DOI:
10.1039/C7SE00137A
(Review Article)
Sustainable Energy Fuels, 2017,
1, 1217-1231
Recent advances in quantum dot-sensitized solar cells: insights into photoanodes, sensitizers, electrolytes and counter electrodes
Received
11th March 2017
, Accepted 8th May 2017
First published on 8th May 2017
Abstract
Quantum dot-sensitized solar cells (QDSCs), as promising candidates for cost-effective photoelectrochemical solar cells, have attracted much attention due to their characteristic properties such as processability at low cost, feasibility to control light absorption spectrum in a wide region, and possibility of multiple electron generation with a theoretical conversion efficiency up to 44%. QDSCs have analogous structures to dye-sensitized solar cells typically consisting of semiconductor photoanodes sensitized with quantum dots (QDs), redox electrolytes, and counter electrodes (CEs). Much effort has been dedicated to optimizing each component of QDSCs for higher device performance. In this review, recent advances of photoanodes with various architectures, QDs with tunable band gaps, electrolytes in liquid, quasi-solid or solid state, and CEs with great electrocatalytic activity for QDSCs will be highlighted. We aim to elaborate the rational strategies in material design for QDSC applications. Finally, the conclusion and future prospects emphasize the key developments and remaining challenges for QDSCs.
Introduction
Solar energy is sustainable and particularly abundant as the most promising energy source for humanity's future. Several technologies have been exploited to convert solar photons into heat, electricity, fuel gas and biomass for practical use. Among these, solar cells are photovoltaic devices that directly convert sunlight into electricity via the photoelectric effect (e.g., silicon/thin film solar cells) or photochemical effect (e.g., dye-/quantum dot-sensitized solar cells (DSCs/QDSCs)).1,2 Of the various kinds of solar cells, QDSCs possess some distinct advantages, such as ease in fabrication, size-dependence of quantum dots (QDs) with tunable band gaps and high extinction coefficients, and potential of high power conversion efficiency (PCE) based on the generation of multiple excitons by the impact-ionization effect in QDs.3–7 Thus, QDSCs have been considered as promising candidates of the third-generation solar cells, and thus the number of relevant research articles is increasing nearly year by year (Fig. 1).
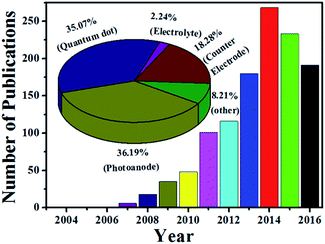 |
| Fig. 1 Evolution of the number of publications for quantum dot-sensitized solar cells. Inset is the share distribution of publications for each component of QDSCs in 2014. (Source: ISI Web of Science, Thomson Reuters. Date: January 1, 2017. Key words: quantum dot-sensitized solar cells.) | |
Essentially, QDSCs have a similar structure and working mechanism to DSCs. As shown in Fig. 2, a typical QDSC has a sandwich structure consisting of a several micron thick semiconductor photoanode (e.g., TiO2 or ZnO) sensitized with a moderate amount of QDs (e.g., CdS, CdSe or CdTe), an electrolyte containing a redox couple (e.g., S2−/Sx2−), and a counter electrode (CE; e.g., Pt, Cu2S or CoS).8 Under light illumination, the absorbed QDs on the photoanodes harvest some light within a certain region of wavelengths according to their band gaps to generate photo-excited electrons, which are subsequently injected from the conduction band (CB) of the QDs into the CB of the semiconductor and then quickly migrated to the external circuit through the conductive substrate, thereby creating an electric current. Meanwhile, holes are synchronously produced by the photo-excitation process in the valence band (VB) of the QDs, which are immediately transferred to the redox electrolyte to oxidize it. The oxidized electrolyte is restored by the electrons in the CE from the external circuit back to the cycling circuit in the cell.9 Briefly, QDs show a photovoltaic phenomenon when exposed to light, consequently producing photovoltage and photocurrent. The photovoltage (VOC) corresponds to the difference between the Fermi level (EF) of the QD-sensitized photoanode and the redox potential of the electrolyte. The photocurrent is strongly associated with the light harvesting efficiency as well as the electron separation, transport and collection efficiency of the cell devices.10
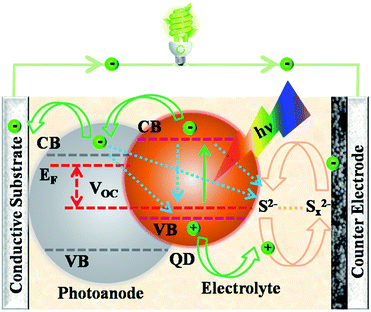 |
| Fig. 2 Operating principle and schematic diagram of the interfacial charge-transfer processes in a quantum dot-sensitized solar cell. | |
Many research experiments have been made for QDSCs in order to achieve high-performance devices.11,12 Each component of the cells vitally determines the cost and cell efficiency of QDSCs. Therefore, in past years, all the materials used in QDSCs have experienced rational design and careful modification (inset in Fig. 1 and 3), including the fabrication of nanostructured semiconductor photoanodes with effective dye loading and fast electron transport,13–15 the synthesis of versatile QDs with high absorption coefficients and appropriate band gaps to maximize light harvesting,16–18 the preparation of redox electrolytes with strong capacity for efficient hole transport and long-term stability,19–21 and the formation of various CEs with high electrocatalytic ability in reduction of the oxidized electrolyte.22,23 After a series of optimization processes, the best PCE of QDSCs up to 12% has been achieved.24
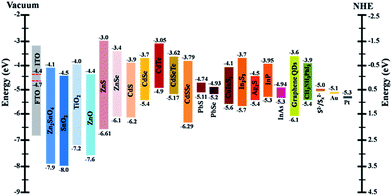 |
| Fig. 3 A summarized energy level diagram of some representative materials applied in QDSCs. | |
Hence, this review highlights recent advances with insights into the photoanodes, QDs, electrolytes and CEs of QDSCs. A general overview of these aspects in QDSCs will be given. Detailed information and comprehensive discussion of different issues for QDSCs can be found in some special review articles.25–29
Recent advances in photoanodes of QDSCs
Photoanodes composed of semiconductor films with various nanostructures tremendously influence the PCE of QDSCs.30 The functions of photoanodes in QDSCs can be summarized into two main aspects: (1) offer smooth electrical channels to facilitate the transfer of photo-excited electrons from photoanodes to conductive substrates and finally to the external circuit (Fig. 2); (2) provide sufficient surface area to load enough QDs for light absorption.31 Accordingly, a preferred photoanode should possess a fast electron transport rate to guarantee a high electron collection efficiency and a large surface area to ensure a high QD loading capacity. Therefore, intensive studies have been concentrated on these two issues.15,32–35 In general, TiO2 nanoparticle (NP) and ZnO nanowire (NW) films are the most widely used semiconductor photoanodes in QDSCs because of their attractive advantages, such as high electron mobility, large surface area, easy fabrication, high stability and low cost.33,36–38 During the past few years, considerable effort has been dedicated to designing and optimizing the preparation routes to form TiO2/ZnO photoanodes with increased QD loading, shortened electron transport pathways, and reduced charge recombination.39–42 These rational strategies of photoanodes can be roughly divided into several aspects: nanostructure design,43–46 additional treatment (e.g., TiCl4 immersion, etching, etc.),36,47,48 doping with ions (e.g., Zn,49 Nb,50 B/S,51etc.), and decoration with other materials (e.g., Au or Ag NPs,52,53 g-C3N4,54 graphene,55 carbon nanotubes (CNTs),56 Al2O3/MgO,57 up/down conversion crystals,58,59etc.). Next, the recent progress in the photoanodes of QDSCs will be discussed in detail according to the above mentioned aspects.
Nanostructure design is a popular way to obtain effective TiO2/ZnO photoanodes for QDSCs. As listed in Table 1, in addition to NPs and NWs, other morphologies, such as nanosheets (NSs),46,60,61 nanorods (NRs),62–64 microspheres (MSs),43,65–67 nanotubes (NTs),68,69 nanofibers (NFs),70,71 mesoporous (MPs),14,72 tetrapods (TPs),42,73 and nanodisks (NDs),74 have also been developed for TiO2/ZnO photoanodes. For example, “oriented attachment” TiO2 NRs prepared from a hydrothermal process showed a high QD loading capacity and efficient electrolyte diffusion ability due to the porous structures of the resultant films, in combination with a high electron diffusion coefficient and long electron lifetime due to their quasi-one-dimensional feature. Therefore, a best efficiency of 5.96% (Table 1) was achieved for the TiO2 NR-based QDSCs.35 Recently, complex hierarchical architectures constructed of two or more kinds of single structures together have also been intensively investigated since they are especially favourable to combine different merits of their building blocks. Several hierarchical structures, particularly branched or dendrite structures (denoted as “backbone-branch”), such as NRs–NTs,75 NRs–NSs,76 NWs–NWs,77 NFs–NTs78 and MPs–NWs15 of TiO2, NRs–NSs of ZnO,32,79 and TiO2 NFs–ZnO NSs,80 ZnO NRs–TiO2 NSs,40 TiO2 NWs–TiO2 NSs–ZnO NRs,34 and TiO2 NWs–ZnO NSs39 of hybrid materials, have been rationally designed for high-performance QDSCs. For instance, TiO2 NWs–TiO2 NSs–ZnO NRs hyperbranched heterostructured arrays were vertically grown on FTO substrates via a multi-step route (Fig. 4A). Compared to pristine TiO2 NWs, double-branched photoanodes are beneficial to enhance light harvesting due to the improved ability of light scattering and absorption (Fig. 4B) and strengthened charge collection capability due to the larger surface area for a thinner QD layer (Fig. 4C), ultimately leading to a peak efficiency of 5.38% for the optimized QDSCs with Cu2S CEs.34
Table 1 Photovoltaic parameters and photoactive materials of QDSCs based on different photoanodes
Photoanode |
Quantum dot |
Electrolyte |
Counter electrode |
J
SC (mA cm−2) |
V
OC (mV) |
FF |
PCE (%) |
Ref. |
TiO2 NPs |
CdSe/ZnS |
S2−/Sx2− |
Cu2S |
15.54 |
563 |
0.61 |
5.53 |
36
|
TiO2 NSs |
CdSe/ZnS/SiO2 |
S2−/Sx2− |
Cu2S |
16.95 |
591 |
0.50 |
5.01 |
60
|
TiO2 NRs |
CdSe/ZnS |
S2−/Sx2− |
Cu2ZnSnS(Se)4 |
17.5 |
563 |
0.605 |
5.96 |
35
|
TiO2 MSs |
CdS/CdSe/ZnS |
S2−/Sx2− |
Cu2S |
13.89 |
540 |
0.54 |
4.05 |
66
|
TiO2 NTs |
CdS/CdSe/ZnS |
S2−/Sx2− |
Cu2S |
10.81 |
689 |
0.62 |
4.61 |
68
|
TiO2 MPs |
CdS/CdSe/ZnS |
S2−/Sx2− |
Cu2S |
14.05 |
571 |
0.517 |
4.15 |
14
|
ZnO NWs |
CdS/CdSe |
S2−/Sx2− |
Au |
17.3 |
627 |
0.383 |
4.15 |
33
|
ZnO NRs |
CdS/CdSe/ZnS |
S2−/Sx2− |
Cu2S |
9.93 |
0.61 |
0.52 |
3.14 |
64
|
ZnO TPs |
CdS/CdSe |
S2−/Sx2− |
Pt |
13.85 |
722 |
0.424 |
4.24 |
42
|
ZnO NDs |
CdS/CdSe |
S2−/Sx2− |
Pt |
16.0 |
620 |
0.49 |
4.86 |
74
|
TiO2 NWs–NWs |
CdS/CdSe |
S2−/Sx2− |
Pt |
17.98 |
465 |
0.502 |
4.20 |
77
|
TiO2 NFs–NTs |
CdS/CdSe/ZnS |
S2−/Sx2− |
Carbon black |
8.8 |
610 |
0.503 |
2.8 |
78
|
TiO2 MPs–NWs |
CdS/CdSe |
S2−/Sx2− |
Cu2S |
19.32 |
531 |
0.586 |
6.011 |
15
|
ZnO NRs–NSs |
CdS/CdSe |
S2−/Sx2− |
Au |
14.2 |
631 |
0.48 |
4.4 |
79
|
ZnO NRs–NSs |
CdS/CdSe/ZnS |
