1, v/v) in a screw-capped vial. A concentrated HNO3 (0.03 mL) (65%, aq.) was added to the mixture, the vial was capped and placed in an oven at 65 °C for one day. The resulting dark-green pyramid-shaped crystals were filtered and washed with DMF several times to give HHU-3 materials. Yield: 76%. Elemental analysis: calcd for activated Cu2(ABDPB), (%): C, 48.44; H, 2.02; N, 2.57; found: C, 48.16; H, 2.27; N, 2.83.‡
H4OBDPB. 1H NMR (500 MHz, DMSO-d6, δ ppm): 13.62 (brs, 4H, COO
), 8.51 (s, 2H, Ar
), 8.47 (s, 4H, Ar
), 8.05 (s, 1H, O
), 7.81 (d, 4JH–H = 7.5 Hz, 2H, Ar
), 7.67 (t, 4JH–H = 7.5 Hz, 1H, Ar
). Selected FTIR (neat, cm−1): 3420, 3083, 2525, 1705, 1598, 1444, 1385, 1203, 914, 851, 756, 673, 636.
HHU-4. A mixture of H4OBDPB (5.06 mg, 0.012 mmol) and CuCl2·2H2O (6.8 mg, 0.04 mmol) was dissolved in DMF/H2O (2 mL, 5: 1, v/v) in a screw-capped vial. A concentrated HNO3 (0.05 mL) (65%, aq.) was added to the mixture, the vial was capped and placed in an oven at 65 °C for one day. The resulting blue pyramid-shaped crystals were filtered and washed with DMF several times to give HHU-4 materials. Yield: 64%. Elemental analysis: calcd for activated Cu2(OBDPB), (%): C, 48.35; H, 1.83; N, 0; found: C, 48.01; H, 2.02; N, 0.03.
H4BDPB. 1H NMR (500 MHz, DMSO-d6, δ ppm): 13.56 (brs, 4H, COOH), 8.51 (s, 2H, ArH), 8.47 (s, 4H, ArH), 8.05 (s, 1H, ArH), 7.81 (d, J = 5 Hz, 2H, ArH), 7.68 (d, J = 5 Hz, 1H, ArH). Selected FTIR (neat, cm−1): 3405, 2991, 1700, 1599, 1440, 1234, 905, 755, 675, 633.
PCN-306. A mixture of H4BDPB (4.86 mg, 0.012 mmol) and CuCl2·2H2O (13.6 mg, 0.08 mmol) was dissolved in DMF/H2O (2 mL, 5: 1, v/v) in a screw-capped vial. A concentrated HNO3 (0.05 mL) (65%, aq.) was added to the mixture, the vial was capped and placed in an oven at 65 °C for two days. The resulting blue pyramid-shaped crystals were filtered and washed with DMF several times to give PCN-306 materials. Yield: 53%. Elemental analysis: calcd for activated Cu2(BDPB), (%): C, 49.91; H, 1.70; N, 0.00; found: C, 49.65; H, 1.91; N, 0.05.
Results and discussion
Single-crystal X-ray diffraction analysis reveals that HHU-4 crystallizes in orthorhombic space group Cmcm. The asymmetric unit of HHU-4 consists of three crystallographically unique Cu2+ ions (Cu1, Cu2, and Cu3 with the occupation of 1, 1/4, and 1/4, respectively), one half and a quarter OBDPB-ligand and three coordinated water molecules (Fig. S1†). HHU-3 crystallizes in a different orthorhombic space group Cmc21, which has less symmetric elements than Cmcm. Therefore, the asymmetric unit of HHU-3 consists of more moieties, with four crystallographically unique Cu2+ ions, one and a half ABDPB-ligand and four coordinated water molecules. Although they are in different space groups due to different torsion angles, the structures of HHU-3 and HHU-4 are isostructural to PCN-306, as shown in Fig. 1 by their PXRD patterns and crystal structures. The structure of both HHU-3 and HHU-4 contains two types of ligands with different dihedral angles between central and terminal benzene rings. As illustrated in Fig. 2, the dihedral angles between central and terminal benzene rings in HHU-3 are about 38.0°, 47.7° and 52.8°, while the dihedral angels in HHU-4 are approximately 43.4° and 52.7°. Bridged by these ligands, all the paddlewheel dinuclear Cu clusters [Cu2(COO)4] are assembled into the same (3,3,4,4)-c 4-nodal mfj-type net in both HHU-3 and HHU-4 if paddlewheel Cu clusters are simplified as 4-connected nodes and ligands as two 3-connected nodes (Fig. S2 and S3†). Obviously, due to functionalization, windows along c axis were narrowed from 9 Å to 6 Å while windows along a axis exhibit no obvious change (Fig. 1a and b). By PLATON, the total potential solvent accessible volume of HHU-3 and HHU-4 were calculated to be 70.8% and 69.8%. Therefore, from the perspective of volume occupancy, the introduction of –NH2 and –OH costs almost the same pore volume.
