DOI:
10.1039/C7SC01700F
(Edge Article)
Chem. Sci., 2017,
8, 5622-5627
Photoredox ketone catalysis for the direct C–H imidation and acyloxylation of arenes†
Received
17th April 2017
, Accepted 4th June 2017
First published on 5th June 2017
Abstract
The photoexcited aryl ketone-catalyzed C–H imidation of arenes and heteroarenes is reported. Using 3,6-dimethoxy-9H-thioxanthen-9-one as a catalyst in combination with a bench-stable imidating reagent, C–N bond formation proceeds with high efficiency and a broad substrate scope. A key part of this method is that the thioxanthone catalyst acts as an excited-state reductant, thus establishing an oxidative quenching cycle for radical aromatic substitution. The synthetic potential of this photoexcited ketone catalysis is further demonstrated by application to the direct C–H acyloxylation of arenes.
Introduction
Since its inception, the photochemistry of carbonyl compounds, especially ketones, has been studied extensively, and the electronically excited state of ketones is known to undergo different types of bond scission and reformation depending on the reaction conditions.1 In addition to their own structural reorganizations and transformations, a series of aryl ketones, such as benzophenone and its derivatives, act as effective photosensitizers.2 Upon exposure to light, they are excited to a singlet state, and subsequent rapid transition to a triplet state through intersystem crossing (ISC) proceeds almost quantitatively. Owing to their relatively long lifetimes, aryl ketone triplets have long been appreciated for their capability to facilitate photochemical reactions. However, their actual usage as catalysts in selective organic synthesis has been rather limited (Scheme 1a). In particular, while the ability of photoexcited aryl ketones to mediate triplet energy transfer (EnT)3 and hydrogen atom transfer (HAT)4 has been exploited in several reaction systems, the utility of their photoinduced electron transfer (PET) reactivity in catalysis remains largely underexplored.5 This is rather intriguing as the simple aryl ketones offer a unique opportunity to tune the redox properties for a given transformation through elaboration and ready modification of the primary ketone frameworks. In this context, and in consideration of the prevailing mode of photocatalysis with the currently available organic chromophores,6 we became interested in exploring the potential of aryl ketones as photoredox catalysts, specifically as excited-state reductants, in synthetically valuable bond-forming reactions. As an initial step, we disclose herein the efficient catalysis of appropriately modified thioxanthones under photoirradiation for the direct C–H imidation of arenes and heteroarenes (Scheme 1b). The applicability of thioxanthone catalysis to the C–H acyloxylation of arenes is also demonstrated.
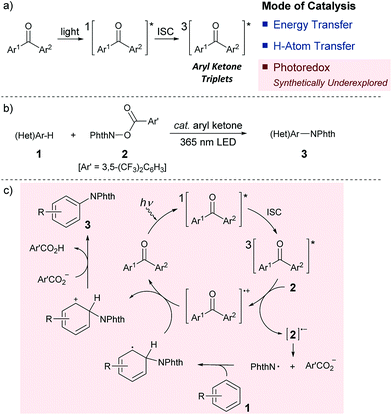 |
| Scheme 1 (a) Modes of photoexcited aryl ketone catalysis. (b) Photoexcited aryl ketone-catalyzed C–H imidation of arenes (Phth = phthaloyl). (c) Proposed catalytic cycle. | |
Aromatic and heteroaromatic amines constitute the core structural components of a wide array of functional organic molecules.7 Accordingly, the development of reliable methods for the assembly of arylamines has been a subject of central importance in synthetic chemistry, and direct arene C–H aminations have emerged as powerful means for this pursuit.8,9 Among the various strategies developed to date, the photocatalytic system reported by Sanford is unique,9a wherein a key nitrogen-based radical was generated from N-acyloxyphthalimide through one-electron reduction by an iridium-centred photosensitizer under visible light irradiation. This mechanistic proposal, in addition to the inherent synthetic value of C–N bond formation in its own right, inspired us to choose this class of C–H amination as a testing ground for photoredox ketone catalysis. We envisaged that if the triplet excited state of an aryl ketone could donate an electron to the aminating reagent, the corresponding anion radical would form with concomitant generation of a ketone cation radical. The anion radical then fragments to generate a requisite phthalimidyl radical that participates in the radical aromatic substitution process (Scheme 1c). At this stage, we recognized that aryl ketones are generally poor reductants, and distinguishing between the energy and electron transfer pathways may also be challenging.10 Nevertheless, we reasoned that the use of aryl ketones with appropriate structural features in combination with an electronically modulated N-acyloxyphthalimide would enable the establishment of an oxidative quenching cycle, thereby allowing the photoredox ketone-catalyzed C–H imidation of arenes.