S2−/Sx2− |
Cu2S |
10.74 |
610 |
0.50 |
3.28 |
32
|
TiO2 NFs–ZnO NSs |
CdS/CdSe/ZnS |
S2−/Sx2− |
Cu2S |
13.64 |
511 |
0.44 |
3.05 |
80
|
TiO2 NWs–TiO2 NSs–ZnO NRs |
CdS/CdSe |
S2−/Sx2− |
Cu2S |
19.19 |
517 |
0.54 |
5.38 |
34
|
TiO2 NWs–ZnO NSs |
CdS/CdSe |
S2−/Sx2− |
Cu2S |
16.11 |
512 |
0.55 |
4.57 |
39
|
TiO2 NFs/TiO2 NPs |
CdSe/ZnS |
S2−/Sx2− |
Cu2S |
11.92 |
503 |
0.54 |
3.24 |
71
|
TiO2 MSs/TiO2 NPs |
CdSe/ZnS |
S2−/Sx2− |
Cu2S |
14.06 |
608 |
0.55 |
4.70 |
81
|
ZnO NDs/TiO2 NPs |
CdS/CdSe |
S2−/Sx2− |
PbSe |
15.34 |
659 |
0.53 |
5.36 |
46
|
ZnO NPs + ZnO MSs |
CdS/CdSe/ZnS |
S2−/Sx2− |
Cu2S |
17.13 |
560 |
0.53 |
5.08 |
61
|
ZnO NWs + ZnO MSs |
CdS |
S2−/Sx2− |
Pt |
9.01 |
511 |
0.51 |
2.35 |
82
|
ZnO NRs + ZnO TPs |
CdS/CdSe/ZnS |
S2−/Sx2− |
Cu2S |
16.56 |
703 |
0.45 |
5.24 |
73
|
ZnO NRs/Ag NPs/TiO2 NPs |
CdS/CdSe |
S2−/Sx2− |
Cu2S |
15.65 |
744 |
0.508 |
5.92 |
52
|
ZnO NRs/g-C3N4 |
CdS |
S2−/Sx2− |
Pt |
11.1 |
650 |
0.34 |
2.43 |
54
|
ZnO NR/Zn2SnO4 MSs |
CdS/CdSe |
S2−/Sx2− |
Pt |
11.32 |
492 |
0.37 |
2.08 |
88
|
TiO2 NPs/MgO/Al2O3 |
CdS/CdSe |
S2−/Sx2− |
CuS |
11.4 |
630 |
0.56 |
3.25 |
57
|
SnO2 MSs |
CdS/CdSe/ZnS |
S2−/Sx2− |
Carbon NFs |
7.5 |
587 |
0.562 |
2.5 |
93
|
BiTiO3 NPs |
CdS/ZnS |
S2−/Sx2− |
Cu2S |
3.74 |
608 |
0.554 |
1.26 |
89
|
NiO MPs |
CuInSxSe2−x |
S2−/Sx2− |
CuxS |
9.13 |
350 |
0.39 |
1.25 |
91
|
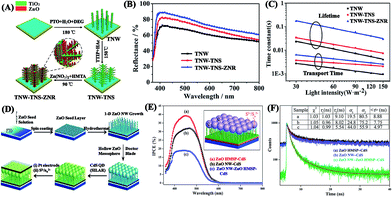 |
| Fig. 4 (A) Schematic diagram of the growth procedures and (B) diffuse reflectance spectra of hyperbranched TiO2 NW–TiO2 NS–ZnO NR heterostructured arrays, and (C) IMPS/IMVS tests of QDSCs based on those photoanodes (reproduced from ref. 34 with permission from the Royal Society of Chemistry). (D) Schematic diagram of the preparation routes and (E) IPCE plots of QDSCs based on double-layer photoanodes, and (F) time resolved PL spectra of different CdS-sensitized photoanodes (reproduced from ref. 82 with permission from the Royal Society of Chemistry). | |
Moreover, photoanode films prepared by simply mixing two different structures (denoted as “one structure/another structure”), such as TiO2 NFs/TiO2 NPs,71 TiO2 MSs/TiO2 NPs,81 and ZnO NDs/TiO2 NPs,46 are also available to enhance the performance of QDSCs. For example, an appropriate amount of ZnO NDs with higher conductivity was embedded into TiO2 NP films to reduce the resistance at the interface for effective electron transport, thus yielding an enhanced cell efficiency up to 5.36% (Table 1).46 Furthermore, photoanodes consisting of double layers (denoted as “under layer + upper layer”), such as ZnO NPs + ZnO MSs,61 ZnO NRs + ZnO TPs,73 and ZnO NWs + ZnO MSs,82 are well accepted to combine the different advantages of the two layers. For instance, a layer of hollow mesoporous ZnO MSs was covered onto another layer of vertical ZnO NW arrays to form a double-layered photoanode (Fig. 4D) with large surface area attributed to the upper layer (Fig. 4E), and fast electron transport ability for high charge collection associated with the under layer (Fig. 4F).82
The post-treatment of photoanodes via a TiCl4 solution is a common way to modify TiO2 films for DSCs and QDSCs.36,47 Such TiCl4 treatment can form a compact TiO2 layer with a thickness of hundreds of nanometers to improve the photovoltaic performance of devices. Recently, Zhong et al. studied the effect of TiCl4 treatment on FTO substrates and TiO2 mesoporous films with different modes (i.e., FTO/TiO2/TiCl4, FTO/TiCl4/TiO2, and FTO/TiCl4/TiO2/TiCl4). It was indicated that all the TiCl4 treatments were helpful to improve the performance of QDSCs with enhanced photocurrent due to the lower charge recombination rate. In particular, the sole TiCl4 treatment on FTO substrates is more efficient than any other modes on the TiO2 films.36
Ion doping is a common approach to tune the position of either the CBs or VBs of semiconductor materials for photocatalysis applications. For QDSCs, ion doping (e.g. B/S,51 N,83 Nb,50 Zn49 and Y84) has recently been applied to retard charge recombination and accelerate electron transfer in photoanodes. For example, Nb doping in TiO2 photoanodes could obviously increase the photovoltaic performance of the resultant QDSCs, primarily due to enhanced electron injection and electron transfer efficiency resulting from the negative shift in CB and the increased electron conductivity.50
Decoration with some useful materials is authentically beneficial to boost the photovoltaic performance of TiO2/ZnO photoanodes in DSCs and QDSCs. One kind of such decoration materials is novel metals, such as Au and Ag NPs, mainly owing to their surface plasmon resonance effect to localize incident light and extend the optical path length for increased light harvesting of QDSCs.52,53 Significantly, the incorporation of Ag NPs into TiO2/ZnO NRs not only enhanced light absorption and increased electron injection rate, but also reduced charge recombination and prolonged electron lifetime (Fig. 5A–C), consequently resulting in improved photocurrent (Table 1). More impressively, an enhanced photovoltage was also achieved due to the direct contact of Ag NPs with TiO2 NPs leading to Fermi level alignment with an upward shift of more negative potential in TiO2 NPs/Ag NPs/ZnO NRs (Fig. 5A).52 Another type of decoration materials is carbon-based materials, including CNTs,56 graphene,55 carbon dots,85 and graphitic carbon nitride (g-C3N4),54 largely due to their positive effects on the electron transport rate of QDSCs. Remarkably, the insertion of g-C3N4 sheets into ZnO NR photoanodes maximized the light harvesting efficiency of cell devices primarily because of the lower rate of photoinduced electron interception, the optimum loading of sensitizer QDs, and the stronger electronic interactions between g-C3N4 sheets and ZnO NRs (Table 1 and Fig. 5D–F).54 Finally, the introduction of some metal oxides (e.g., Al2O3/MgO,57 MoO3,86 BaTiO3 (ref. 87) and Zn2SnO4 (ref. 88)) into TiO2/ZnO photoanodes is also feasible to enhance the photovoltaic performance of QDSCs. For example, photoanodes of TiO2 NPs were coated with two different insulating metal oxides of MgO and Al2O3 to optimize the passivation effect and reduce the charge recombination losses for higher performance QDSCs as compared to the bare TiO2 films.57
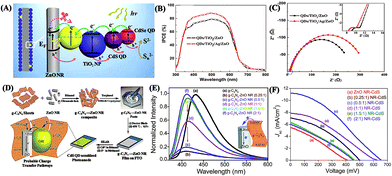 |
| Fig. 5 (A) Schematic of the charge behavior, (B) IPCE plots, and (C) EIS spectra of QDSCs based on photoanodes of TiO2 NPs/Ag NPs/ZnO NRs (reproduced from ref. 52 with permission from the American Chemical Society). (D) Schematic illustration of the fabrication process and charge transport of g-C3N4–ZnO NR composites, and (E) steady-state photoluminescence (PL) spectra of the composites with varying g-C3N4 to ZnO NR weight ratios and (F) I–V curves of the corresponding QDSCs (reproduced from ref. 54 with permission from the Royal Society of Chemistry). | |
Apart from TiO2 and ZnO, a series of other materials including BaTiO3,89 BiVO4,90 NiO,91 CoO,92 SnO2,93 SrTiO3,94 Zn2GaO4,95 Zn2SnO4,96–98 ZnTiO3
99 and ZrO2
100 have been exploited to act as photoanodes in QDSCs. For example, cauliflower-like SnO2 hollow MSs were sandwiched with carbon nanofibers as CEs to form QDSCs with a best PCE of 2.5%.101 NiO is a typical p-type semiconductor and has been used in p-type sensitized solar cells.91,102–104 The application of mesoporous NiO electrodes combined with CuInSxSe2−x QDs in p-type QDSCs could give a cell efficiency of 1.25% by optimizing the materials.91
Recent advances in quantum dots of QDSCs
QDs, as an essential part in a QDSC, should possess a high absorption coefficient and an appropriate band-gap energy to maximize the harvesting efficiency of the incident light.105,106 The energy levels of QDs applied in QDSCs must match those of the sensitized wide band-gap semiconductors. If the band-gap energy of the QDs is high, although a large VOC can be achieved, the wavelength range of light absorption will be narrowed concurrently. Contrarily, a low band-gap energy of QDs may contribute to a wide wavelength region of light absorption, but inevitably may lead to a low VOC.105 Accordingly, numerous research attempts have been carried out to develop different QDs with suitable band gaps as light harvesters in QDSCs, including Ag2S,107 Bi2S3,108 CdS,109 CdSe,110 CdTe,111 CuInS2,112,113 Cu2ZnSnS4,114 InAs,115 In2S3,116 InP,117 PbS,118,119 PbSe,120 Si,121 graphene,122 perovskite CH3NH3PbI3 (ref. 123) and so on (Fig. 3 and Table 2). The strategies for fabrication of high-performance QDs can be generally summarized into several types: QD type (e.g., co-sensitization,124 and ternary alloyed QDs125), synthesis methods (e.g., chemical bath deposition (CBD),126 successive ionic layer adsorption reaction (SILAR),127 and linker-assisted assembly (LAA)128), parameter optimization (e.g., size,129 layer,130 and coverage131), and modification treatment (e.g., doping,132 post-annealing,133 and deposition of passivation layers109).
Table 2 Photovoltaic parameters and photoactive materials of QDSCs based on different QDs
Quantum dot |
Method |
Photoanode |
Electrolyte |
Counter electrode |
J
SC (mA cm−2) |
V
OC (mV) |
FF |
PCE (%) |
Ref. |
Ag2S/ZnS |
SILAR |
TiO2 NPs |
I−/I3− |
Pt |
28.5 |
270 |
0.238 |
1.76 |
107
|
CdS/Bi2S3/ZnS |
SILAR |
TiO2 NPs |
S2−/Sx2− |
Cu2S |
9.3 |
502 |
0.537 |
2.52 |
108
|
CdS/CdSe |
ED |
TiO2 MSs |
S2−/Sx2− |
Pt |
18.23 |
489 |
0.54 |
4.81 |
136
|
CdSe/ZnS |
SILAR |
TiO2 NPs |
S2−/Sx2− |
Cu2S |
13.9 |
538 |
0.51 |
3.84 |
124
|
CdSe/ZnS |
LAA |
TiO2 NPs |
S2−/Sx2− |
Cu2S |
16.96 |
561 |
0.566 |
5.42 |
174
|
CdSe/ZnS |
EPD |
TiO2 NTs |
S2−/Sx2− |
Cu2S |
9.0 |
554 |
0.35 |
1.7 |
177
|
CuInS2/ZnS |
LAA |
TiO2 NPs |
S2−/Sx2− |
Cu2S |
20.65 |
586 |
0.581 |
7.04 |
112
|
CuInSe/ZnS |
LAA/SILAR |
TiO2 NPs |
S2−/Sx2− |
Cu2S |
26.93 |
528 |
0.57 |
8.10 |
16
|
CuInSexS2−x/ZnS |
LAA |
TiO2 NPs |
S2−/Sx2− |
Cu2S |
16.8 |
560 |
0.59 |
5.51 |
151
|
Cu2ZnSnS4/ZnS |
LAA |
TiO2 NPs |
S2−/Sx2− |
Cu2S |
18.86 |
510 |
0.494 |
4.70 |
114
|
CdS/CdSe/ZnS |
CBD |
TiO2 NPs |
S2−/Sx2− |
Au |
16.8 |
514 |
0.49 |
4.22 |
126
|
CdS/CdSe/CdS/ZnS |
CBD/SILAR |
TiO2 NTs |
S2−/Sx2− |
Cu2S |
17.7 |
555 |
0.557 |
5.47 |
109
|
CdS/CdSe/ZnS |
LAA |
TiO2 NPs |
S2−/Sx2− |
Cu2S |
18.02 |
527 |
0.56 |
5.32 |
128
|
CdS/CdSe/ZnSe |
SILAR |
TiO2 NPs |
S2−/Sx2− |
Cu2S |
20.11 |
580 |
0.55 |
6.39 |
166
|
ZnCuInSe/ZnS |
LAA |
TiO2 NPs |
S2−/Sx2− |
Carbon |
25.25 |
739 |
0.622 |
11.61 |
18
|
Mn–CdS/CdSe/ZnS |
SILAR |
TiO2 NPs |
S2−/Sx2− |
Cu2S |
20.7 |
558 |
0.47 |
5.42 |
132
|
Ni-doped CdS |
SILAR |
TiO2 NPs |
S2−/Sx2− |
CuS |
8.91 |
643 |
0.543 |
3.11 |
178
|
CdS/CdSe/Mn–ZnSe |
SILAR |
TiO2 NPs |
S2−/Sx2− |
CuS |
17.59 |
584 |
0.551 |
5.67 |
185
|
CdSexTe1−x/ZnS |
LAA |
TiO2 NPs |
S2−/Sx2− |
Cu2S |
19.35 |
571 |
0.575 |
6.36 |
125
|
CdSexTe1−x/ZnS/SiO2 |
LAA |
TiO2 NPs |
S2−/Sx2− |
Cu2S |
20.78 |
653 |
0.605 |
8.21 |
189
|
CdSexTe1−x/CdS/ZnS/SiO2 |
LAA |
TiO2 NPs |
S2−/Sx2− |
Cu2S |
20.82 |
712 |
0.639 |
9.48 |
155
|
CdSxSe1−x/CdSe/ZnS |
SILAR |
TiO2 NPs |