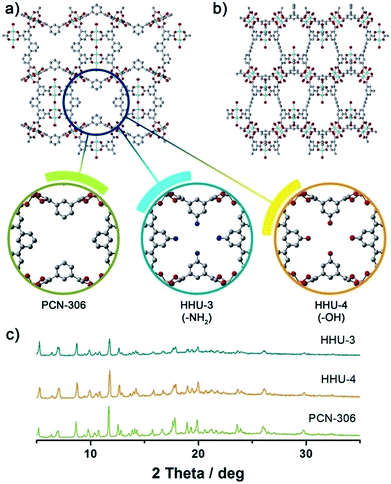 |
| Fig. 1 Functionalizing PCN-306 by –OH and –NH2 group. (a) and (b) are channels along c axis and a axis; (c) is the PXRD patterns of as-synthesized samples of PCN-306, HHU-3, and HHU-4. | |
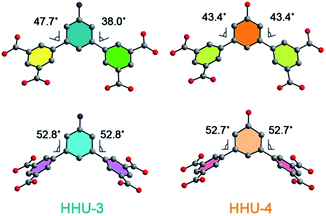 |
| Fig. 2 Two types of ligands in HHU-3 and HHU-4. | |
In order to investigate the effect of functional groups, the permanent porosities of HHU-3 and HHU-4 were determined by N2 adsorption measurements at 77 K. For a clear comparison, we also measured the N2 adsorption of PCN-306. Before measurements, the solvent-exchanged samples were evacuated at certain temperature for 12 hours, with crystallinity remained after solvent removal (Fig. S4–S6†). BET analysis reveals obvious decreases in surface area of functionalized MOFs. As shown in Fig. 3, the N2 uptake amount of PCN-306 at P/P0 = 1 is 690.3 cm3 g−1, which agrees quite well with that reported by Zhou group.20 Functionalization caused a slight decrease of N2 uptake amount in HHU-3 and HHU-4, with the value of 596.0 cm3 g−1 and 618.6 cm3 g−1, respectively. Based on N2 isotherms, the BET surface areas of PCN-306, HHU-3 and HHU-4 were calculated to be 2772 m2 g−1, 2354 m2 g−1, and 2353 m2 g−1, respectively. If monolayer coverage of N2 is assumed, the Langmuir surface areas of PCN-306, HHU-3 and HHU-4 were estimated to be 3003 m2 g−1, 2570 m2 g−1, and 2656 m2 g−1, respectively. As expected, the total pore volume decreases from 1.066 cm3 g−1 in PCN-306 to 0.920 cm3 g−1 in HHU-3, and 0.954 cm3 g−1 in HHU-4, consistent with the size of functional groups.
