DOI:
10.1039/C7BM00747G
(Paper)
Biomater. Sci., 2018,
6, 200-206
Zwitterionic stealth peptide-protected gold nanoparticles enable long circulation without the accelerated blood clearance phenomenon†
Received
16th August 2017
, Accepted 20th November 2017
First published on 24th November 2017
Abstract
Poly(ethylene glycol) (PEG), which is considered as a gold standard for surface modification of nanoparticles in biomedical applications, has been reported to encounter the accelerated blood clearance (ABC) phenomenon after repeated administration. Herein, as an ideal substitute for PEG, a zwitterionic peptide sequence of alternating negatively charged glutamic acid (E) and positively charged lysine (K) was designed as a good candidate for surface modification of nanoparticles without the ABC phenomenon in vivo. PEG-protected gold nanoparticles (AuNP-PEG) suffered from a serious ABC phenomenon with very fast blood clearance after repeated injection. Meanwhile, the plasma IgM and IgG levels were significantly increased after the repeated injection of AuNP-PEG. However, zwitterionic stealth peptide-protected gold nanoparticles (AuNP-EK10) could avoid the activation of the ABC phenomenon. The increase of IgM and IgG levels was not observed after the repeated injection of AuNP-EK10. More interestingly, compared to AuNP-PEG, more AuNP-EK10 could be accumulated in tumor tissues after repeated injection of the nanoparticles to tumor-bearing nude mice, which might be especially important for the design of drug nanocarriers in cancer therapy.
Introduction
Nanomedicine, which applies nanotechnology for better treatment of numerous medical issues, is one of the most hot research topics.1–5 As is known, nanoparticles (NPs) can be accumulated in tumor tissue in a passive way by the enhanced permeability and retention (EPR) effect.6 On the other hand, NPs can be easily cleared by the reticuloendothelial system (RES), which results in a short blood circulation time and low tumor accumulation. The surface engineering of NPs is extremely important to obtain a prolonged circulation time.7,8 At present, PEGylation of NPs is the most successful strategy to achieve improved colloidal stability, prolonged circulation time, minimal immunogenicity, and enhanced tumor accumulation.9–11 However, PEGylated NPs always suffer from reduced uptake by cancer cells, which is well known as the “PEG dilemma”.12,13 More importantly, PEGylated NPs can lead to the accelerated blood clearance (ABC) phenomenon upon repeated injections owing to the presence of anti-PEG antibodies.14–16 Therefore, it is of great interest to search for alternative materials other than PEG.
Many strategies have been reported to overcome the drawbacks caused by PEGylated NPs, especially the ABC phenomenon.17–21 Zwitterionic surface engineering is considered as one of the most promising strategies.22,23 Zwitterions are considered as ideal candidates for designing bio-inspired nanomaterials, which can minimize the interaction with biological components such as proteins and cells. We and other groups have fabricated a series of zwitterionic nanomaterials using phosphorylcholine (PC), carboxybetaine (CB), sulfobetaine (SB), and other zwitterionic molecules.24–29 Very recently, mixed charged zwitterionic NPs were reported using various counter-charged terminal groups, which is regarded as a much more simple and feasible strategy to fabricate zwitterionic NPs.30,31 More importantly, naturally occurring components such as sugars, proteins, and peptides are always more appealing in biomedical applications owing to their biocompatibility and non-immunogenicity. A non-fouling zwitterionic peptide sequence of alternating negatively charged glutamic acid (E) and positively charged lysine (K) was recently reported to construct non-fouling materials.32–35 Unfortunately, the in vivo fate of such zwitterionic peptide sequence-modified NPs is still completely unknown, which is however of great importance for their biomedical applications.
In this research, we tried to synthesize zwitterionic peptide sequence EK-protected NPs. Owing to the bio-based zwitterionic peptide coating, the NPs are expected to have a stealth surface, thus preventing the production of antibodies, avoiding the ABC phenomenon, and having a long circulation time. In order to prove this hypothesis, polyethylene glycol (PEG)-protected NPs are used as the negative control since PEG is considered as the gold standard in the biomaterial area. Meanwhile, gold nanoparticles (AuNPs) are used as model NPs because of their biocompatibility and bioinertia.