S2−/Sx2− |
Cu2S |
14.22 |
557 |
0.553 |
4.46 |
148
|
CdTe/CdSexTe1−x/ZnS |
LAA/SILAR |
TiO2 NPs |
S2−/Sx2− |
Cu2S |
16.58 |
629 |
0.694 |
7.24 |
111
|
CdS/CdSxSe1−x/CdSe/ZnS |
SILAR |
TiO2 NPs |
S2−/Sx2− |
Cu2S |
14.65 |
640 |
0.54 |
5.06 |
147
|
CdTe/CdSe/ZnS |
LAA |
TiO2 NPs |
S2−/Sx2− |
Cu2S |
19.59 |
606 |
0.569 |
6.76 |
139
|
CdTe/CdS/CdS |
LAA/SILAR |
TiO2 NPs |
S2−/Sx2− |
Cu2S |
20.19 |
610 |
0.51 |
6.32 |
143
|
PbxCd1−xS/CdS/ZnS |
SILAR |
TiO2 NPs |
S2−/Sx2− |
Cu2S |
24.47 |
421 |
0.52 |
5.33 |
140
|
PbS/CdS/ZnS |
LAA |
TiO2 NPs |
S2−/Sx2− |
Cu2S |
18.81 |
595 |
0.642 |
7.19 |
141
|
ZnTe/CdSe/ZnS |
LAA |
TiO2 NPs |
S2−/Sx2− |
Cu2S |
19.29 |
640 |
0.552 |
6.82 |
146
|
CH3NH3PbI3 |
CBD |
TiO2 NPs |
I−/I3− |
Pt |
15.82 |
706 |
0.586 |
6.54 |
123
|
Among various QDs, Cd chalcogenide QDs (Fig. 3; e.g., CdS,134 CdSe135 and CdTe111) are preferred because of their relatively high stability in QDSCs even though they maybe unstable under visible light. Nevertheless, other kinds of QDs with narrow band gaps (Fig. 3), such as PbS,118 PbSe,120 Ag2S107 and InAs,115 have also been considered due to their abilities to widen the light absorption into the infrared (IR) region. In addition, co-sensitization of two or three types of QDs, for example, CdS/CdSe,136 CdS/CdTe,137 CdSe/CdTe,138,139 CdS/PbS,140,141 CdS/CdSe/PbS,142 CdTe/CdS/CdS,143 CdS/CuInS2,17 CuInS2/In2S3,144 In2S3/PbS,145 and ZnTe/CdSe,146 is well accepted because of the complementary effect of extending the wavelength range of light harvesting, and/or facilitating the electron transfer process. More significantly, the design of alloy materials, such as CdSexS1−x,147,148 CdSexTe1−x,149,150 CuInSexS2−x,151 and Zn–Cu–In–Se,18,152 has recently attracted much attention since it is feasible to engineer the physical characteristics (e.g., band gaps, energy band positions and photoelectrical properties) of QDs through controlling the compositional ratio between each chalcogenide and metal element.125 For example, Zn–Cu–In–Se QDs with an absorption onset extending to ∼1000 nm were successfully fabricated for high-efficiency QDSCs with a champion PCE of 11.91% and a certified PCE of 11.61% (Table 2). The remarkably photovoltaic performance was supposed to be associated with the high loading capacity of QDs, broad light harvesting range stretching to near-IR light (Fig. 6A and B), and reduced charge recombination rate and fast electron extraction from the special alloyed structure of Zn–Cu–In–Se QDs (Fig. 6C).18 CdSexTe1−x alloyed QDs are another promising alternative due to their intrinsic superior optoelectronic properties, such as an absorption edge extending to the near-IR region (Fig. 6D), a high conduction band edge, and high chemical stability.125 Accordingly, the application of CdSexTe1−x alloyed QDs with good crystalline quality and high surface coverage in QDSCs showed a stable cell efficiency of 6.36% (Table 2 and Fig. 6E and F).125 By controlling the semiconductor photoanodes, a higher efficiency up to 7.55% was obtained.153 After introducing Mn-doped CdSe and CdS coating layers onto the CdSexTe1−x alloyed QDs, the corresponding efficiencies were further improved to 8.14% (ref. 154) and 9.48%,155 respectively. In addition, QDSCs employing a sensitizer with a structure of Mn doped CdSexTe1−x/Mn doped ZnS/SiO2 also showed a best efficiency of 9.40%.156 Moreover, when adopting Ti mesh supported mesoporous carbon (MC/Ti) films as CEs, the CdSe0.65Te0.35-based QDSCs exhibited a certified efficiency of 11.16%.157
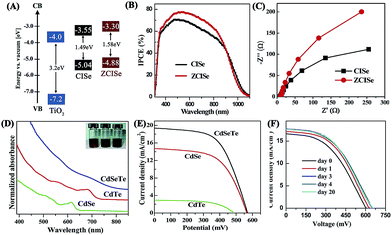 |
| Fig. 6 (A) Schematic energy level diagrams of TiO2, Cu–In–Se (CISe), and Zn–Cu–In–Se (ZCISe) QDs, (B) IPCE spectra and (C) Nyquist plots of the corresponding QDSCs (reproduced from ref. 18 with permission from the American Chemical Society). (D) Absorption spectra of CdSexTe1−x, CdTe, and CdSe QD aqueous dispersions, (E) J−V curves of the corresponding QDSCs, and (F) temporal evolution of J−V curves of the QDSCs based on CdSexTe1−x QDs (reproduced from ref. 125 with permission from the American Chemical Society). | |
To date, a series of routes have been developed to prepare QDs with high crystalline quality and well-defined particle sizes. Among them, CBD, SILAR and LAA are the three common techniques to synthesize and incorporate QDs into photoanodes as sensitizers (Table 2). CBD is one simple and cost-effective way to directly grow QDs onto nanostructured photoanodes by immersing photoactive electrodes into a solution containing the cationic (e.g., Cd) and anionic (S and Se) precursors for the slow precipitation of the desired QDs.110,158 Intriguingly, some additional procedures, such as hydrothermal treatment,133 microwave,159,160in situ electric field,161 and linker seeding,162,163 recently were adopted to assist the preparation of QDs via CBD for a facile, fast and effective deposition. SILAR is another simple and reproducible method involving dipping the nanostructured electrodes into different solutions in succession.107,135,164 For one deposition cycle of QDs, the electrodes are firstly dipped into a solution with cationic precursor (e.g., Cd, Ag and Pb) to strongly adsorb the desired ions, subsequently water rinsing is performed to remove the excess solution on the electrodes, and finally a second dipping of the above electrodes into a new solution containing the corresponding anionic precursor (e.g., S and Se) for chemical reaction with the pre-adsorbed cations.165,166 The particle sizes and quality of QDs prepared by SILAR can be readily optimized by tuning the reaction conditions, including deposition cycle,124 concentration and type of precursors,134,167 solution acidity,168 some additives,169 and so on. LAA involves the deposition of ex situ grown QDs onto nanostructured photoanodes.111,170,171 In general, monodisperse QDs are pre-synthesized by using capping agents to adjust the nanocrystal size, shape and light absorption properties, and then they are cleaned and dissolved in an organic solvent for use. Meanwhile, the metal oxide photoanodes are soaked in a solution with a bifunctional molecular linker (e.g., (COOH)-R-SH, like mercapto-carboxylic acid). The carboxyl groups of this bifunctional molecular are able to link with the metal oxide, while the mercapto groups wait for the attachment with QDs. After immersing the modified metal oxide electrodes into the above mentioned solution of QDs, the mercapto groups assist the adsorption of QDs onto the surface of the metal oxide films typically by partial ligand exchange.172–174 Additionally, other methods, such as pulsed laser deposition,175 electrodeposition (ED),136 spray pyrolysis deposition,176 and electrophoretic deposition (EPD),120,177 also can be found in some of the literature.
Metal ion dopants are usually employed to manipulate the optoelectronic properties of QDs in QDSCs. A variety of transition metal ions, such as Mn2+,132 Ni2+,178 Co2+,179 and Cu+,180 have been reported recently for doping into QDs. Particularly, the doping of Mn2+ is most commonly used in QDs (e.g., CdS,181 CdSe,182 CdSexTe1−x,156 and PbS183), which may produce new sub-band-gap electronic states due to the Mn d–d transition with a long emission lifetime of a few hundred microseconds and then significantly affect the charge transfer and recombination kinetics of QDs (Fig. 7A–C).132 Moreover, coating a passivation layer (e.g., ZnS,109 ZnSe,184,185 CuxS,186 TiO2
187 and Al2O3
188) onto QDs is also helpful to enhance the photovoltaic performance of QDSCs. For example, a thin ZnS overlayer onto CuInSe2 QD-sensitized TiO2 electrodes did not influence the energetic characteristics of the photoanodes; on the contrary, it was capable of reducing the interfacial electron recombination with electrolyte and nonradiative recombination associated with QDs, therefore yielding a best PCE of 8.10% (Fig. 7D–F).16 Recently, a combined ZnS/SiO2 treatment on CdSexTe1−x QDs was revealed to retard the interfacial recombination and improve the charge collection efficiency as compared with the conventional ZnS treatment alone.189 In addition, an Al2O3 overlayer prepared by atomic layer deposition can remarkably boost the photoelectrochemical performance of PbS/CdS QD-sensitized TiO2 nanotube arrays, primarily due to surface recombination passivation, catalytic improvement in interfacial charge transfer kinetics, and chemical stabilization.188
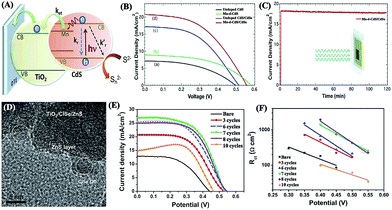 |
| Fig. 7 (A) Schematic diagram illustrating electron transfer (ket), (B) J–V characteristics, and (C) photocurrent stability of Mn-doped CdS/CdSe (reproduced from ref. 132 with permission from the American Chemical Society). (D) TEM image of CuInSe2-sensitized TiO2 after 7 ZnS SILAR cycles, (E) J–V characteristics and (F) recombination resistance of the CuInSe2 QDSCs with respect to the number of ZnS SILAR cycles (reproduced from ref. 16 with permission from the American Chemical Society). | |
Recent advances in electrolytes of QDSCs
Redox electrolytes, as the mediums to transfer charge between counter electrodes and photoanodes for the regeneration of oxidized QDs, significantly influence both the efficiency and stability of QDSCs.25 Although the iodide/triiodide (I−/I3−) couple serves as the most efficient electrolyte in DSCs, cadmium chalcogenide QD-based QDSCs are unstable in the iodide/triiodide electrolyte because of photoanodic dissolution and the formation of cadmium iodide. Thus, a polysulfide (S2−/Sn2−) redox couple aqueous solution electrolyte is commonly applied in QDSCs due to its ability to stabilize the cadmium chalcogenide QDs with a fairly decent performance of the corresponding QDSCs.190 However, there are still some disadvantages of the polysulfide electrolyte; for example, the VOC of the resultant QDSCs is usually lower than 0.65 V due to the relatively high redox potential of the polysulfide redox couple.8 Hence, many works have been done to optimize the polysulfide electrolyte, including controlling the concentration of the redox mediator,21 introducing additives (e.g., SiO2,191 polyethylene glycol (PEG),19 poly(vinyl pyrrolidone) (PVP),192 and guanidine thiocyanate193), and using a modifying solvent.194 For example, it was reported that the addition of SiO2 NPs into the polysulfide electrolyte enabled the formation of an energy barrier for the recombination at the photoanode/electrolyte interface (Fig. 8A), thereby contributing a higher electron collection efficiency (98%) (Fig. 8B) and a longer electron lifetime (Fig. 8C) for the resultant CdSexTe1−x-based QDSCs with an impressive PCE of 11.23%.191 In addition, alternative redox couples with relatively low redox potentials, such as [Co(o-phen)3]2+/3+, Fe(CN)63−/4−, Mn poly(pyrazolyl)borate and some organic redox couples,195,196 have been exploited to improve the photovoltaic performance, especially the VOC values of QDSCs. Unfortunately, the stability of the most popular cadmium chalcogenide QDs in these electrolytes is still a big problem.