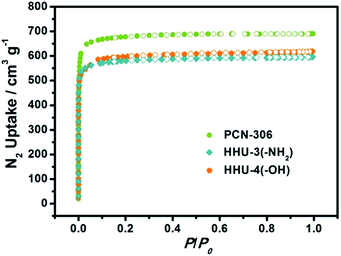 |
| Fig. 3 N2 adsorption isotherms of PCN-306, HHU-3 and HHU-4 at 77 K. | |
Both the crystal structures and N2 adsorption measurements reveal that –NH2 and –OH have similar size, which help us exclude the pore-size effect on gas adsorption caused by functional groups with different sizes. Therefore, we measured the H2 adsorption capacities of both HHU-3 and HHU-4, as well as their prototypical MOF, PCN-306. As shown in Fig. 4a, the H2 adsorption capacities of PCN-306, HHU-3, and HHU-4 at 1 bar and 77 K are 2.61 wt%, 2.46 wt%, and 2.36 wt%, respectively. PCN-306 shows the highest H2 adsorption capacity at 77 K, which is consistent with its large surface area and pore volume. After functionalization, the decline of surface area and pore volume lead to the decrease of H2 adsorption capacity in HHU-3 and HHU-4. Based upon the isotherms measured at 77 K and 87 K, the H2 adsorption enthalpies of PCN-306, HHU-3, and HHU-4 were calculated. As shown in Fig. 4b, the zero-coverage H2 adsorption enthalpies of HHU-3 and HHU-4 are 6.41 kJ mol−1, 6.60 kJ mol−1, which are slightly lower than that of PCN-306 (6.65 kJ mol−1). Therefore, the introduction of polar functional groups do not benefit H2 adsorption in mfj-type MOFs, but causes the decrease of surface area and pore volume which closely correlate with hydrogen uptake capacity.
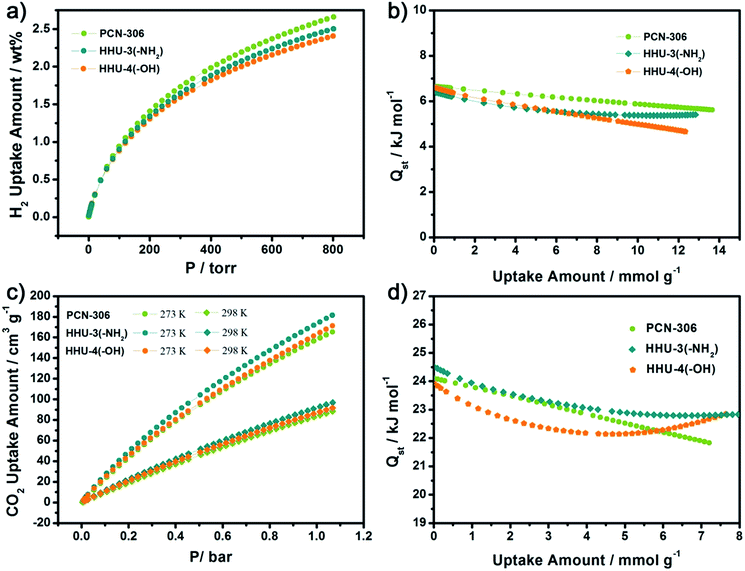 |
| Fig. 4 (a) H2 adsorption isotherms for PCN-306, HHU-3 and HHU-4 at 77 K; (b) H2 adsorption enthalpies of PCN-306, HHU-3 and HHU-4; (c) CO2 adsorption isotherms for PCN-306, HHU-3 and HHU-4 at 273 K and 298 K; (d) CO2 adsorption enthalpies of PCN-306, HHU-3 and HHU-4. | |
Since we deliberately grafted polar functional groups in MOF materials, an improvement in CO2 adsorption was expected. Thus, we measured the CO2 adsorption of each MOF, as shown in Fig. 4c. Compared with the prototype MOF, PCN-306, the CO2 adsorption capacity of the amino-functionalized MOF, HHU-3, exhibits an obvious improvement. At 1 bar and 273 K, the CO2 uptake amount of HHU-3 is 175 cm3 g−1 (25.6 wt%), which is higher than most of its iso-structural analogues (Table 1) and meanwhile much higher than that of some typical MOFs, such as CAU-1 (ref. 26) (161 cm3 g−1), SNU-4 (ref. 27) (20.6 wt%) and MOF-5 (ref. 28) (6.2 wt%). This value is also quit comparable with that of Cu-EBTC29 (25.9 wt%). The hydroxyl-functionalized MOF, HHU-4, however, exhibits a lower adsorption capacity with the value of 164.7 cm3 g−1 (24.4 wt%) at 1 bar. Similar behavior was also observed in CO2 isotherms of HHU-3, PCN-306, and HHU-4 at 298 K (Fig. 4c), with the values of 93.0 cm3 g−1 (15.4 wt%), 84.7 cm3 g−1 (14.3 wt%), and 88.0 cm3 g−1 (14.7 wt%) at 1 bar, respectively. Thus the adsorption enthalpy of CO2 adsorption for each MOF was calculated by the virial method, using experimental isotherm data at 273 K and 298 K. As shown in Fig. 4d, the CO2 adsorption enthalpies at zero-coverage for PCN-306, HHU-3 and HHU-4 were 24.1 kJ mol−1, 24.6 kJ mol−1 and 23.9 kJ mol−1, respectively. Although the improvement is not significant in zero-coverage, the CO2 adsorption enthalpy of HHU-3 is higher than that of PCN-306 at all loading range, while that of HHU-4 is lower than that of PCN-306 at most range. From the perspective of enhancing CO2 adsorption capacity, amino group performs much better than hydroxyl group due to its basic property which is favored by CO2 molecules.