Results and discussion
At the outset of our investigation to assess the validity of this hypothesis, we selected benzotrifluoride (1a) as a model substrate with the expectation that if a sufficient level of reactivity was attained with this generally less reactive arene, we could demonstrate the advantages of our approach through the reaction development (Table 1). An initial experiment was thus conducted by stirring a mixture of 3,5-bis(trifluoromethyl)phenylacyloxyphthalimide (2),111a (10 equiv.) and a catalytic amount of benzophenone (I) (5 mol%) in acetonitrile (CH3CN) under 365 nm LED light irradiation (1500 W m−2) at ambient temperature for 15 h. However, 1H-NMR analysis of the crude material showed very low conversion. Subsequent attempts with benzophenone derivatives, such as II and III, revealed that an electron-rich catalyst exhibited better efficacy, while changing the ketone skeleton to fluorenone (IV) turned out to be ineffective. To further evaluate the relationship between the structure and activity of aryl ketone catalysts, we examined the reaction in the presence of thioxanthone (V), which is known to have a long-lived triplet excited state, and observed an improved reactivity profile.12 On the other hand, the use of structurally related xanthone (VI) and 10-benzylacridin-9(10H)-one (VII) resulted in lower conversions. We next pursued the structural modification of the thioxanthone framework by introducing an electron-donating group to the 3-position, which had a notable yet beneficial impact on the catalytic activity. Under the influence of 3-methyl and 3-dimethylamino-substituted VIII and IX as catalysts, C–N bond formation occurred with significantly higher efficiency and the imidated product 3a was isolated in good yields. Interestingly, 3-methoxy derivative X exerted even higher catalytic activity. These observations led us to prepare 3,6-dimethoxy-9H-thioxanthen-9-one (XI) and we found that it delivered a critical improvement in the reactivity. Eventually, by increasing the loading of XI to 10 mol%, this imidation of the electron-deficient arene 1a proceeded smoothly to afford 3a in near quantitative yield (98%). Meanwhile, we screened other representative N-acyloxyphthalimides with different leaving abilities of the carboxylate anion as imidating agents; however, 2 remained optimal.13 It is also worth adding that the C–H imidation relied on the intensity of 365 nm LED, as the reaction under irradiation with an intensity of 500 W m−2 exhibited lower conversion (75%), whereas full conversion was observed with an intensity of 1000 W m−2 and 1500 W m−2.13
Table 1 Optimization for the photoexcited ketone-catalyzed C–H imidation of arenesa,b
The optimal catalyst and reaction conditions were applied to probe the scope of this photoexcited ketone-catalyzed C–H imidation protocol (Table 2). As summarized in Table 2, a broad range of arenes and heteroarenes underwent imidations in good to high yields under the catalysis of XI. The reactivity profile depended on the electronic nature of the arenes. The present system accommodated simple arenes, heteroarenes and electron-rich arenes, and the use of 5 mol% of XI was sufficient for smooth reactions. The imidations of electron-deficient arenes were generally challenging; however, a satisfactory level of reactivity could be attained by increasing the loading of XI to 10 mol%. It should be noted that the observed site selectivity is analogous to that anticipated for a radical aromatic substitution reaction.9b,14 Moreover, reactions with the arene as the limiting reagent also appeared feasible under slightly modified conditions, as exemplified by the direct installation of the phthalimide functionality onto caffeine.