Experimental
Materials
Hydrogen tetrachloroaurate(III) hydrate (HAuCl4·4H2O) and trisodium citrate dehydrate (C6H5Na3O7·2H2O) were purchased from Sinopharm Chemical Reagent Co. Ltd. α-Methoxy-ω-thiol-poly(ethylene glycol) (HS-PEG, MW 2 kDa) was obtained from Shanghai Xibao Biochemical Co., Ltd. The peptide CGGGEKEKEKEKEKEKEKEKEKEK (HS-EK10) was custom-synthesized by Shanghai Top-peptide Biotechnology Co., Ltd with more than 95% purity and confirmed by High-Performance Liquid Chromatography (HPLC) and electrospray ionization mass spectrometry (ESI-MS) (Fig. S1 and S2†). Human umbilical vein endothelial cells (HUVECs) and HeLa cells were obtained from KeyGen BioTECH (Nanjing, China). MilliQ water (18.2 MΩ cm−1) was obtained using a Millipore MilliQ Academic Water Purification System. All other reagents were used as received without further purification.
Synthesis of HS-EK10-protected AuNPs (AuNP-EK10)
The synthesis of citrate-protected AuNPs followed the reported procedure.36 In a 250 mL round-bottom flask equipped with a condenser, 100 mL of MilliQ water was added. After boiling, 4.12 mL of 10 mg mL−1 HAuCl4 was added with vigorous stirring. Rapid addition of 11.57 mL of 38.8 mM sodium citrate to the boiling solution resulted in a color change from pale yellow to burgundy. Boiling was continued for 10 min; the heating mantle was then removed, and stirring was continued for an additional 15 min. The UV-Vis spectrum exhibits a characteristic plasmon band at 519 nm. The diameter of the particles, measured from TEM images, was 15.8 nm ± 2 nm.
HS-EK10-protected AuNPs were obtained from the citrate-coated Au-NPs by the exchange of citrate molecules with HS-EK10. This reaction was performed according to the literature.34 A large excess equivalent of thiol ligand was used: 100 monolayers per particle, estimated by assuming that the occupied surface area by a single thiol molecule is ca. 0.20 nm2. Using this calculation, 1 mL of 0.05 M HS-EK10 aqueous solution was added into 12 mL of citrate-coated Au-NP solution. The mixture was rapidly stirred at room temperature for 24 h. The HS-EK10-protected AuNPs were purified by ultrafiltration at 4300 rpm three times, and then re-dispersed in phosphate buffered saline (PBS).
The HS-PEG-protected AuNPs (AuNP-PEG) were synthesized using the same method except that HS-PEG was used instead of HS-EK10.
Cell culture
The HeLa cells were cultured in Dulbecco's modified Eagle's medium (DMEM) supplemented with 10% fetal bovine serum (FBS) and 1% penicillin–streptomycin. HUVEC cells were cultured with RPMI 1640 supplemented with 10% FBS. All the cells were incubated at 37 °C under a humidified atmosphere containing 5% CO2.
In vitro cell viability assays
The cell viability of AuNP-EK10 was evaluated by the standard 3-(4,5-dimethylthiazol-2-yl)-2,5-diphenyltetrazolium bromide (MTT) assays. HUVEC cells were seeded into a 96-well plate at a density of 5000 cells per well using RPMI 1640 for 24 h. AuNP-EK10 with a Au concentration from 1 to 50 mM was added into the cells respectively. 48 h later, 20 μL of MTT (0.1 mg mL−1) was added. The cells were then cultured for another 4 h. The culture medium was removed, 150 μL of DMSO was added to dissolve the obtained crystals, and the absorbance was measured at a wavelength of 490 nm. Data are expressed as average ± SD (n = 4). The cell viability of AuNP-PEG was investigated using the same method except that AuNP-PEG was used instead of AuNP-EK10.
In vivo assays
All in vivo experiments were performed according to the “Principles of Laboratory Animal Care” (NIH publication no. 86-23, revised 1985) and the guidelines for Lab Animal Welfare and Research Committee, Zhejiang University. Healthy Sprague-Dawley (SD) rats and BALB/c nude mice were purchased from Vital River Laboratory Animal Technology Co. Ltd.
Blood clearance evaluation
In order to evaluate the blood circulation time of the AuNPs, SD rats were used as the animal model and randomly divided into 2 groups (n = 5). The rats were intravenously administered with AuNP-EK10 or AuNP-PEG at a dose of 2 mg per kg body weight for three injections. The concentration of the remaining Au in the blood at different time intervals was measured by inductively coupled plasma mass spectrometry (ICP-MS). Blood samples were collected by eye puncture at 15 min, 1 h, 3 h, 6 h, 12 h, and 24 h after every injection. Every injection was received seven days apart.