Table 1 Pore characteristics and CO2 adsorption capacities for selected iso-structural analogues
MOFs |
SLangmuir [m2 g−1] |
Vpore [cm3 g−1] |
Qst [kJ mol−1] |
CO2 at 273 K [wt%] |
According to ref. 20. Data collected in this article. |
PCN-306 (–H) |
2929a/3003b |
1.043a/1.066b |
24.0a/24.1b |
22.9a/23.8b |
HHU-3 (–NH2) |
2570 |
0.920 |
24.6 |
25.6 |
PCN-305 (–N) |
2599 |
0.926 |
23.8 |
23.2 |
PCN-307 (–CH3) |
2235 |
0.808 |
22.8 |
23.2 |
HHU-4 (–OH) |
2656 |
0.954 |
23.9 |
24.4 |
PCN-308 (–CF3) |
2234 |
0.810 |
22.2 |
24.8 |
As amino group has positive effect on CO2 adsorption, we further compared the CO2 selectivities of HHU-3 and PCN-306 over N2 via the Ideal Adsorption Solution Theory (IAST). As shown Fig. 5, the CO2 selectivity of PCN-306 towards N2 is 76 at 1 bar in the case of CO2/N2 = 15
:
85 gas mixtures. For HHU-3, obvious improvement in CO2/N2 selectivity was observed, with the value of 129 at 1 bar. Meanwhile, this value is much higher than some well-known MOFs such as Fe-BTT30,31 (CO2/N2: 61), Cu-TDPAT30 (CO2/N2: 88), and quite comparable with en-CuBTTri32 (CO2/N2: 143), SIFSIX-2-Cu-i5 (CO2/N2: 140), and Mg-MOF-74 (ref. 33) (CO2/N2: 148.1), indicating a high CO2 selectivity. The incorporation of amino groups benefits CO2 adsorption, therefore contributes to a higher CO2 selectivity compared to the prototypical MOF.
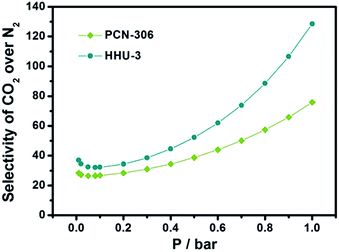 |
| Fig. 5 CO2/N2 selectivity of HHU-3 and PCN-306 at 273 K by IAST. | |
Conclusions
In summary, based upon a structural-stable mfj-type MOF, PCN-306, a pre-synthetic approach of functionalization was applied and its amino- and hydroxyl-functionalized analogues were obtained respectively. Due to comparable size of functional groups, HHU-3 and HHU-4 show similar decreases in both BET surface area and pore volume, which cause decline in their capacities for H2 adsorption. Although both amino and hydroxyl groups are regarded to be effective in strengthen CO2 interactions with the framework, in this type of MOF, amino group works better than hydroxyl group due to its basic nature favoured by CO2 molecules. The high CO2 uptake amount among mfj-type MOFs and high CO2 selectivity make HHU-3 one of potential candidates for CO2 capture.
Conflicts of interest
There are no conflicts to declare.
Acknowledgements
This work was supported by the Natural Science Fund of Jiangsu Province (Grant No. BK20150798, BK20140968, BK20161506 and BK20160857), the National Natural Science Foundation of China (Grant No. 21601047 and 21501094), the Fundamental Research Funds for the Central Universities (Grant No. 2015B12014) and Jiangsu Doctor Program (Grant No. B15065).