Table 2 Substrate scope of the photoexcited ketone-catalyzed C–H imidation of arenesa,b
Having grasped the general applicability, we then studied the reaction mechanism with the primary objective of distinguishing the presumed electron transfer (ET) pathway from the possible alternative that involves energy transfer (EnT) from the triplet excited state of XI to the imidating agent 2, followed by homolytic cleavage of the N–O bond. This mechanistic study was initiated by measuring the UV-visible spectra of the representative catalysts, V and XI, and 2 in CH3CN, which revealed that only the catalyst has an absorption in the range of 365 nm. We then performed a reaction with benzotrifluoride (1a) under the optimized conditions but with light irradiation at fixed intervals, and observed that the reaction proceeded only when irradiated.13 We also detected a low quantum yield (Φ = 0.036) for the imidation.13 These results not only confirmed that photoexcitation was essential but also suggested the limited intervention of a radical chain process.15 Another useful piece of information to ascertain the involvement of the triplet excited state of the ketone catalyst was that the reaction was significantly suppressed by triplet quenchers (O2, pyridazine and 2,5-dimethylhexa-2,4-diene)16a (Scheme 2a).
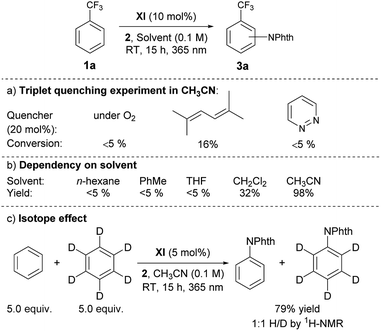 |
| Scheme 2 (a) Influence of triplet quenchers. (b) Solvent dependency. (c) Isotope effect. | |
Unlike reactions that proceed through EnT, this imidation reaction depended heavily on the solvent, and substantial product formation was observed only in CH3CN, a general characteristic of reactions involving ET processes (Scheme 2b).16 Furthermore, the ΔGet for XI was calculated to be −4.61 kcal mol−1 by the Rehm–Weller equation, indicating the feasibility of ET from XI to 2.13 At the same time, however, we recognized that these observations were still circumstantial, and thus, more compelling evidence was obtained by determining the triplet excited state energies and redox potentials of ketone catalysts XI, I and V, and the imidating agent 2, by measuring phosphorescence spectra and using cyclic voltammetry as well as theoretical calculations (Scheme 3).13
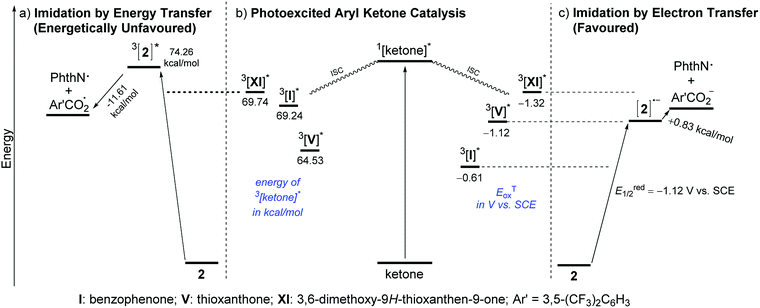 |
| Scheme 3 (a–c) Representative diagram for the comparison of C–H imidation through energy or electron transfer pathways (values determined through phosphorescence spectra, cyclic voltammetry measurements and UB3RYP/6-311+G(d,p) calculations).18 | |
As illustrated in Scheme 3a and b, the triplet excited state of 2, 3[2]*, has an energy of 74.26 kcal mol−1, whereas those of the ketones, 3[ketone]*, lie at much lower energy levels (3[XI]* = 69.74 kcal mol−1, 3[I]* = 69.24 kcal mol−1 and 3[V]* = 64.53 kcal mol−1). This energy gap between 3[ketone]* and 3[2]* (4.5 kcal mol−1 even for XI) is significant enough to preclude the possibility of the EnT pathway. In addition, even if the triplet excited state energy is a critical element for the ketone catalyst to be able to mediate the imidation, the nearly equal energy levels of 3[XI]* and 3[I]* could not rationalize the experimentally observed considerable difference in reactivity between I and XI. On the other hand, comparison of the triplet excited state oxidation potentials (ETox) of the ketones and the reduction potential (Ered1/2) of 2 strongly supported the operation of the ET pathway. Among XI, V and I with ETox values of −1.32 V, −1.12 V and −0.61 V, respectively, versus SCE, XI should be the most competent electron donor to 2 (Ered1/2 = −1.12 V vs. SCE), followed by V and I (Scheme 3b and c), which is in accordance with the experimental results.