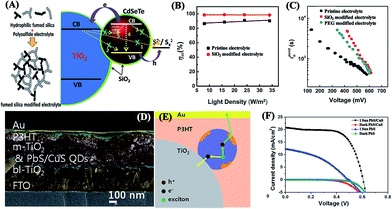 |
| Fig. 8 (A) Schematic illustration of the role of SiO2 NPs in the polysulfide electrolyte as well as the electron transport and charge recombination processes, (B) charge collection efficiency (ηcc), and (C) plots of electron lifetime in CdSexTe1−x QDSCs (reproduced from ref. 191 with permission from the Royal Society of Chemistry). (D) SEM cross-sectional image, (E) illustration of the proposed working mechanism, and (F) I–V curves of solid-sate QDSCs (reproduced from ref. 212 with permission from the American Chemical Society). | |
Moreover, for liquid electrolytes, sealing problems and long-term durability related to the leakage and volatilization of the liquid solution substantially hinder the practical applications of QDSCs. Thus, alternatives to liquid electrolytes, that is, quasi-solid-state and solid-state electrolytes have been developed as well. Ionic liquids,197 gel electrolytes,198–202 and hydrogel electrolytes203,204 containing redox couples are commonly used as quasi-solid-state electrolytes to overcome the stability challenge of liquid electrolytes. For example, a gel electrolyte containing sodium carboxymethylcellulose (CMC-Na) to solidify a polysulfide aqueous solution showed superabsorbent and water-holding capability with a high ion mobility in the three dimensional (3D) porous network, and then a notable PCE of 9.21% was achieved when it was applied in CdSexTe1−x-sensitized QDSCs.205 Solid-state electrolytes, including various hole-transporting materials, are also popular due to their relatively high stability. Organic polymers (e.g. 2,2′,7,7′-tetrakis(N,N-di-4-methoxyphenylamino)-9,9′-spirobifluorene (spiro-MeOTAD),206–209 poly(3-hexylthiophene) (P3HT),210–212 3,3′′′-didodecylquaterthiophene (QT12),213 PVP,214 and poly(ethylene oxide)-poly(vinylidene fluoride) (PEO-PVDF)215) have been successfully employed in solid-state QDSCs. For instance, solid-state QDSCs with a structure of FTO/blocking TiO2/mesoscopic TiO2/PbS/CuS QDs/P3HT/Au presented an impressive PCE of 8.07% (Fig. 8D–F; VOC: 0.6 V, JSC: 20.7 mA cm−2, and FF: 0.65).212
Recent advances in counter electrodes of QDSCs
CEs in QDSCs have the important task of transferring electrons from the external circuit and catalyzing the reduction reaction of the oxidized electrolytes at the electrolyte/CE interface. To ensure a superior electrocatalytic performance, CEs should possess high electrical conductivity, excellent electrocatalytic activity and great stability.10,216 Noble metals, especially Pt films, are the most common CE materials in DSCs due to their good electrocatalytic ability for the reduction of iodide/triiodide electrolyte. However, Pt CEs are poorly active in polysulfide electrolyte since sulfur atoms would easily adsorb onto the Pt surface to lower the conductivity and then cause a considerable overpotential for electrolyte reduction, consequently reducing the device efficiency of QDSCs.23 Accordingly, alternative CE materials in QDSCs have been extensively investigated, which can be generally summarized into several main types (Table 3): metal chalcogenides,217,218 carbon-based materials,219,220 and various composites.22,221,222
Table 3 Photovoltaic parameters and photoactive materials of QDSCs based on different CEs
Counter electrode |
Photoanode |
Quantum dot |
Electrolyte |
J
SC (mA cm−2) |
V
OC (mV) |
FF |
PCE (%) |
Ref. |
Cu2S/brass |
TiO2 NPs |
CdS/CdSe/ZnS |
S2−/Sx2− |
14.54 |
634 |
0.667 |
6.15 |
226
|
Cu2−xSe NPs |
TiO2 NPs |
CdSeTe/ZnS/SiO2 |
S2−/Sx2− |
21.09 |
673 |
0.614 |
8.72 |
24
|
Cu7S4 NTs |
TiO2 NPs |
CdS/CdSe/ZnS |
S2−/Sx2− |
19.09 |
480 |
0.494 |
4.53 |
234
|
Cu1.8S NSs |
TiO2 MSs |
CdS/CdSe |
S2−/Sx2− |
16.07 |
500 |
0.41 |
3.30 |
237
|
CuS NSs |
TiO2 NPs |
CdS/CdSe/ZnS |
S2−/Sx2− |
17.43 |
596 |
0.495 |
5.15 |
238
|
CuS NPs |
TiO2 NPs |
CdSe/ZnS |
S2−/Sx2− |
17.7 |
551 |
0.491 |
4.78 |
239
|
CuS MSs |
TiO2 NPs |
CdS/CdSe |
S2−/Sx2− |
16.05 |
550 |
0.489 |
4.32 |
240
|
Mn-doped CuS NPs |
TiO2 NPs |
CdS/CdSe |
S2−/Sx2− |
17.58 |
583 |
0.532 |
5.46 |
241
|
Cu1.8Se NSs |
TiO2 NPs |
CdS/CdSe/ZnS |
S2−/Sx2− |
20.5 |
540 |
0.50 |
5.01 |
242
|
Cu2−xSe NTs |
TiO2 NPs |
CdS/CdSe |
S2−/Sx2− |
18.85 |
559 |
0.593 |
6.25 |
243
|
Cu2−xSe NPs |
TiO2 NPs |
CdS/CdSe/ZnS |
S2−/Sx2− |
21.39 |
567 |
0.586 |
7.11 |
244
|
Cu2SnSe3 NPs |
TiO2 NPs |
CdSe |
S2−/Sx2− |
16.29 |
563 |
0.54 |
4.93 |
218
|
Co9S8 NRs |
TiO2 NRs |
CdS/CdSe |
S2−/Sx2− |
16.80 |
480 |
0.462 |
3.72 |
217
|
Cu-doped CoS NSs |
TiO2 NPs |
CdSe0.45Te0.55/ZnS |
S2−/Sx2− |
18.2 |
565 |
0.593 |
6.1 |
246
|
CoS2 NPs |
TiO2 MSs |
CdS/CdSe |
S2−/Sx2− |
14.44 |
510 |
0.565 |
4.16 |
247
|
FeS2 MSs |
ZnO NWs |
ZnSe/CdSe/ZnS |
S2−/Sx2− |
13.58 |
743 |
0.387 |
3.90 |
248
|
FeS NSs/Ni foam |
TiO2 NPs |
CdS/CdSe |
S2−/Sx2− |
18.70 |
410 |
0.58 |
4.39 |
250
|
MoS2 NSs |
TiO2 NPs |
CdS/ZnS |
S2−/Sx2− |
6.22 |
477 |
0.41 |
1.21 |
251
|
NiS NPs |
TiO2 NPs |
CdS/CdSe/ZnS |
S2−/Sx2− |
11.67 |
493 |
0.527 |
3.03 |
253
|
NiCo2S4 NRs |
TiO2 NPs |
CdSe/ZnS |
S2−/Sx2− |
15.58 |
550 |
0.489 |
4.22 |
254
|
Cu2ZnSnS4 MSs |
ZnO NWs |
ZnSe/CdSe |
S2−/Sx2− |
11.06 |
822 |
0.41 |
3.73 |
255
|
Cu2ZnSn(S1−xSex)4 |
TiO2 NPs |
CdS/CdSe/ZnS |
S2−/Sx2− |
12.71 |
550 |
0.43 |
3.01 |
258
|
PbS NPs |
TiO2 NPs |
CuInS2/CdS/ZnS |
S2−/Sx2− |
18.3 |
580 |
0.45 |
4.7 |
261
|
PbSe NPs |
TiO2 NPs |
CdS/CdSe/ZnS |
S2−/Sx2− |
16.91 |
570 |
0.48 |
4.67 |
262
|
CuAgSe NTs |
TiO2 NPs |
CdS/CdSe |
S2−/Sx2− |
17.57 |
581 |
0.55 |
5.61 |
265
|
CoS MSs/NiS NPs |
TiO2 NPs |
CdS/CdSe/ZnS |
S2−/Sx2− |
14.76 |
590 |
0.53 |
4.70 |
268
|
NiS NPs/PbS NPs |
TiO2 NPs |
CdS/CdSe/ZnS |
S2−/Sx2− |
14.52 |
596 |
0.539 |
4.52 |
271
|
CNTs |
TiO2 NPs |
CdS/CdSe/ZnS |
S2−/Sx2− |
16.09 |
586 |
0.495 |
4.67 |
219
|
Carbon NFs |
TiO2 NPs |
CdSe/ZnS |
S2−/Sx2− |
11.99 |
620 |
0.60 |
4.81 |
274
|
Carbon NPs |
TiO2 MPs |
CdS/CdSe/ZnS |
S2−/Sx2− |
13.53 |
510 |
0.40 |
2.67 |
276
|
Carbon MSs |
TiO2 NPs |
CdSe/ZnS |
S2−/Sx2− |
12.41 |
600 |
0.52 |
3.90 |
277
|
Carbon foam |
ZnO NWs |
CdS/CdSe |
S2−/Sx2− |
12.6 |
685 |
0.42 |
3.60 |
278
|
C60 |
TiO2 NPs |
CdS/CdSe/ZnS |
S2−/Sx2− |
12.6 |
546 |
0.60 |
4.18 |
279
|
AuPt NPs/graphene |
ZnO NWs |
CdS/CdSe |
S2−/Sx2− |
15.2 |
720 |
0.409 |
4.5 |
280
|
Pt/CuS |
TiO2 NPs |
CdS/CdSe/ZnS |
S2−/Sx2− |
15.31 |
600 |
0.46 |
4.22 |
281
|
Au/CuS NSs |
TiO2 NPs |
PbS/ZnS |
S2−/Sx2− |
24.17 |
420 |
0.564 |
5.73 |
282
|
Cu2S MSs/RGO |
TiO2 MSs |
CdS/CdSe |
S2−/Sx2− |
15.85 |
556 |
0.44 |
3.85 |
23
|
CuS NPs/carbon NFs |
TiO2 NPs |
CdS/CdSe/ZnS |
S2−/Sx2− |
14.46 |
521 |
0.507 |
3.86 |
286
|
CuS NPs/3D graphene |
TiO2 NPs |
CdS/CdSe/ZnS |
S2−/Sx2− |
16.19 |
590 |
0.528 |
5.04 |
287
|
Cu1.18S/GO nanoribbon |
TiO2 NPs |
CdTe/CdS/CdS |
S2−/Sx2− |
20.55 |
626 |
0.53 |
6.81 |
288
|
FeS/carbon NPs |
TiO2 NPs |
CdS/CdSe |
S2−/Sx2− |
20.33 |
440 |
0.51 |
4.58 |
289
|
PbS NPs/graphene |
TiO2 NPs |
CdS/CdSe/ZnS |
S2−/Sx2− |
11 |
559 |
0.42 |
2.63 |
290
|
ITO NWs/Cu2S NPs |
TiO2 NPs |
CdS/CdSe/ZnS |
S2−/Sx2− |
14.31 |
540 |
0.525 |
4.06 |
291
|
CuS NPs/ZnO NRs |
ZnO NRs |
CdS/CdSe |
S2−/Sx2− |
14.48 |
760 |
0.38 |
4.18 |
292
|
PbS NPs/ZnO NRs |
TiO2 NPs |
CdS/CdSe/ZnS |
S2−/Sx2− |
13.28 |
633 |
0.566 |
4.76 |
293
|
NiS NPs/PANI NFs |
TiO2 NPs |
CdSe |
S2−/Sx2− |
13.62 |
610 |
0.50 |
4.15 |
294
|
Mo2N/CNT–RGO |
ZnO NWs |
CdS/CdSe |
S2−/Sx2− |
16.93 |
680 |
0.47 |
5.41 |
295
|
Carbon MPs/Ti mesh |
TiO2 NPs |
CdSe0.65Te0.35/ZnS/SiO2 |
S2−/Sx2− |
20.68 |
798 |
0.68 |
11.16 |
157
|
CNT/Cu2ZnSnSe4 NPs |
TiO2 NPs |
CdSe/ZnS |
S2−/Sx2− |
17.04 |
530 |
0.51 |
4.60 |
256
|
TiN NPs/CNTs–RGO |
ZnO NWs |
CdS/CdSe |
S2−/Sx2− |
14.0 |
642 |
0.46 |
4.13 |
222
|
TiC NPs |
TiO2 NPs |
CdS |
S2−/Sx2− |
8.51 |
530 |
0.47 |
2.11 |
297
|
Metal chalcogenides, including a large variety of compounds, have been much exploited to replace the conventional Pt or Au CEs for DSCs and QDSCs.223,224 Among these materials, Cu2S CEs are the most popular choice due to their relatively high electrocatalytic activity in the polysulfide redox system. Normally, Cu2S CEs are obtained by placing brass sheets in a sulfide solution to form Cu2S layers on the metal surface.225,226 Nevertheless, the problem of mechanical instability is inevitable for these Cu2S CEs when they are used in polysulfide electrolyte. Accordingly, copper chalcogenides (i.e., CuxS and CuxSe) with different stoichiometric forms and various morphologies (Table 3) have been successfully developed to function as effective CE materials in QDSCs with high electrocatalytic activity as well as excellent stability.218,226–244 For example, CuxS films with various stoichiometries were prepared to study the Cu/S ratio effect on the electrocatalytic activity toward polysulfide reduction (Fig. 9A). It was indicated that Cu-deficient films (CuS and Cu1.12S) were superior to Cu-rich films (Cu1.75S and Cu1.8S) as CEs in QDSCs (Fig. 9B and C). Meanwhile, the stability of CuS and Cu1.12S CEs was also higher than that of Cu1.75S and Cu1.8S.236 In addition, many other metal chalcogenides with satisfactory electrocatalytic properties have been fabricated (Table 3), including cobalt sulfides (e.g., CoS,245,246 Co9S8,217 and CoS2
247), iron sulfides (e.g., FeS2,248 and FeS249,250), molybdenum sulfides (e.g., MoS2),251 nickel sulfides (e.g., Ni2S3,252 and NiS253), nickel cobalt sulfides (e.g., NiCo2S4),254 copper zinc tin sulfides/selenides (e.g., Cu2ZnSnS4 (CZTS)255–257 and Cu2ZnSn(S1−xSex)4
258), lead sulfides/selenides (e.g., PbS,259–261 and PbSe242,262), and others (e.g., AgBiS2,263 Bi2S3,264 CuAgSe,265 Cu2SnS3,266 and Cu2SnSe3
218). For example, Cu–CoS porous nanosheet films grown on FTO substrates via a hydrothermal process followed by a cation exchange reaction exhibited excellent electrocatalytic activity toward polysulfide electrolyte with a best PCE of 6.1% and high stability for the resultant QDSCs.246 Besides, combining two kinds of metal chalcogenides, such as CoS/CuS,267 CoS/NiS,268 CuS/NiS,269 CuS/PbS,270 and NiS/PbS,271 is usually adopted to further boost the electrocatalytic performance of CEs in QDSCs. For instance, NiS/PbS composites (4.52%) prepared by the CBD method showed high electrocatalytic activity and stability in the corresponding QDSCs as compared with NiS (3.26%), PbS (3.06%) and Pt (1.29%) CEs (Fig. 9D–F).271
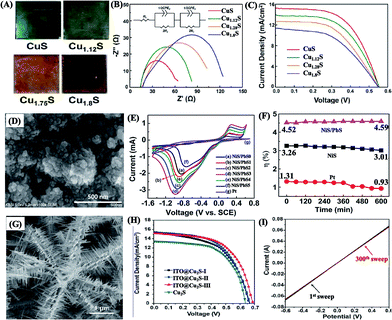 |
| Fig. 9 (A) Photographs and (B) EIS plots of different copper sulfides, and (C) J–V curves of QDSCs with various CuxS CEs (reproduced from ref. 236 with permission from the American Chemical Society). (D) SEM image of NiS/PbS electrode, (E) cyclic voltammetry measurements of NiS/PbS and Pt electrodes in a polysulfide redox couple, and (F) stability test of QDSCs based on different CEs (reproduced from ref. 271 with permission from the Royal Society of Chemistry). (G) SEM image of ITO/Cu2S film, (H) J–V curves of QDSCs and (I) stability test of a symmetrical cell based on ITO/Cu2S CEs (reproduced from ref. 291 with permission from the American Chemical Society). | |
Carbon-based materials are widely applied as CEs both in DCSs and QDSCs because of their high electrical conductivity, great electrocatalytic activity, and excellent thermal and chemical stability.216,272,273 A series of carbon-based materials, including carbon films with various structures (e.g., NTs,219 NFs,274 dots,275 NPs,276 hollow spheres277 and foam278), C60,279 and graphene,280 are well confirmed as highly efficient CEs in QDSCs. For example, Ti mesh supported mesoporous carbon (MC/Ti) with a 3D interconnected framework not only provided high electrocatalytic activity, but also reduced the redox potential of polysulfide electrolyte for a high photovoltage, thereby yielding an unprecedented PCE of 11.16% with a remarkable enhancement of over 26% as compared to Cu2S CEs (8.79%) in QDSCs.157
Composite films usually consisting of two or more components that combine the advantages of each component into one have been widely accepted as effective CEs in QDSCs. Significantly, these composite CEs involve a variety of functional materials, such as novel metals combined with metal sulfides (e.g., Pt/CuS,281 Au/CoS,22 and Au/CuS282), metal sulfides (e.g., CuxS,23,283–288 FeS,289 and PbS290) combined with carbon-based materials, ITO/Cu2S,291 CuS/ZnO,292 PbS/ZnO,293 CNTs/PANI,221 NiS/PANI,294 Mo-compound/CNT/graphene composites,295 and so on. For example, Cu2S nanocrystals were selectively grown on conductive single-crystalline ITO nanowire arrays to assemble 3D hierarchically nanostructured composites (Fig. 9G), which exhibited a superior electrocatalytic performance in QDSCs and thus a highest PCE of 6.12% with a 21.2% improvement as compared with a conventional brass/Cu2S CE (Fig. 9H and I).291
Moreover, other special materials, such as metal nitrides (e.g., NiN,296 and TiN222), metal carbides (e.g., TiC),297 conductive polymers (e.g., poly(3,4-ethylenedioxythiophene) (PEDOT)298 and PANI294), and so on, have also been researched as CEs in QDSCs. For example, Ti-based ceramic materials of TiN and TiC NPs were employed as CEs in CdS QDSCs, and the TiC NPs showed the best efficiency (2.11%) as compared with TiN NPs (1.97%) and Au films (1.64%).297
Conclusion and future prospects
Over the past few years, QDSCs have been the subject of extensive studies for the optimization of all the active materials relating to cell devices. Significantly, the conversion efficiency of QDSCs has greatly improved from less than 1% up to 12% in no more than ten years. In this feature article, we roughly summarize the recent developments of photoanodes, QD sensitizers, electrolytes and CEs in QDSCs. Many attempts have been carried out to obtain effective photoanode materials for fast electron extraction, optimum QDs to maximize light harvesting, stable electrolyte mediums for efficient charge transport, and versatile CE materials with excellent electrocatalytic ability. Remarkably, it is inspiring that the best PCE of QDSCs is now comparable to that of DSCs. However, many challenges still hinder the practical applications of QDSCs, such as achieving higher efficiency, long-term durability, and large-scale production. Therefore, the future big leaps in QDSCs will involve rational strategies to increase the VOC and FF values of cell devices by optimizing the relevant active materials, developing more stable sensitizer layers and electrolytes or hole-transporting materials for solid-state QDSCs, and exploiting some techniques available for large-scale manufacture.