Notes and references
- P. M. Bhatt, Y. Belmabkhout, A. Cadiau, K. Adil, O. Shekhah, a. shkurenko, L. J. Barbour and M. Eddaoudi, J. Am. Chem. Soc., 2016, 138, 9301–9307 CrossRef CAS PubMed.
- Y. He, W. Zhou, G. Qian and B. Chen, Chem. Soc. Rev., 2014, 43, 5657–5678 RSC.
- X. Zhao, X. Bu, Q. G. Zhai, H. Tran and P. Feng, J. Am. Chem. Soc., 2015, 137, 1396–1399 CrossRef CAS PubMed.
- T. F. Liu, D. Feng, Y. P. Chen, L. Zou, M. Bosch, S. Yuan, Z. Wei, S. Fordham, K. Wang and H. C. Zhou, J. Am. Chem. Soc., 2015, 137, 413–419 CrossRef CAS PubMed.
- P. Nugent, Y. Belmabkhout, S. D. Burd, A. J. Cairns, R. Luebke, K. Forrest, T. Pham, S. Ma, B. Space, L. Wojtas, M. Eddaoudi and M. J. Zaworotko, Nature, 2013, 495, 80–84 CrossRef CAS PubMed.
- X. Zhao, X. Bu, E. T. Nguyen, Q. G. Zhai, C. Mao and P. Feng, J. Am. Chem. Soc., 2016, 138, 15102–15105 CrossRef CAS PubMed.
- O. Shekhah, Y. Belmabkhout, Z. Chen, V. Guillerm, A. Cairns, K. Adil and M. Eddaoudi, Nat. Commun., 2014, 5, 4228 CAS.
- C. E. Wilmer, O. K. Farha, T. Yildirim, I. Eryazici, V. Krungleviciute, A. A. Sarjeant, R. Q. Snurr and J. T. Hupp, Energy Environ. Sci., 2013, 6, 1158 CAS.
- D. Wang, B. Liu, S. Yao, T. Wang, G. Li, Q. Huo and Y. Liu, Chem. Commun., 2015, 51, 15287–15289 RSC.
- I. Senkovska and S. Kaskel, Chem. Commun., 2014, 50, 7089–7098 RSC.
- Z. Lu, J. Zhang, J. Duan, L. Du and C. Hang, J. Mater. Chem. A, 2017, 5, 17287–17292 CAS.
- A. L. Grzesiak, F. J. Uribe, N. W. Ockwig, O. M. Yaghi and A. J. Matzger, Angew. Chem., Int. Ed., 2006, 45, 2553–2556 CrossRef CAS PubMed.
- H.-M. Wen, G. Chang, B. Li, R.-B. Lin, T.-L. Hu, W. Zhou and B. Chen, Cryst. Growth Des., 2017, 17, 2172–2177 CAS.
- P. V. Dau and S. M. Cohen, Chem. Commun., 2014, 50, 12154–12157 RSC.
- R. Banerjee, H. Furukawa, D. Britt, C. Knobler, M. O'Keeffe and O. M. Yaghi, J. Am. Chem. Soc., 2009, 131, 3875–3877 CrossRef CAS PubMed.
- N. H. Alsmail, M. Suyetin, Y. Yan, R. Cabot, C. P. Krap, J. Lu, T. L. Easun, E. Bichoutskaia, W. Lewis, A. J. Blake and M. Schroder, Chem.–Eur. J., 2014, 20, 7317–7324 CrossRef CAS PubMed.
- X. Kong, H. Deng, F. Yan, J. Kim, J. A. Swisher, B. Smit, O. M. Yaghi and J. A. Reimer, Science, 2013, 341, 882–885 CrossRef CAS PubMed.
- R. K. Deshpande, J. L. Minnaar and S. G. Telfer, Angew. Chem., Int. Ed., 2010, 49, 4598–4602 CrossRef CAS PubMed.
- Z. Wang and S. M. Cohen, Chem. Soc. Rev., 2009, 38, 1315–1329 RSC.
- Y. Liu, J. R. Li, W. M. Verdegaal, T. F. Liu and H. C. Zhou, Chem.–Eur. J., 2013, 19, 5637–5643 CrossRef CAS PubMed.