The outcomes of these investigations prove that the photoexcited ketone-catalyzed direct arene imidation proceeds through an ET pathway, meaning that aryl ketones with suitable electronic properties, such as the optimal catalyst XI, act as excited-state reductants to establish an oxidative quenching cycle for radical aromatic substitution, as we initially postulated (Scheme 1b). The catalytic cycle commences with photoexcitation and subsequent ISC to afford 3[ketone]*. The ketone triplet with an appropriate oxidation potential donates an electron to the imidating agent, 2, to form a ketone cation radical ([ketone]˙+)17 and an anion radical of 2 ([2]˙−) that undergoes fragmentation to generate a phthalimidyl radical (PhthN˙) and a 3,5-bis(trifluoromethyl)benzoate anion. The PhthN˙ adds to the arene to bring forth a neutral radical species that can be oxidized by [ketone]˙+ to provide a Wheland intermediate and regenerate the ketone catalyst. Deprotonation of the Wheland intermediate by the 3,5-bis(trifluoromethyl)benzoate anion yields 3 and the corresponding carboxylic acid.19 It is noteworthy that the absence of the kinetic isotope effect rules out the possibility of C–H abstraction as a rate-determining step (Scheme 2c).
After establishing the C–H imidation of arenes, we decided to further explore the synthetic potential of photoexcited ketone catalysis and found it to be applicable to the C–H acyloxylation of simple arenes.20 For instance, light irradiation (325 W m−2) over a mixture of pentafluorobenzoyl peroxide (4) and benzene (10 equiv.) in CH3CN/DCE (1
:
1) in the presence of 3-methoxy-9H-thioxanthen-9-one (X) (10 mol%) at room temperature for 15 h resulted in the formation of the acyloxylated product 5a in good yield (Table 3).21 Other selected examples listed in Table 3 show the tolerance of the present system to the electronic property of arenes.
Table 3 Photoexcited ketone-catalyzed C–H acyloxylation of arenesa,b
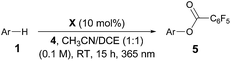
|
Reactions were carried out on a 0.2 mmol scale with 4 (1.0 equiv.) and arenes (10.0 equiv.) under light irradiation (325 W m−2).
Isolated yields of 5 are indicated.
|
|
Conclusions
In conclusion, we have developed a photoexcited ketone-catalyzed C–H imidation of arenes. Under simple and mild conditions, direct C–N bond formation proceeds efficiently with a broad range of arenes and heteroarenes. A distinct feature of this novel photocatalytic system is that the thioxanthone-derived catalyst behaves as an excited-state one-electron reductant and thus establishes an oxidative quenching cycle, as verified unambiguously through mechanistic investigations based on experimental and theoretical approaches. The utility of photoexcited ketone catalysis has also been demonstrated by application to the direct C–H acyloxylation of arenes. We believe that the present study indicates the possibility of designing and structurally manipulating simple aryl ketones to explore their potential utility as photoredox catalysts, which would be valuable in the development of unique synthetic transformations under organic photoredox catalysis.
Acknowledgements
This work is funded by the CREST-JST (JPMJCR13L2: 13418441) and Program for Leading Graduate Schools “Integrative Graduate Education and Research Program in Green Natural Sciences” at Nagoya University. We are grateful to Prof. T. Mori (Osaka University) for valuable discussion. MTC would like to thank JSPS for a postdoctoral fellowship.
References
-
(a) C. Chatgilialoglu, D. Crich, M. Komatsu and I. Ryu, Chem. Rev., 1999, 99, 1991 CrossRef CAS PubMed;
(b)
J. D. Coyle, Photochemistry of Organic Carbonyl Compounds, in Introduction to Organic Photochemistry, Wiley-VCH, Berlin, 1986, p.106 Search PubMed;
(c) J. D. Coyle and H. A. J. Carless, Chem. Soc. Rev., 1972, 1, 465 RSC;
(d) N. C. Yang, Photochem. Photobiol., 1968, 7, 767 CrossRef CAS.