Acknowledgements
The authors gratefully acknowledge the financial support from the National Nature Science Foundation of China (no. 21503177), the Natural Science Foundation of Fujian Province of China (no. 2017J01026), the Fundamental Research Funds for the Central Universities of China (no. 20720150031), and the “111” Project (B16029).
References
- T. R. Cook, D. K. Dogutan, S. Y. Reece, Y. Surendranath, T. S. Teets and D. G. Nocera, Chem. Rev., 2010, 110, 6474–6502 CrossRef CAS PubMed.
- N. S. Lewis, Science, 2007, 315, 798–801 CrossRef CAS PubMed.
- V. Gonzaález-Pedro, X. Xu, I. Mora-Seroó and J. Bisquert, ACS Nano, 2010, 4, 5783 CrossRef PubMed.
- M. A. Hossain, J. R. Jennings, C. Shen, J. H. Pan, Z. Y. Koh, N. Mathews and Q. Wang, J. Mater. Chem., 2012, 22, 16235–16242 RSC.
- P. V. Kamat, J. Phys. Chem. C, 2008, 112, 18737–18753 CAS.
- W. Ma, S. L. Swisher, T. Ewers, J. Engel, V. E. Ferry, H. A. Atwater and A. P. Alivisatos, ACS Nnano, 2011, 5, 8140–8147 CrossRef CAS PubMed.
- D. Segets, J. M. Lucas, R. N. K. Taylor, M. Scheele, H. Zheng, A. P. Alivisatos and W. Peukert, ACS Nnano, 2012, 6, 9021–9032 CrossRef CAS PubMed.
- I. Hod and A. Zaban, Langmuir, 2014, 30, 7264–7273 CrossRef CAS PubMed.
- I. Mora-Sero, S. Gimenez, F. Fabregat-Santiago, R. Gomez, Q. Shen, T. Toyoda and J. Bisquert, Acc. Chem. Res., 2009, 42, 1848–1857 CrossRef CAS PubMed.
- I. Hwang and K. Yong, ChemElectroChem, 2015, 2, 634–653 CrossRef CAS.
- X. Xin, B. Li, J. Jung, Y. J. Yoon, R. Biswas and Z. Lin, Part. Part. Syst. Charact., 2015, 32, 80–90 CrossRef CAS.
- D. Zheng, X. Pang, M. Wang, Y. He, C. Lin and Z. Lin, Chem. Mater., 2015, 27, 5271–5278 CrossRef CAS.
- J. Tian, Q. Zhang, E. Uchaker, R. Gao, X. Qu, S. Zhang and G. Cao, Energy Environ. Sci., 2013, 6, 3542–3547 CAS.
- X. Du, X. He, L. Zhao, H. Chen, W. Li, W. Fang, W. Zhang, J. Wang and H. Chen, J. Power Sources, 2016, 332, 1–7 CrossRef CAS.
- Y.-F. Xu, W.-Q. Wu, H.-S. Rao, H.-Y. Chen, D.-B. Kuang and C.-Y. Su, Nano Energy, 2015, 11, 621–630 CrossRef CAS.
- J.-Y. Kim, J. Yang, J. H. Yu, W. Baek, C.-H. Lee, H. J. Son, T. Hyeon and M. J. Ko, ACS Nnano, 2015, 9, 11286–11295 CrossRef CAS PubMed.
- T.-L. Li, Y.-L. Lee and H. Teng, Energy Environ. Sci., 2012, 5, 5315–5324 CAS.
- J. Du, Z. Du, J.-S. Hu, Z. Pan, Q. Shen, J. Sung, D. Long, H. Dong, L. Sun, X. Zhong and L.-J. Wan, J. Am. Chem. Soc., 2016, 138, 4201–4209 CrossRef CAS PubMed.
- J. Du, X. Meng, K. Zhao, Y. Li and X. Zhong, J. Mater. Chem. A, 2015, 3, 17091–17097 CAS.
- V. Chakrapani, D. Baker and P. V. Kamat, J.
Am. Chem. Soc., 2011, 133, 9607–9615 CrossRef CAS PubMed.
- Y. Liao, J. Zhang, W. Liu, W. Que, X. Yin, D. Zhang, L. Tang, W. He, Z. Zhong and H. Zhang, Nano Energy, 2015, 11, 88–95 CrossRef CAS.
- W. Guo, X. Zhang, R. Yu, M. Que, Z. Zhang, Z. Wang, Q. Hua, C. Wang, Z. L. Wang and C. Pan, Adv. Energy Mater., 2015, 5, 1500141 CrossRef.
- M. Ye, C. Chen, N. Zhang, X. Wen, W. Guo and C. Lin, Adv. Energy Mater., 2014, 4, 1301564 CrossRef.
-
https://www.nrel.gov/pv/assets/images/efficiency-chart.png
.
- M. Wu, X. Lin, Y. Wang and T. Ma, J. Mater. Chem. A, 2015, 3, 19638–19656 CAS.
- K. Meng, G. Chen and K. R. Thampi, J. Mater. Chem. A, 2015, 3, 23074–23089 CAS.
- R. S. Selinsky, Q. Ding, M. S. Faber, J. C. Wright and S. Jin, Chem. Soc. Rev., 2013, 42, 2963–2985 RSC.
- H. K. Jun, M. A. Careem and A. K. Arof, Renewable Sustainable Energy Rev., 2013, 22, 148–167 CrossRef CAS.
- I. J. Kramer and E. H. Sargent, Chem. Rev., 2014, 114, 863–882 CrossRef CAS PubMed.
- J. Tian and G. Cao, J. Phys. Chem. Lett., 2015, 6, 1859–1869 CrossRef CAS PubMed.
- P. V. Kamat, Acc. Chem. Res., 2012, 45, 1906–1915 CrossRef CAS PubMed.
- J. Tian, E. Uchaker, Q. Zhang and G. Cao, ACS Appl. Mater. Interfaces, 2014, 6, 4466–4472 CAS.
- M. Seol, H. Kim, Y. Tak and K. Yong, Chem. Commun., 2010, 46, 5521–5523 RSC.
- H.-L. Feng, W.-Q. Wu, H.-S. Rao, L.-B. Li, D.-B. Kuang and C.-Y. Su, J. Mater. Chem. A, 2015, 3, 14826–14832 CAS.
- W. Zhang, X. Zeng, H. Wang, R. Fang, Y. Xu, Y. Zhang and W. Chen, RSC Adv., 2016, 6, 33713–33722 RSC.
- Z. Du, H. Zhang, H. Bao and X. Zhong, J. Mater. Chem. A, 2014, 2, 13033–13040 CAS.
- J. Jean, S. Chang, P. R. Brown, J. J. Cheng, P. H. Rekemeyer, M. G. Bawendi, S. Gradecak and V. Bulovic, Adv. Mater., 2013, 25, 2790–2796 CrossRef CAS PubMed.
- P. H. Rekemeyer, S. Chang, C.-H. M. Chuang, G. W. Hwang, M. G. Bawendi and S. Gradecak, Adv. Energy Mater., 2016, 6, 1600848 CrossRef.
- H.-L. Feng, W.-Q. Wu, H.-S. Rao, Q. Wan, L.-B. Li, D.-B. Kuang and C.-Y. Su, ACS Appl. Mater. Interfaces, 2015, 7, 5199–5205 CAS.
- J. Tian, Q. Zhang, L. Zhang, R. Gao, L. Shen, S. Zhang, X. Qu and G. Cao, Nanoscale, 2013, 5, 936–943 RSC.
- J. Dong, Y. Zhu, S. Jia and Z. Zhu, RSC Adv., 2016, 6, 14224–14228 RSC.
- H.-M. Cheng, K.-Y. Huang, K.-M. Lee, P. Yu, S.-C. Lin, J.-H. Huang, C.-G. Wu and J. Tang, Phys. Chem. Chem. Phys., 2012, 14, 13539–13548 RSC.
- D. Wu, J. He, S. Zhang, K. Cao, Z. Gao, F. Xu and K. Jiang, J. Power Sources, 2015, 282, 202–210 CrossRef CAS.
- C. Cui, Y. Qiu, J. Zhao, B. Lu, H. Hu, Y. Yang, N. Ma, S. Xu, L. Xu and X. Li, Electrochim. Acta, 2015, 173, 551–558 CrossRef CAS.
- C. Li, L. Yang, J. Xiao, Y.-C. Wu, M. Sondergaard, Y. Luo, D. Li, Q. Meng and B. B. Iversen, Phys. Chem. Chem. Phys., 2013, 15, 8710–8715 RSC.
- B. B. Jin, Y. F. Wang and J. H. Zeng, Chem. Phys. Lett., 2016, 660, 76–80 CrossRef CAS.
- F. Zhao, G. Tang, J. Zhang and Y. Lin, Electrochim. Acta, 2012, 62, 396–401 CrossRef CAS.
- J. Wan, R. Liu, Y. Tong, S. Chen, Y. Hu, B. Wang, Y. Xu and H. Wang, Nanoscale Res. Lett., 2016, 11, 12 CrossRef PubMed.
- G. Zhu, Z. Cheng, T. Lv, L. Pan, Q. Zhao and Z. Sun, Nanoscale, 2010, 2, 1229–1232 RSC.
- L. Jiang, T. You and W.-Q. Deng, Nanotechnology, 2013, 24, 415401 CrossRef PubMed.
- L. Li, X. Yang, W. Zhang, H. Zhang and X. Li, J. Power Sources, 2014, 272, 508–512 CrossRef CAS.
- H. Zhao, F. Huang, J. Hou, Z. Liu, Q. Wu, H. Cao, Q. Jing, S. Peng and G. Cao, ACS Appl. Mater. Interfaces, 2016, 8, 26675–26682 CAS.
- T. Kawawaki and T. Tatsuma, Phys. Chem. Chem. Phys., 2013, 15, 20247–20251 RSC.
- T. R. Chetia, M. S. Ansari and M. Qureshi, J. Mater. Chem. A, 2016, 4, 5528–5541 CAS.
- J. Chen, C. Li, G. Eda, Y. Zhang, W. Lei, M. Chhowalla, W. I. Milne and W.-Q. Deng, Chem. Commun., 2011, 47, 6084–6086 RSC.
- M. R. Golobostanfard, H. Abdizadeh and S. Mohajerzadeh, Nanotechnology, 2014, 25, 345402 CrossRef PubMed.
- D. Punnoose, C. S. S. P. Kumar, H. W. Seo, M. Shiratani, A. E. Reddy, S. S. Rao, C. V. Thulasi-Varma, S.-K. Kim, S.-H. Chung and H.-J. Kim, New J. Chem., 2016, 40, 3423–3431 RSC.
- K. Wang, J. Jiang, S. Wan and J. Zhai, Electrochim. Acta, 2015, 155, 357–363 CrossRef CAS.
- H. Sun, L. Pan, G. Zhu, X. Piao, L. Zhang and Z. Sun, Dalton Trans., 2014, 43, 14936–14941 RSC.
- H. Zhou, L. Li, D. Jiang, Y. Lu and K. Pan, RSC Adv., 2016, 6, 67968–67975 RSC.
- J. Tian, L. Lv, X. Wang, C. Fei, X. Liu, Z. Zhao, Y. Wang and G. Cao, J. Phys. Chem. C, 2014, 118, 16611–16617 CAS.
- C. Wang, Z. Jiang, L. Wei, Y. Chen, J. Jiao, M. Eastman and H. Liu, Nano Energy, 2012, 1, 440–447 CrossRef CAS.
- C. Chen, M. Ye, M. Lv, C. Gong, W. Guo and C. Lin, Electrochim. Acta, 2014, 121, 175–182 CrossRef CAS.
- J. Tian, Q. Zhang, E. Uchaker, Z. Liang, R. Gao, X. Qu, S. Zhang and G. Cao, J. Mater. Chem. A, 2013, 1, 6770–6775 CAS.
- X.-J. Shi, Y. Wang, D. Wu, Y. Qin, X.-C. Ai, D. Xu and J.-P. Zhang, RSC Adv., 2015, 5, 32110–32117 RSC.
- D. Wu, X. Shi, H. Dong, F. Zhu, K. Jiang, D. Xu, X. Ai and J. Zhang, J. Mater. Chem. A, 2014, 2, 16276–16284 CAS.
- Y. Lin, Y. Lin, J. Wu, Y. Tu, X. Zhang and B. Fang, Ceram. Int., 2015, 41, 14501–14507 CrossRef CAS.
- H. Huang, L. Pan, C. K. Lim, H. Gong, J. Guo, M. S. Tse and O. K. Tan, Small, 2013, 9, 3153–3160 CrossRef CAS PubMed.