- Z. Lu, L. Du, K. Tang and J. Bai, Cryst. Growth Des., 2013, 13, 2252–2255 CAS.
- L. Du, Z. Lu, L. Xu and J. Zhang, RSC Adv., 2017, 7, 21268–21272 RSC.
- Y. Zhao, H. Wu, T. J. Emge, Q. Gong, N. Nijem, Y. J. Chabal, L. Kong, D. C. Langreth, H. Liu, H. Zeng and J. Li, Chem.–Eur. J., 2011, 17, 5101–5109 CrossRef CAS PubMed.
- K. Sumida, D. L. Rogow, J. A. Mason, T. M. McDonald, E. D. Bloch, Z. R. Herm, T. H. Bae and J. R. Long, Chem. Rev., 2012, 112, 724–781 CrossRef CAS PubMed.
- Q. Yang, A. D. Wiersum, P. L. Llewellyn, V. Guillerm, C. Serre and G. Maurin, Chem. Commun., 2011, 47, 9603–9605 RSC.
- X. Si, C. Jiao, F. Li, J. Zhang, S. Wang, S. Liu, Z. Li, L. Sun, F. Xu, Z. Gabelica and C. Schick, Energy Environ. Sci., 2011, 4, 4522 CAS.
- Y. G. Lee, H. R. Moon, Y. E. Cheon and M. P. Suh, Angew. Chem., Int. Ed., 2008, 47, 7741–7745 CrossRef CAS PubMed.
- K. S. Walton, A. R. Millward, D. Dubbeldam, H. Frost, J. J. Low, O. M. Yaghi and R. Q. Snurr, J. Am. Chem. Soc., 2008, 130, 406–407 CrossRef CAS PubMed.
- Y. Hu, S. Xiang, W. Zhang, Z. Zhang, L. Wang, J. Bai and B. Chen, Chem. Commun., 2009, 7551–7553 RSC.
- Z. Zhang, Z. Li and J. Li, Langmuir, 2012, 28, 12122–12133 CrossRef CAS PubMed.
- K. Sumida, S. Horike, S. S. Kaye, Z. R. Herm, W. L. Queen, C. M. Brown, F. Grandjean, G. J. Long, A. Dailly and J. R. Long, Chem. Sci., 2010, 1, 184 RSC.
- A. Demessence, D. M. D'Alessandro, M. L. Foo and J. R. Long, J. Am. Chem. Soc., 2009, 131, 8784–8786 CrossRef CAS PubMed.
- J. A. Mason, K. Sumida, Z. R. Herm, R. Krishna and J. R. Long, Energy Environ. Sci., 2011, 4, 3030 CAS.
Footnotes |
† Electronic supplementary information (ESI) available: Experimental details, PXRD patterns, crystallographic data, additional gas adsorption isotherms, heat of adsorption, TG curves. CCDC 1566591 and 1566592. For ESI and crystallographic data in CIF or other electronic format see DOI: 10.1039/c7ra10369g |
‡ Crystal data for HHU-3: C66H33Cu6N3O30·x(solv.), M = 1729.25, orthorhombic, Cmc21, a = 24.600(2) Å, b = 33.412(3) Å, c = 18.478(2) Å, α = β = γ = 90°, V = 15 188(2) Å3, Z = 4, Dc = 0.756 g cm−3, GOF = 0.898 based on F2, final R1 = 0.0604, wR2 = 0.1201 [for 15 966 data I > 2σ(I)]; data for structure was treated with Squeeze. Crystal data for HHU-4: C66H30Cu6O33·x(solv.), M = 1732.20, orthorhombic, Cmcm, a = 24.6748(11) Å, b = 33.5109(15) Å, c = 18.4547(11) Å, α = β = γ = 90°, V = 15 259.7(13) Å3, Z = 4, Dc = 0.754 g cm−3, GOF = 1.021 based on F2, final R1 = 0.0751, wR2 = 0.1828 [for 6843 data I > 2σ(I)]; data for structure was treated with Squeeze. |
|
This journal is © The Royal Society of Chemistry 2017 |
Click here to see how this site uses Cookies. View our privacy policy here.