-
(a) J. Zhao, W. Wu, J. Sun and S. Guo, Chem. Soc. Rev., 2013, 42, 5323 RSC;
(b) J. B. Borak and D. E. Falvey, Photochem. Photobiol. Sci., 2010, 9, 854 RSC;
(c) S. Hecht and J. M. J. Fréchet, J. Am. Chem. Soc., 2001, 123, 6959 CrossRef CAS;
(d) D. P. Specht, P. A. Martic and S. Farid, Tetrahedron, 1982, 38, 1203 CrossRef CAS.
- For significant contributions from the Bach group, see:
(a) S. Poplata, A. Tröster, Y.-Q. Zou and T. Bach, Chem. Rev., 2016, 116, 9748 CrossRef CAS PubMed;
(b) A. Tröster, R. Alonso, A. Bauer and T. Bach, J. Am. Chem. Soc., 2016, 138, 7808 CrossRef PubMed;
(c) R. Alonso and T. Bach, Angew. Chem., Int. Ed., 2014, 53, 4368 CrossRef CAS PubMed;
(d) S. C. Coote and T. Bach, J. Am. Chem. Soc., 2013, 135, 14948 CrossRef CAS PubMed;
(e) C. Müller, A. Bauer and T. Bach, Angew. Chem., Int. Ed., 2009, 48, 6640 CrossRef PubMed and references cited therein. For additional important contributions, see:
(f) K. Meier and H. Zweifel, J. Photochem., 1986, 35, 353 CrossRef CAS;
(g) R. Bensasson and E. J. Land, Trans. Faraday Soc., 1971, 67, 1904 RSC;
(h) D. L. Dexter, J. Chem. Phys., 1953, 21, 836 CrossRef CAS.
-
(a) S. Kamijo, K. Kamijo, K. Maruoka and T. Murafuji, Org. Lett., 2016, 18, 6516 CrossRef CAS PubMed;
(b) C. Chen, Org. Biomol. Chem., 2016, 14, 8641 RSC;
(c) J.-B. Xia, C. Zhu and C. Chen, Chem. Commun., 2014, 50, 11701 RSC;
(d) J.-B. Xia, C. Zhu and C. Chen, J. Am. Chem. Soc., 2013, 135, 17494 CrossRef CAS PubMed;
(e) M. Fagnoni, D. Dondi, D. Ravelli and A. Albini, Chem. Rev., 2007, 107, 2725 CrossRef CAS PubMed;
(f) A. Bauer, F. Westkamper, S. Grimme and T. Bach, Nature, 2005, 436, 1139 CrossRef CAS PubMed;
(g) L. Cokbaglan, N. Arsu, Y. Yagci, S. Jockusch and N. J. Turro, Macromolecules, 2003, 36, 2649 CrossRef CAS;
(h) C. Manfrotto, M. Mella, M. Freccero, M. Fagnoni and A. Albini, J. Org. Chem., 1999, 64, 5024 CrossRef CAS.
- When the outcome of the reactions proceeding through H-atom or photoelectron transfer is the same, it is rather challenging to ascertain the mode of catalysis (see ref. 6a). For reactions with a similar mechanistic perplexity, see:
(a) D. Harakat, J. Pesch, S. Marinkovic and N. Hoffmann, Org. Biomol. Chem., 2006, 4, 1202 RSC;
(b) S. Bertrand, N. Hoffmann, S. Humbel and J.-P. Pete, J. Org. Chem., 2000, 65, 8690 CrossRef CAS PubMed;
(c) S. Bertrand, N. Hoffmann and J.-P. Pete, Eur. J. Org. Chem., 2000, 2227 CrossRef CAS;
(d) S. Bertrand, C. Glapski, N. Hoffmann and J.-P. Pete, Tetrahedron Lett., 1999, 40, 3169 CrossRef CAS. For some studies on H-atom abstraction from alkylamines, see:
(e) S. Inbar, H. Linschitz and S. G. Cohen, J. Am. Chem. Soc., 1981, 103, 1048 CrossRef CAS;
(f) S. Inbar, H. Linschitz and S. G. Cohen, J. Am. Chem. Soc., 1980, 102, 1419 CrossRef CAS For the photoinduced aromatic trifluoromethylation reaction with acetone as the solvent and promoter, see: L. Li, X. Mu, W. Liu, Y. Wang, Z. Mi and C.-J. Li, J. Am. Chem. Soc., 2016, 138, 5809 CrossRef CAS PubMed . For the application of thioxanthone to the Ni-catalyzed homocoupling reaction, see: Y. Masuda, N. Ishida and M. Murakami, Eur. J. Org. Chem., 2016, 5822 CrossRef.