- Q. Zhang, G. Chen, Y. Yang, X. Shen, Y. Zhang, C. Li, R. Yu, Y. Luo, D. Li and Q. Meng, Phys. Chem. Chem. Phys., 2012, 14, 6479–6486 RSC.
- S. Yang, A. S. Nair and S. Ramakrishna, Mater. Lett., 2014, 116, 345–348 CrossRef CAS.
- M. Samadpour, S. Gimenez, A. I. Zad, N. Taghavinia and I. Mora-Sero, Phys. Chem. Chem. Phys., 2012, 14, 522–528 RSC.
- R. Zhou, Q. Zhang, E. Uchaker, J. Lan, M. Yin and G. Cao, J. Mater. Chem. A, 2014, 2, 2517–2525 CAS.
- Z. Zhu, J. Qiu, K. Yan and S. Yang, ACS Appl. Mater. Interfaces, 2013, 5, 4000–4005 CAS.
- T. R. Chetia, M. S. Ansari and M. Qureshi, ACS Appl. Mater. Interfaces, 2015, 7, 13266–13279 CAS.
- B. Liu, Y. Sun, X. Wang, L. Zhang, D. Wang, Z. Fu, Y. Lin and T. Xie, J. Mater. Chem. A, 2015, 3, 4445–4452 CAS.
- F. Zhu, H. Dong, Y. Wang, D. Wu, J. Li, J. Pan, Q. Li, X. Ai, J. Zhang and D. Xu, Phys. Chem. Chem. Phys., 2013, 15, 17798–17803 RSC.
- H.-S. Rao, W.-Q. Wu, Y. Liu, Y.-F. Xu, B.-X. Chen, H.-Y. Chen, D.-B. Kuang and C.-Y. Su, Nano Energy, 2014, 8, 1–8 CrossRef CAS.
- H. Han, P. Sudhagar, T. Song, Y. Jeon, I. Mora-Sero, F. Fabregat-Santiago, J. Bisquert, Y. S. Kang and U. Paik, Chem. Commun., 2013, 49, 2810–2812 RSC.
- H. Kim and K. Yong, Phys. Chem. Chem. Phys., 2013, 15, 2109–2116 RSC.
- Y. Cao, Y.-J. Dong, H.-Y. Chen, D.-B. Kuang and C.-Y. Su, RSC Adv., 2016, 6, 78202–78209 RSC.
- E.-H. Kong, Y.-J. Chang, Y.-C. Park, Y.-H. Yoon, H.-J. Park and H. M. Jang, Phys. Chem. Chem. Phys., 2012, 14, 4620–4625 RSC.
- T. R. Chetia, D. Barpuzary and M. Qureshi, Phys. Chem. Chem. Phys., 2014, 16, 9625–9633 RSC.
- T. Shu, P. Xiang, Z.-M. Zhou, H. Wang, G.-H. Liu, H.-W. Han and Y.-D. Zhao, Electrochim. Acta, 2012, 68, 166–171 CrossRef CAS.
- S.-K. Kim, C. V. V. M. Gopi, S. S. Rao, D. Punnoose and H.-J. Kim, Appl. Surf. Sci., 2016, 365, 136–142 CrossRef CAS.
- R. Zhang, B. Zhang, Y. Liu and S. Sun, J. Mater. Chem. C, 2016, 4, 1633–1644 RSC.
- P. R. Brown, R. R. Lunt, N. Zhao, T. P. Osedach, D. D. Wanger, L.-Y. Chang, M. G. Bawendi and V. Bulovic, Nano Lett., 2011, 11, 2955–2961 CrossRef CAS PubMed.
- S.-K. Kim, M.-K. Son, S. Park, M.-S. Jeong, K. Prabakar and H.-J. Kim, Electrochim. Acta, 2014, 118, 118–123 CrossRef CAS.
- L.-B. Li, Y.-F. Wang, H.-S. Rao, W.-Q. Wu, K.-N. Li, C.-Y. Su and D.-B. Kuang, ACS Appl. Mater. Interfaces, 2013, 5, 11865–11871 CAS.
- K. Meng, P. K. Surolia and K. R. Thampi, J. Mater. Chem. A, 2014, 2, 10231–10238 CAS.
- S. Zhou and L. Yin, J. Alloys Compd., 2017, 691, 1040–1048 CrossRef CAS.
- J. Park, M. T. Sajjad, P.-H. Jouneau, A. Ruseckas, J. Faure-Vincent, I. D. W. Samuel, P. Reiss and D. Aldakov, J. Mater. Chem. A, 2016, 4, 827–837 CAS.
- Y.-Q. Mao, Z.-J. Zhou, T. Ling and X.-W. Du, RSC Adv., 2013, 3, 1217–1221 RSC.
- Z. Lan, L. Liu, M. Huang, J. Wu and J. Lin, J. Mater. Sci.: Mater. Electron., 2015, 26, 7914–7920 CrossRef CAS.
- C. Chen, Q. Dai, C. Miao, L. Xu and H. Song, RSC Adv., 2015, 5, 4844–4852 RSC.
- L. Lu, J. Chen and W. Wang, Appl. Phys. Lett., 2013, 103, 123902 CrossRef.
- A. Pimachev, U. Poudyal, V. Proshchenko, W. Wang and Y. Dahnovsky, Phys. Chem. Chem. Phys., 2016, 18, 26771–26776 RSC.
- T. Bora, H. H. Kyaw and J. Dutta, Electrochim. Acta, 2012, 68, 141–145 CrossRef CAS.
- M. M. Jaculine, S. J. Das, H.-J. Kim, B. C. Kim, K.-H. Yu and C. J. Raj, Mater. Lett., 2013, 111, 28–31 CrossRef CAS.
- X. Lan, O. Voznyy, F. P. G. de Arquer, M. Liu, J. Xu, A. H. Proppe, G. Walters, F. Fan, H. Tan, M. Liu, Z. Yang, S. Hoogland and E. H. Sargent, Nano Lett., 2016, 16, 4630–4634 CrossRef CAS PubMed.
- S. Greenwald, S. Ruehle, M. Shalom, S. Yahav and A. Zaban, Phys. Chem. Chem. Phys., 2011, 13, 19302–19306 RSC.
- V. Ganapathy, E.-H. Kong, Y.-C. Park, H. M. Jang and S.-W. Rhee, Nanoscale, 2014, 6, 3296–3301 RSC.
- W. Kong, S. Li, Z. Chen, C. Wei, W. Li, T. Li, Y. Yan, X. Jia, B. Xu and W. Zhang, Part. Part. Syst. Charact., 2015, 32, 1078–1082 CrossRef CAS.
- M. Raissi, Y. Pellegrin, S. Jobic, M. Boujtita and F. Odobel, Sci. Rep., 2016, 6, 24908 CrossRef PubMed.
- X. Li, R. Chen, H. Sui, X. Yuan, M. Li, K. Yang, L. Li, W. Zhang and Y. Zhang, Mater. Res. Bull., 2016, 84, 212–217 CrossRef CAS.
- J. Tian and G. Cao, Nano Rev., 2013, 4, 22578 CrossRef PubMed.
- M. Kouhnavard, S. Ikeda, N. A. Ludin, N. B. A. Khairudin, B. V. Ghaffari, M. A. Mat-Teridi, M. A. Ibrahim, S. Sepeai and K. Sopian, Renewable Sustainable Energy Rev., 2014, 37, 397–407 CrossRef CAS.
- A. Tubtimtae, M.-W. Lee and G.-J. Wang, J. Power Sources, 2011, 196, 6603–6608 CrossRef CAS.
- D. Esparza, I. Zarazua, T. Lopez-Luke, R. Carriles, A. Torres-Castro and E. De la Rosa, Electrochim. Acta, 2015, 180, 486–492 CrossRef CAS.
- L. Mu, C. Liu, J. Jia, X. Zhou and Y. Lin, J. Mater. Chem. A, 2013, 1, 8353–8357 CAS.
- Y. Choi, M. Seol, W. Kim and K. Yong, J. Phys. Chem. C, 2014, 118, 5664–5670 CAS.
- J. Yang and X. Zhong, J. Mater. Chem. A, 2016, 4, 16553–16561 CAS.
- Z. Pan, I. Mora-Sero, Q. Shen, H. Zhang, Y. Li, K. Zhao, J. Wang, X. Zhong and J. Bisquert, J. Am. Chem. Soc., 2014, 136, 9203–9210 CrossRef CAS PubMed.
- J.-Y. Chang, C.-H. Li, Y.-H. Chiang, C.-H. Chen and P.-N. Li, ACS Appl. Mater. Interfaces, 2016, 8, 18878–18890 CAS.
- B. Bai, D. Kou, W. Zhou, Z. Zhou, Q. Tian, Y. Meng and S. Wu, J. Power Sources, 2016, 318, 35–40 CrossRef CAS.
- S. H. Lee, C. Jung, Y. Jun and S. W. Kim, Opt. Mater., 2015, 49, 230–234 CrossRef CAS.
- J. Duan, Q. Tang, B. He and L. Yu, Electrochim. Acta, 2014, 139, 381–385 CrossRef CAS.
- S. Yang, P. Zhao, X. Zhao, L. Qu and X. Lai, J. Mater. Chem. A, 2015, 3, 21922–21929 CAS.
- V. Gonzalez-Pedro, C. Sima, G. Marzari, P. P. Boix, S. Gimenez, Q. Shen, T. Dittrich and I. Mora-Sero, Phys. Chem. Chem. Phys., 2013, 15, 13835–13843 RSC.
- L.-Y. Chang, R. R. Lunt, P. R. Brown, V. Bulovic and M. G. Bawendi, Nano Lett., 2013, 13, 994–999 CrossRef CAS PubMed.
- N. P. Benehkohal, V. Gonzalez-Pedro, P. P. Boix, S. Chavhan, R. Tena-Zaera, G. P. Demopoulos and I. Mora-Sero, J. Phys. Chem. C, 2012, 116, 16391–16397 Search PubMed.
- H. Seo, Y. Wang, G. Uchida, K. Kamataki, N. Itagaki, K. Koga and M. Shiratani, Electrochim. Acta, 2013, 95, 43–47 CrossRef CAS.
- Y. Zhong, H. Zhang, D. Pan, L. Wang and X. Zhong, J. Energy Chem., 2015, 24, 722–728 CrossRef.
- J.-H. Im, C.-R. Lee, J.-W. Lee, S.-W. Park and N.-G. Park, Nanoscale, 2011, 3, 4088–4093 RSC.
- V. Gonzalez-Pedro, X. Xu, I. Mora-Sero and J. Bisquert, ACS Nnano, 2010, 4, 5783–5790 CrossRef CAS PubMed.
- Z. Pan, K. Zhao, J. Wang, H. Zhang, Y. Feng and X. Zhong, ACS Nnano, 2013, 7, 5215–5222 CrossRef CAS PubMed.
- Y.-L. Lee and Y.-S. Lo, Adv. Funct. Mater., 2009, 19, 604–609 CrossRef.
- Y. Zhang, J. Zhu, X. Yu, J. Wei, L. Hu and S. Dai, Sol. Energy, 2012, 86, 964–971 CrossRef CAS.
- Z. Pan, H. Zhang, K. Cheng, Y. Hou, J. Hua and X. Zhong, ACS Nnano, 2012, 6, 3982–3991 CrossRef CAS PubMed.
- H. J. Yun, T. Paik, B. Diroll, M. E. Edley, J. B. Baxter and C. B. Murray, ACS Appl. Mater. Interfaces, 2016, 8, 14692–14700 CAS.
- M. Shalom, S. Buhbut, S. Tirosh and A. Zaban, J. Phys. Chem. Lett., 2012, 3, 2436–2441 CrossRef CAS PubMed.
- N. Guijarro, T. Lana-Villarreal, I. Mora-Sero, J. Bisquert and R. Gomez, J. Phys. Chem. C, 2009, 113, 4208–4214 CAS.
- P. K. Santra and P. V. Kamat, J. Am. Chem. Soc., 2012, 134, 2508–2511 CrossRef CAS PubMed.
- Q. Zhang, J. Cao and H. Li, RSC Adv., 2015, 5, 107957–107963 RSC.
- R. Zhou, Q. Zhang, J. Tian, D. Myers, M. Yin and G. Cao, J. Phys. Chem. C, 2013, 117, 26948–26956 CAS.
- I. P. Liu, C.-W. Chang, H. Teng and Y.-L. Lee, ACS Appl. Mater. Interfaces, 2014, 6, 19378–19384 CAS.
- X.-Y. Yu, J.-Y. Liao, K.-Q. Qiu, D.-B. Kuang and C.-Y. Su, ACS Nnano, 2011, 5, 9494–9500 CrossRef CAS PubMed.
- Q. Wang, S. Li, J. Qiao, R. Jin, Y. Yu and S. Gao, Sol. Energy Mater. Sol. Cells, 2015, 132, 650–654 CrossRef CAS.
- J. H. Bang and P. V. Kamat, ACS Nnano, 2009, 3, 1467–1476 CrossRef CAS PubMed.
- J. Wang, I. Mora-Sero, Z. Pan, K. Zhao, H. Zhang, Y. Feng, G. Yang, X. Zhong and J. Bisquert, J. Am. Chem. Soc., 2013, 135, 15913–15922 CrossRef CAS PubMed.
- D. Yuan, L. Xiao, J. Luo, Y. Luo, Q. Meng, B.-W. Mao and D. Zhan, ACS Appl. Mater. Interfaces, 2016, 8, 18150–18156 CAS.
- S. Jiao, J. Wang, Q. Shen, Y. Li and X. Zhong, J. Mater. Chem. A, 2016, 4, 7214–7221 CAS.
- N. Zhou, Y. Yang, X. Huang, H. Wu, Y. Luo, D. Li and Q. Meng, Chemsuschem, 2013, 6, 687–692 CrossRef CAS PubMed.
- A. Sahasrabudhe and S. Bhattacharyya, Chem. Mater., 2015, 27, 4848–4859 CrossRef CAS.
- Y.-q. Wang, Y.-c. Rui, Q.-h. Zhang, Y.-g. Li and H.-z. Wang, ACS Appl. Mater. Interfaces, 2013, 5, 11858–11864 CAS.
- M. A. Basit, M. A. Abbas, E. S. Jung, Y. M. Park, J. H. Bang and T. J. Park, Electrochim. Acta, 2016, 211, 644–651 CrossRef.
- S. Jiao, Q. Shen, I. Mora-Sero, J. Wang, Z. Pan, K. Zhao, Y. Kuga, X. Zhong and J. Bisquert, ACS Nnano, 2015, 9, 908–915 CrossRef CAS PubMed.