- For selected reports and reviews on photocatalysis, see:
(a) N. A. Romero and D. A. Nicewicz, Chem. Rev., 2016, 116, 10075 CrossRef CAS PubMed;
(b) J. Davies, T. D. Svejstrup, D. Fernandez Reina, N. S. Sheikh and D. Leonori, J. Am. Chem. Soc., 2016, 138, 8092 CrossRef CAS PubMed;
(c) R. A. Angnes, Z. Li, C. R. D. Correia and G. B. Hammond, Org. Biomol. Chem., 2015, 13, 9152 RSC;
(d) M. N. Hopkinson, B. Sahoo, J.-L. Li and F. Glorius, Chem.–Eur. J., 2014, 20, 3874 CrossRef CAS PubMed;
(e) T. Koike and M. Akita, Inorg. Chem. Front., 2014, 1, 562 RSC;
(f) C. K. Prier, D. A. Rankic and D. W. C. MacMillan, Chem. Rev., 2013, 113, 5322 CrossRef CAS PubMed;
(g) D. Ravelli, M. Fagnoni and A. Albini, Chem. Soc. Rev., 2013, 42, 97 RSC;
(h) J. M. R. Narayanam and C. R. J. Stephenson, Chem. Soc. Rev., 2011, 40, 102 RSC.
-
(a)
A. Ricci, in Amino group chemistry: from synthesis to the life sciences, Wiley-VCH, Weinheim, 2008 Search PubMed;
(b) L. Zhang, X.-M. Peng, G. L. V. Damu, R.-X. Geng and C.-H. Zhou, Med. Res. Rev., 2014, 34, 340 CrossRef CAS PubMed;
(c) M. Liang and J. Chen, Chem. Soc. Rev., 2013, 42, 3453 RSC;
(d) Z. Jin, Nat. Prod. Rep., 2011, 28, 1143 RSC;
(e) D. A. Horton, G. T. Bourne and M. L. Smythe, Chem. Rev., 2003, 103, 893 CrossRef CAS;
(f) D. J. Newman, G. M. Cragg and K. M. Snader, J. Nat. Prod., 2003, 66, 1022 CrossRef CAS.
-
(a) R. K. Rit, M. Shankar and A. K. Sahoo, Org. Biomol. Chem., 2017, 15, 1282 RSC;
(b) T. Xiong and Q. Zhang, Chem. Soc. Rev., 2016, 45, 3069 RSC;
(c) J. Jiao, K. Murakami and K. Itami, ACS Catal., 2016, 6, 610 CrossRef CAS;
(d) E. B. Corcoran, M. T. Pirnot, S. Lin, S. D. Dreher, D. A. DiRocco, I. W. Davies, S. L. Buchwald and D. W. C. MacMillan, Science, 2016, 353, 279 CrossRef CAS;
(e) G. B. Boursalian, W. S. Ham, A. R. Mazzotti and T. Ritter, Nat. Chem., 2016, 8, 810 CrossRef CAS PubMed;
(f) N. A. Romero, K. A. Margrey, N. E. Tay and D. A. Nicewicz, Science, 2015, 349, 1326 CrossRef CAS PubMed;
(g) T. Kawakami, K. Murakami and K. Itami, J. Am. Chem. Soc., 2015, 137, 2460 CrossRef CAS PubMed;
(h) E. Brachet, T. Ghosh, I. Ghosh and B. König, Chem. Sci., 2015, 6, 987 RSC;
(i) L. Song, L. Zhang, S. Luo and J.-P. Cheng, Chem.–Eur. J, 2014, 20, 14231 CrossRef CAS PubMed;
(j) S. L. McDonald, C. E. Hendrick and Q. Wang, Angew. Chem., Int. Ed., 2014, 53, 4667 CrossRef CAS PubMed;
(k) R. Shrestha, P. Mukherjee, Y. Tan, Z. C. Litman and J. F. Hartwig, J. Am. Chem. Soc., 2013, 135, 8480 CrossRef CAS PubMed;
(l) H. J. Kim, J. Kim, S. H. Cho and S. Chang, J. Am. Chem. Soc., 2011, 133, 16382 CrossRef CAS PubMed.