- R. Zhou, L. Wan, H. Niu, L. Yang, X. Mao, Q. Zhang, S. Miao, J. Xu and G. Cao, Sol. Energy Mater. Sol. Cells, 2016, 155, 20–29 CrossRef CAS.
- Z. Chen, W. Peng, K. Zhang, J. Zhang, X. Yang, Y. Numata and L. Han, J. Mater. Chem. A, 2014, 2, 7004–7014 CAS.
- J. Luo, H. Wei, F. Li, Q. Huang, D. Li, Y. Luo and Q. Meng, Chem. Commun., 2014, 50, 3464–3466 RSC.
- J. Yang, T. Oshima, W. Yindeesuk, Z. Pan, X. Zhong and Q. Shen, J. Mater. Chem. A, 2014, 2, 20882–20888 CAS.
- H. McDaniel, N. Fuke, N. S. Makarov, J. M. Pietryga and V. I. Klimov, Nat. Commun., 2013, 4, 2887 Search PubMed.
- W. Wang, J. Du, Z. Ren, W. Peng, Z. Pan and X. Zhong, ACS Appl. Mater. Interfaces, 2016, 8, 31006–31015 CAS.
- H. Wei, G. Wang, Y. Luo, D. Li and Q. Meng, Electrochim. Acta, 2015, 173, 156–163 CrossRef CAS.
- G. Wang, H. Wei, Y. Luo, H. Wu, D. Li, X. Zhong and Q. Meng, J. Power Sources, 2016, 302, 266–273 CrossRef CAS.
- J. Yang, J. Wang, K. Zhao, T. Izuishi, Y. Li, Q. Shen and X. Zhong, J. Phys. Chem. C, 2015, 119, 28800–28808 CAS.
- J. Wang, Y. Li, Q. Shen, T. Izuishi, Z. Pan, K. Zhao and X. Zhong, J. Mater. Chem. A, 2016, 4, 877–886 CAS.
- Z. Du, Z. Pan, F. Fabregat-Santiago, K. Zhao, D. Long, H. Zhang, Y. Zhao, X. Zhong, J.-S. Yu and J. Bisquert, J. Phys. Chem. Lett., 2016, 7, 3103–3111 CrossRef CAS PubMed.
- S. W. Jung, J.-H. Kim, H. Kim, C.-J. Choi and K.-S. Ahn, J. Appl. Phys., 2011, 110, 044313 CrossRef.
- G. Zhu, L. Pan, T. Xu and Z. Sun, ACS Appl. Mater. Interfaces, 2011, 3, 1472–1478 CAS.
- G. Zhu, L. Pan, T. Xu and Z. Sun, ACS Appl. Mater. Interfaces, 2011, 3, 3146–3151 CAS.
- L. Tao, Y. Xiong, H. Liu and W. Shen, Nanoscale, 2014, 6, 931–938 RSC.
- X.-Y. Yu, B.-X. Lei, D.-B. Kuang and C.-Y. Su, J. Mater. Chem., 2012, 22, 12058–12063 RSC.
- K. Yan, W. Chen and S. Yang, J. Phys. Chem. C, 2013, 117, 92–99 CAS.
- S. S. Mali, H. Kim, P. S. Patil and C. K. Hong, Dalton Trans., 2013, 42, 16961–16967 RSC.
- R. Zhou, H. Niu, Q. Zhang, E. Uchaker, Z. Guo, L. Wan, S. Miao, J. Xu and G. Cao, J. Mater. Chem. A, 2015, 3, 12539–12549 CAS.
- F. Huang, J. Hou, Q. Zhang, Y. Wang, R. C. Masse, S. Peng, H. Wang, J. Liu and G. Cao, Nano Energy, 2016, 26, 114–122 CrossRef CAS.
- Y. Liu, Z. Li, L. Yu and S. Sun, J. Nanopart. Res., 2015, 17, 132 CrossRef.
- K. E. Roelofs, S. M. Herron and S. F. Bent, ACS Nnano, 2015, 9, 8321–8334 CrossRef CAS PubMed.
- I. P. Liu, L.-Y. Chen and Y.-L. Lee, J. Power Sources, 2016, 325, 706–713 CrossRef CAS.
- B. Gao, C. Shen, S. Yuan, B. Zhang, M. Zhang, Y. Yang and G. Chen, J. Alloys Compd., 2014, 612, 323–329 CrossRef CAS.
- F. Liu, J. Zhu, J. Wei, Y. Li, L. Hu, Y. Huang, O. Takuya, Q. Shen, T. Toyoda, B. Zhang, J. Yao and S. Dai, J. Phys. Chem. C, 2014, 118, 214–222 CAS.
- A. N. Jumabekov, T. D. Siegler, N. Cordes, D. D. Medina, D. Boehm, P. Garbus, S. Meroni, L. M. Peter and T. Bein, J. Phys. Chem. C, 2014, 118, 25853–25862 CAS.
- H. J. Yun, T. Paik, M. E. Edley, J. B. Baxter and C. B. Murray, ACS Appl. Mater. Interfaces, 2014, 6, 3721–3728 CAS.
- H. Zhang, K. Cheng, Y. M. Hou, Z. Fang, Z. X. Pan, W. J. Wu, J. L. Hua and X. H. Zhong, Chem. Commun., 2012, 48, 11235–11237 RSC.
- Q. Dai, E. M. Sabio, W. Wang and J. Tang, Appl. Phys. Lett., 2014, 104, 183901 CrossRef.
- G. Zhu, T. Lv, L. Pan, Z. Sun and C. Sun, J. Alloys Compd., 2011, 509, 362–365 CrossRef CAS.
- A. Salant, M. Shalom, I. Hod, A. Faust, A. Zaban and U. Banin, ACS Nnano, 2010, 4, 5962–5968 CrossRef CAS PubMed.
- C. V. V. M. Gopi, M. Venkata-Haritha, H. Seo, S. Singh, S.-K. Kim, M. Shiratani and H.-J. Kim, Dalton Trans., 2016, 45, 8447–8457 RSC.
- N. Firoozi, H. Dehghani and M. Afrooz, J. Power Sources, 2015, 278, 98–103 CrossRef CAS.
- M. P. A. Muthalif, Y.-S. Lee, C. D. Sunesh, H.-J. Kim and Y. Choe, Appl. Surf. Sci., 2016, 25, 2724–2729 Search PubMed.
- T. Shen, J. Tian, L. Lv, C. Fei, Y. Wang, T. Pullerits and G. Cao, Electrochim. Acta, 2016, 191, 62–69 CrossRef CAS.
- M. Venkata-Haritha, C. V. V. M. Gopi, C. V. Thulasi-Varma, S.-K. Kim and H.-J. Kim, J. Photochem. Photobiol., A, 2016, 315, 34–41 CrossRef CAS.
- H.-J. Kim, H.-D. Lee, C. S. S. P. Kumar, S. S. Rao, S.-H. Chung and D. Punnoose, New J. Chem., 2015, 39, 4805–4813 RSC.
- F. Huang, Q. Zhang, B. Xu, J. Hou, Y. Wang, R. C. Masse, S. Peng, J. Liu and G. Cao, J. Mater. Chem. A, 2016, 4, 14773–14780 CAS.
- C. V. V. M. Gopi, M. Venkata-Haritha, S.-K. Kim and H.-J. Kim, Nanoscale, 2015, 7, 12552–12563 RSC.
- T. Shen, L. Bian, B. Li, K. Zheng, T. Pullerits and J. Tian, Appl. Phys. Lett., 2016, 108, 213901 CrossRef PubMed.
- K. Yu, X. Lin, G. Lu, Z. Wen, C. Yuan and J. Chen, RSC Adv., 2012, 2, 7843–7848 RSC.
- M. Zeng, X. Peng, J. Liao, G. Wang, Y. Li, J. Li, Y. Qin, J. Wilson, A. Song and S. Lin, Phys. Chem. Chem. Phys., 2016, 18, 17404–17413 RSC.
- K. Zhao, Z. Pan, I. Mora-Sero, E. Canovas, H. Wang, Y. Song, X. Gong, J. Wang, M. Bonn, J. Bisquert and X. Zhong, J. Am. Chem. Soc., 2015, 137, 5602–5609 CrossRef CAS PubMed.
- J. Duan, H. Zhang, Q. Tang, B. He and L. Yu, J. Mater. Chem. A, 2015, 3, 17497–17510 CAS.
- H. Wei, G. Wang, J. Shi, H. Wu, Y. Luo, D. Li and Q. Meng, J. Mater.
Chem. A, 2016, 4, 14194–14203 CAS.
- G. Jiang, Z. Pan, Z. Ren, J. Du, C. Yang, W. Wang and X. Zhong, J. Mater. Chem. A, 2016, 4, 11416–11421 CAS.
- C.-Y. Chou, C.-P. Lee, R. Vittal and K.-C. Ho, J. Power Sources, 2011, 196, 6595–6602 CrossRef CAS.
- L. Li, X. Yang, J. Gao, H. Tian, J. Zhao, A. Hagfeldt and L. Sun, J. Am. Chem. Soc., 2011, 133, 8458–8460 CrossRef CAS PubMed.
- Z. Ning, C. Yuan, H. Tian, Y. Fu, L. Li, L. Sun and H. Agren, J. Mater. Chem., 2012, 22, 6032–6037 RSC.
- K. Meng, P. K. Surolia, O. Byrne and K. R. Thampi, J. Power Sources, 2015, 275, 681–687 CrossRef CAS.
- V. Jovanovski, V. Gonzalez-Pedro, S. Gimenez, E. Azaceta, G. Cabanero, H. Grande, R. Tena-Zaera, I. Mora-Sero and J. Bisquert, J. Am. Chem. Soc., 2011, 133, 20156–20159 CrossRef CAS PubMed.
- W. Feng, Y. Li, J. Du, W. Wang and X. Zhong, J. Mater. Chem. A, 2016, 4, 1461–1468 CAS.
- K. Meng and K. R. Thampi, ACS Appl. Mater. Interfaces, 2014, 6, 20768–20775 CAS.
- D. Karageorgopoulos, E. Stathatos and E. Vitoratos, J. Power Sources, 2012, 219, 9–15 CrossRef CAS.
- S. Wang, Q.-X. Zhang, Y.-Z. Xu, D.-M. Li, Y.-H. Luo and Q.-B. Meng, J. Power Sources, 2013, 224, 152–157 CrossRef CAS.
- H. Kim, I. Hwang and K. Yong, ACS Appl. Mater. Interfaces, 2014, 6, 11245–11253 CAS.
- Z. Huo, L. Tao, S. Wang, J. Wei, J. Zhu, W. Dong, F. Liu, S. Chen, B. Zhang and S. Dai, J. Power Sources, 2015, 284, 582–587 CrossRef CAS.
- Z. Yu, Q. Zhang, D. Qin, Y. Luo, D. Li, Q. Shen, T. Toyoda and Q. Meng, Electrochem. Commun., 2010, 12, 1776–1779 CrossRef CAS.
- W. Feng, L. Zhao, J. Du, Y. Li and X. Zhong, J. Mater. Chem. A, 2016, 4, 14849–14856 CAS.
- K. E. Roelofs, T. P. Brennan, J. C. Dominguez, C. D. Bailie, G. Y. Margulis, E. T. Hoke, M. D. McGehee and S. F. Bent, J. Phys. Chem. C, 2013, 117, 5584–5592 CAS.
- A. N. Jumabekov, N. Cordes, T. D. Siegler, P. Docampo, A. Ivanova, K. Fominykh, D. D. Medina, L. M. Peter and T. Bein, ACS Appl. Mater. Interfaces, 2016, 8, 4600–4607 CAS.
- H. Lee, H. C. Leventis, S.-J. Moon, P. Chen, S. Ito, S. A. Haque, T. Torres, F. Nueesch, T. Geiger, S. M. Zakeeruddin, M. Graetzel and M. K. Nazeeruddin, Adv. Funct. Mater., 2009, 19, 2735–2742 CrossRef CAS.
- T. P. Brennan, P. Ardalan, H.-B.-R. Lee, J. R. Bakke, I. K. Ding, M. D. McGehee and S. F. Bent, Adv. Energy Mater., 2011, 1, 1169–1175 CrossRef CAS.
- J. Qian, Q.-S. Liu, G. Li, K.-J. Jiang, L.-M. Yang and Y. Song, Chem. Commun., 2011, 47, 6461–6463 RSC.
- S. H. Im, H.-j. Kim, S. W. Kim, S.-W. Kim and S. I. Seok, Energy Environ. Sci., 2011, 4, 4181–4186 CAS.
- J. P. Park, J. H. Heo, S. H. Im and S.-W. Kim, J. Mater. Chem. A, 2016, 4, 785–790 CAS.
- I. Barcelo, J. M. Campina, T. Lana-Villarreal and R. Gomez, Phys. Chem. Chem. Phys., 2012, 14, 5801–5807 RSC.
- J. Duan, Q. Tang, Y. Sun, B. He and H. Chen, RSC Adv., 2014, 4, 60478–60483 RSC.
- Y. Yang and W. Wang, J. Power Sources, 2015, 285, 70–75 CrossRef CAS.
- S. Wang and J. Tian, RSC Adv., 2016, 6, 90082–90099 RSC.
- C. Chen, M. Ye, N. Zhang, X. Wen, D. Zheng and C. Lin, J. Mater. Chem. A, 2015, 3, 6311–6314 CAS.
- F. Liu, J. Zhu, Y. Li, J. Wei, M. Lv, Y. Xu, L. Zhou, L. Hu and S. Dai, J. Power Sources, 2015, 292, 7–14 CrossRef CAS.
- C. V. V. M. Gopi, M. Venkata-Haritha, S.-K. Kim and H.-J. Kim, J. Power Sources, 2016, 311, 111–120 CrossRef CAS.
- A. Sahasrabudhe, S. Kapri and S. Bhattacharyya, Carbon, 2016, 107, 395–404 CrossRef CAS.
- J. Wei, C. Zhang, Z. Du, H. Li and W. Zou, J. Mater. Chem. C, 2014, 2, 4177–4185 RSC.
- D. H. Youn, M. Seol, J. Y. Kim, J.-W. Jang, Y. Choi, K. Yong and J. S. Lee, Chemsuschem, 2013, 6, 261–267 CrossRef CAS PubMed.
- X. Meng, C. Yu, B. Lu, J. Yang and J. Qiu, Nano Energy, 2016, 22, 59–69 CrossRef CAS.
- X. Xin, M. He, W. Han, J. Jung and Z. Lin, Angew. Chem., Int. Ed., 2011, 50, 11739–11742 CrossRef CAS PubMed.
- W. Liang, L. Zhu, H. Liu, F. Xi and W. Li, Electrochim. Acta, 2015, 184, 285–294 CrossRef CAS.
- L. Liu, C. Liu, W. Fu, L. Deng and H. Zhong, ChemPhysChem, 2016, 17, 771–776 CrossRef CAS PubMed.
- C. K. Kamaja, R. R. Devarapalli, Y. Dave, J. Debgupta and M. V. Shelke, J. Power Sources, 2016, 315, 277–283 CrossRef CAS.
- Q. Vu Hong Vinh, J.-H. Kim, S.-H. Kang, C.-J. Choi, J. A. Rajesh and K.-S. Ahn, J. Power Sources, 2016, 316, 53–59 CrossRef.
- K. Zhao, H. Yu, H. Zhang and X. Zhong, J. Phys. Chem. C, 2014, 118, 5683–5690 CAS.
- G. S. Selopal, I. Concina, R. Milan, M. M. Natile, G. Sberveglieri and A. Vomiero, Nano Energy, 2014, 6, 200–210 CrossRef CAS.
- A. D. Savariraj, G. Rajendrakumar, S. Selvam, S. N. Karthick, B. Balamuralitharan, H.-J. Kim, K. K. Viswanathan, M. Vijayakumar and K. Prabakar, RSC Adv., 2015, 5, 100560–100567 RSC.
- S. Wang, T. Shen, H. Bai, B. Li and J. Tian, J. Mater. Chem. C, 2016, 4, 8020–8026 RSC.
- W. Chen, M. Wang, T. Qian, H. Cao, S. Huang, Q. He, N. Liang, C. Wang and J. Zai, Nano Energy, 2015, 12, 186–196 CrossRef CAS.
- M. Wang, W. Chen, J. Zai, S. Huang, Q. He, W. Zhang, Q. Qiao and X. Qian, J. Power Sources, 2015, 299, 212–220 CrossRef CAS.
- H.-J. Kim, L. Myung-Sik, C. V. V. M. Gopi, M. Venkata-Haritha, S. S. Rao and S.-K. Kim, Dalton Trans., 2015, 44, 11340–11351 RSC.
- C. S. Kim, S. H. Choi and J. H. Bang, ACS Appl. Mater. Interfaces, 2014, 6, 22078–22087 CAS.
- M. Ye, X. Wen, N. Zhang, W. Guo, X. Liu and C. Lin, J. Mater. Chem. A, 2015, 3, 9595–9600 CAS.
- M. Venkata-Haritha, C. V. V. M. Gopi, L. Young-Seok and H.-J. Kim, RSC Adv., 2016, 6, 45809–45818 RSC.
- C. Shen, L. Sun, Z. Y. Koh and Q. Wang, J. Mater. Chem. A, 2014, 2, 2807–2813 CAS.
- F. Wang, H. Dong, J. Pan, J. Li, Q. Li and D. Xu, J. Phys. Chem. C, 2014, 118, 19589–19598 CAS.
- C. V. V. M. Gopi, M. Venkata-Haritha, S.-K. Kim, S. S. Rao, D. Punnoose and H.-J. Kim, RSC Adv., 2015, 5, 2963–2967 RSC.
- H. M. Choi, I. A. Ji and J. H. Bang, ACS Appl. Mater. Interfaces, 2014, 6, 2335–2343 CAS.
- X. Q. Chen, Z. Li, Y. Bai, Q. Sun, L. Z. Wang and S. X. Dou, Chem.–Eur. J., 2015, 21, 1055–1063 CrossRef CAS PubMed.
- Y. Bai, C. Han, X. Chen, H. Yu, X. Zong, Z. Li and L. Wang, Nano Energy, 2015, 13, 609–619 CrossRef CAS.
- Z. Yang, C.-Y. Chen, C.-W. Liu and H.-T. Chang, Chem. Commun., 2010, 46, 5485–5487 RSC.
- S. Peng, T. Zhang, L. Li, C. Shen, F. Cheng, M. Srinivasan, Q. Yan, S. Ramakrishna and J. Chen, Nano Energy, 2015, 16, 163–172 CrossRef CAS.
- M. S. Faber, K. Park, M. Caban-Acevedo, P. K. Santra and S. Jin, J. Phys. Chem. Lett., 2013, 4, 1843–1849 CrossRef CAS PubMed.
- J. Xu, H. Xue, X. Yang, H. Wei, W. Li, Z. Li, W. Zhang and C.-S. Lee, Small, 2014, 10, 4754–4759 CrossRef CAS PubMed.
- L. Quan, W. Li, L. Zhu, H. Geng, X. Chang and H. Liu, J. Power Sources, 2014, 272, 546–553 CrossRef CAS.
- H. Geng, L. Zhu, W. Li, H. Liu, L. Quan, F. Xi and X. Su, J. Power Sources, 2015, 281, 204–210 CrossRef CAS.
- S. T. Finn and J. E. Macdonald, Adv. Energy Mater., 2014, 4, 1400495 CrossRef.
- A. D. Mani, M. Deepa, N. Xanthopoulos and C. Subrahmanyam, Mater. Chem. Phys., 2014, 148, 395–402 CrossRef CAS.
- C. V. V. M. Gopi, S. S. Rao, S.-K. Kim, D. Punnoose and H.-J. Kim, J. Power Sources, 2015, 275, 547–556 CrossRef CAS.
- J. Xiao, X. Zeng, W. Chen, F. Xiao and S. Wang, Chem. Commun., 2013, 49, 11734–11736 RSC.
- J. Xu, X. Yang, Q.-D. Yang, T.-L. Wong and C.-S. Lee, J. Phys. Chem. C, 2012, 116, 19718–19723 CAS.
- X. Zeng, D. Xiong, W. Zhang, L. Ming, Z. Xu, Z. Huang, M. Wang, W. Chen and Y.-B. Cheng, Nanoscale, 2013, 5, 6992–6998 RSC.
- X. Zeng, W. Zhang, Y. Xie, D. Xiong, W. Chen, X. Xu, M. Wang and Y.-B. Cheng, J. Power Sources, 2013, 226, 359–362 CrossRef CAS.
- Y. Cao, Y. Xiao, J.-Y. Jung, H.-D. Um, S.-W. Jee, H. M. Choi, J. H. Bang and J.-H. Lee, ACS Appl. Mater. Interfaces, 2013, 5, 479–484 CAS.
- Z. Tachan, M. Shalom, I. Hod, S. Ruehle, S. Tirosh and A. Zaban, J. Phys. Chem. C, 2011, 115, 6162–6166 CAS.
- C. V. Thulasi-Varma, S. S. Rao, K. D. Ikkurthi, S.-K. Kim, T.-S. Kang and H.-J. Kim, J. Mater. Chem. C, 2015, 3, 10195–10206 RSC.
- C.-Y. Lin, C.-Y. Teng, T.-L. Li, Y.-L. Lee and H. Teng, J. Mater. Chem. A, 2013, 1, 1155–1162 CAS.
- B. B. Jin, Y. F. Wang, X. Q. Wang and J. H. Zeng, Appl. Surf. Sci., 2016, 369, 436–442 CrossRef CAS.
- N. Liang, W. Chen, F. Dai, X. Wu, W. Zhang, Z. Li, J. Shen, S. Huang, Q. He, J. Zai, N. Fang and X. Qian, CrystEngComm, 2015, 17, 1902–1905 RSC.
- H. Yu, H. Bao, K. Zhao, Z. Du, H. Zhang and X. Zhong, J. Phys. Chem. C, 2014, 118, 16602–16610 CAS.
- X. Q. Chen, Y. Bai, Z. Li, L. Z. Wang and S. X. Dou, Chempluschem, 2016, 81, 414–420 CrossRef CAS.
- J. Xu, X. Yang, T.-L. Wong and C.-S. Lee, Nanoscale, 2012, 4, 6537–6542 RSC.
- L. Givalou, M. Antoniadou, D. Perganti, M. Giannouri, C.-S. Karagianni, A. G. Kontos and P. Falaras, Electrochim. Acta, 2016, 210, 630–638 CrossRef CAS.
- H.-J. Kim, S.-W. Kim, C. V. V. M. Gopi, S.-K. Kim, S. S. Rao and M.-S. Jeong, J. Power Sources, 2014, 268, 163–170 CrossRef CAS.
- H.-J. Kim, S.-M. Suh, S. S. Rao, D. Punnoose, C. V. Tulasivarma, C. V. V. M. Gopi, N. Kundakarla, S. Ravi and I. K. Durga, J. Electroanal. Chem., 2016, 777, 123–132 CrossRef CAS.
- M. Eskandari, V. Ahmadi and R. Ghahary, Electrochim. Acta, 2015, 151, 393–398 CrossRef CAS.
- C. V. V. M. Gopi, M. Venkata-Haritha, S. Ravi, C. V. Thulasi-Varma, S.-K. Kim and H.-J. Kim, J. Mater. Chem. C, 2015, 3, 12514–12528 RSC.
- X. Meng, C. Yu, X. Song, Y. Liu, S. Liang, Z. Liu, C. Hao and J. Qiu, Adv. Energy Mater., 2015, 5, 1500180 CrossRef.
- X. Meng, C. Yu, X. Song, Z. Liu, B. Lu, C. Hao and J. Qiu, J. Mater. Chem. A, 2017, 5, 2280–2287 CAS.
- B. Fang, M. Kim, S.-Q. Fan, J. H. Kim, D. P. Wilkinson, J. Ko and J.-S. Yu, J. Mater. Chem., 2011, 21, 8742–8748 RSC.
- D. Van-Duong, P. Kim, S. Baek, L. L. Larina, K. Yong, R. Ryoo, S. H. Ko and H.-S. Choi, Carbon, 2016, 96, 139–144 CrossRef.
- J. Dong, S. Jia, J. Chen, B. Li, J. Zheng, J. Zhao, Z. Wang and Z. Zhu, J. Mater. Chem., 2012, 22, 9745–9750 RSC.
- S.-Q. Fan, B. Fang, J. H. Kim, J.-J. Kim, J.-S. Yu and J. Ko, Appl. Phys. Lett., 2010, 96, 063501 CrossRef.
- M. Seol, E. Ramasamy, J. Lee and K. Yong, J. Phys. Chem. C, 2011, 115, 22018–22024 CAS.
- Q. Zhang, S. Zhou, Q. Li and H. Li, RSC Adv., 2015, 5, 30617–30623 RSC.
- V.-D. Dao, Y. Choi, K. Yong, L. L. Larina and H.-S. Choi, Carbon, 2015, 84, 383–389 CrossRef CAS.
- S. S. Rao, I. K. Durga, T.-S. Kang, S.-K. Kim, D. Punnoose, C. V. V. M. Gopi, A. E. Reddy, T. N. V. Krishna and H.-J. Kim, Dalton Trans., 2016, 45, 3450–3463 RSC.
- S. D. Sung, I. Lim, P. Kang, C. Lee and W. I. Lee, Chem. Commun., 2013, 49, 6054–6056 RSC.
- W. Guo, Z. Du, Q. Zhao, H. Zhang and X. Zhong, J. Phys. Chem. C, 2016, 120, 16500–16506 CAS.
- J. G. Radich, R. Dwyer and P. V. Kamat, J. Phys. Chem. Lett., 2011, 2, 2453–2460 CrossRef CAS.
- J. H. Zeng, D. Chen, Y. F. Wang and B. B. Jin, J. Mater. Chem. C, 2015, 3, 12140–12148 RSC.
- L. Li, P. Zhu, S. Peng, M. Srinivasan, Q. Yan, A. S. Nair, B. Liu and S. Samakrishna, J. Phys. Chem. C, 2014, 118, 16526–16535 CAS.
- Y. Zhu, H. Cui, S. Jia, J. Zheng, P. Yang, Z. Wang and Z. Zhu, Electrochim. Acta, 2016, 208, 288–295 CrossRef CAS.
- D. Ghosh, G. Halder, A. Sahasrabudhe and S. Bhattacharyya, Nanoscale, 2016, 8, 10632–10641 RSC.
- H. Geng, L. Zhu, W. Li, H. Liu, X. Su, F. Xi and X. Chang, Electrochim. Acta, 2015, 182, 1093–1100 CrossRef CAS.
- P. Parand, M. Samadpour, A. Esfandiar and A. I. Zad, ACS Photonics, 2014, 1, 323–330 CrossRef CAS.
- Y. Jiang, B.-B. Yu, J. Liu, Z.-H. Li, J.-K. Sun, X.-H. Zhong, J.-S. Hu, W.-G. Song and L.-J. Wan, Nano Lett., 2015, 15, 3088–3095 CrossRef CAS PubMed.
- M. Eskandari, R. Ghahary, M. Shokri and V. Ahmadi, RSC Adv., 2016, 6, 51894–51899 RSC.
- C. V. V. M. Gopi, M. Venkata-Haritha, Y.-S. Lee and H.-J. Kim, J. Mater. Chem. A, 2016, 4, 8161–8171 CAS.
- G. Yue, F. Tan, J. Wu, F. Li, J. Lin, M. Huang and W. Zhang, RSC Adv., 2015, 5, 42101–42108 RSC.
- M. Seol, D. H. Youn, J. Y. Kim, J.-W. Jang, M. Choi, J. S. Lee and K. Yong, Adv. Energy Mater., 2014, 4, 1300775 CrossRef.
- J. Soo Kang, M.-A. Park, J.-Y. Kim, S. Ha Park, D. Young Chung, S.-H. Yu, J. Kim, J. Park, J.-W. Choi, K. Jae Lee, J. Jeong, M. Jae Ko, K.-S. Ahn and Y.-E. Sung, Sci. Rep., 2015, 5, 10450 CrossRef PubMed.
- M.-H. Yeh, L.-Y. Lin, C.-P. Lee, C.-Y. Chou, K.-W. Tsai, J.-T. s. Lin and K.-C. Ho, J. Power Sources, 2013, 237, 141–148 CrossRef CAS.
- T. Shu and Z.-L. Ku, J. Alloys Compd., 2014, 586, 257–260 CrossRef CAS.
|
This journal is © The Royal Society of Chemistry 2017 |
Click here to see how this site uses Cookies. View our privacy policy here.