-
(a) L. J. Allen, P. J. Cabrera, M. Lee and M. S. Sanford, J. Am. Chem. Soc., 2014, 136, 5607 CrossRef CAS PubMed. For a conceptually similar phthalimide radical generation from N-chlorophthalimide, see:
(b) H. Kim, T. Kim, D. G. Lee, S. W. Roh and C. Lee, Chem. Commun., 2014, 50, 9273–9276 RSC.
- For an interesting study on competing energy and electron transfer pathways, see: S. Jockusch, H.-J. Timpe, W. Schnabel and N. J. Turro, J. Phys. Chem. A, 1997, 101, 440–445 CrossRef CAS.
- The imidating agent 2 can be stored at room temperature for months without any decomposition. Considering its stability and reactivity, the optimization of reaction conditions was carried out with 2.
- Our efforts to correlate the triplet lifetime (τ) of ketones with their catalytic activity were futile as no definite trend emerged from this analysis. However, the electron-rich ketones with pertinent triplet lifetimes were revealed to be effective for the C–H imidation. For details, see the ESI.†.
- See the ESI† for details.
- D. A. Nagib and D. W. C. MacMillan, Nature, 2011, 480, 224 CrossRef CAS PubMed.
- M. A. Cismesia and T. P. Yoon, Chem. Sci., 2015, 6, 5426 RSC.
- For the role of solvents in energy-transfer reactions, see:
(a) E. Arceo, E. Montroni and P. Melchiorre, Angew. Chem., Int. Ed., 2014, 53, 12064 CrossRef CAS PubMed;
(b) P. J. Wagner and I. Kochevar, J. Am. Chem. Soc., 1968, 90, 2232 CrossRef CAS.
- For carbonyl cation radicals in photochemical reactions, see:
(a) W. D. Cook and F. Chen, Polym. Chem., 2015, 6, 1325 RSC;
(b) M. R. Rodrigues and M. G. Neumann, Macromol. Chem. Phys., 2001, 202, 2776 CrossRef CAS;
(c) Y. Yaǧci, I. Lukáč and W. Schnabel, Polymer, 1993, 34, 1130 CrossRef For a review on thioxanthone-initiated photo-polymerization, see: S. Dadashi-Silab, C. Aydogan and Y. Yagci, Polym. Chem., 2015, 6, 6595 RSC.
-
M. J. Frisch, et al., Gaussian 09, revision D.01, Gaussian, Inc., Wallingford, CT, 2013 Search PubMed.
- We confirmed that the carboxylic acid was recovered in near quantitative yield. For details, see the ESI.†.
- For a photocatalytic C–H benzoyloxylation of electron-rich arenes, see: H. Rao, P. Wang and C.-J. Li, Eur. J. Org. Chem., 2012, 6503 CAS.
- For the detailed optimization of reaction conditions for the C–H acyloxylation of arenes, see the ESI.†.
Footnote |
† Electronic supplementary information (ESI) available: Experimental procedures and characterization data for all relevant compounds. CCDC 1544882. For ESI and crystallographic data in CIF or other electronic format see DOI: 10.1039/c7sc01700f |
|
This journal is © The Royal Society of Chemistry 2017 |
Click here to see how this site uses Cookies. View our privacy policy here.