Determination of IgM and IgG levels in SD rats
To detect the IgM level in the blood, SD rats were intravenously administered with one injection of AuNP-EK10 or AuNP-PEG at a dose of 2 mg per kg body weight. Rats treated with PBS were used as the control. After five days, blood samples were collected. Serum samples were then collected after centrifugation at 10
000 rpm at 4 °C for 15 min. The IgG levels were detected using the SD rats after two injections of AuNPs at a dose of 2 mg per kg body weight. 12 days after the second injection, blood samples were collected. Serum samples were then collected after centrifugation at 10
000 rpm at 4 °C for 15 min. Quantification of the IgM and IgG levels was carried out by enzyme-linked immunosorbent assay (ELISA) using a Rat IgM ELISA Quantification Kit and Rat IgG ELISA Quantification Kit (eBioscience, USA) according to the manufacturer's instructions. Briefly, 100 μL of 1
:
250 diluted primary antibodies (anti-rat IgM or anti-rat IgG antibodies) in coating buffer (pH 9.6) was added to each well of a 96-well plate and then incubated overnight at 4 °C. The wells were then washed five times with wash buffer (pH 8.0). After that, 250 μL of blocking solution (pH 8.0) was added to each well and incubated for 1 h at room temperature. After incubation, the blocking solution was aspirated and the wells were washed five times with wash solution and 100 μL of 1
:
100 standard or sample diluent was added to the wells. After incubation for 2 h at room temperature, the wells were washed five times with the wash solution. 100 μL of a second antibody conjugated to horseradish peroxidase (HRP) for detection (anti-rat IgM-HRP or anti-rat IgG-HRP conjugate) in the conjugate diluent (pH 8.0) was transferred to each well. After incubation for 1 h at room temperature, the detection antibody was removed and the wells were washed five times with the wash solution. The enzyme assay was initiated by adding 3,3′,5,5′-tetramethylbenzidine substrate solution. After 15 min of incubation, the reaction was stopped by adding 100 μL of 0.5 M H2SO4 and the optical density (OD) values were measured at 450 nm using a microplate reader.
The specific binding of IgM with AuNPs was evaluated by incubation of AuNPs with the plasma obtained from the rats injected with AuNP-PEG. Briefly, plasma samples (200 μL) were collected on the fifth day after the first injection of AuNP-PEG. 50 μL of AuNP-EK10 (1 mg mL−1), AuNP-PEG (1 mg mL−1), or PBS was incubated with plasma (200 μL) at 37 °C for 15 min. After that, AuNPs were removed by centrifugation. The IgM remaining in the plasma was measured by ELISA as described above.
Determination of IgM and IgG levels in HeLa tumor-bearing nude mice
HeLa cells (1 × 106) in 0.1 mL of PBS were injected subcutaneously into the right rear flank area of female nude BALB/c mice. Once the HeLa xenografts reached around 100 mm3, the mice were intravenously administered with AuNP-EK10 or AuNP-PEG. All other procedures for the determination of IgM and IgG levels in HeLa tumor-bearing nude mice were the same as those in SD rats as described above.
Biodistribution of AuNPs in HeLa tumor-bearing nude mice
HeLa cells (1 × 106) in 0.1 mL of PBS were injected subcutaneously into the right rear flank area of female nude BALB/c mice. Once the HeLa xenografts reached around 100 mm3, the mice were randomly divided into 4 groups (n = 5). The first two groups were intravenously administered with AuNP-EK10 or AuNP-PEG at a dose of 2 mg per kg body weight for only one injection. The other two groups were intravenously administered with AuNP-EK10 or AuNP-PEG at a dose of 2 mg per kg body weight for two injections five days apart. The nude mice were then sacrificed 24 h post injection. The main organs and tumor were then harvested. The organs and tumors were washed with PBS, weighed and lyophilized for 1 day. The dried tissues and blood were mashed and dissolved in aqua regia for 1 day. The aqua regia was then diluted and the precipitated tissue debris was removed by centrifugation at 10
000 rpm for 5 min. The Au content in the supernatant was measured by ICP-MS.
Statistical analysis
The results were expressed as the mean ± standard deviation (SD). The difference between the two groups and multiple groups were analyzed by Student's t-test and one-way ANOVA, respectively. The difference was considered no significance for n.s., statistically significant when *p < 0.05 and very significant when **p < 0.01.
Characterization
Dynamic light scattering (DLS) and zeta-potential measurements were performed with a Zetasizer Nano-ZS from Malvern Instruments, equipped with a He–Ne laser at a wavelength of 633 nm at 25 °C. Transmission Electron Microscopy (TEM) analysis was performed on a JEM-3010 instrument operating at 80 kV in bright-field mode. AuNP samples were prepared by placing a drop of the colloidal solution on a 400-mesh carbon-coated copper grid and air-drying the grid at 25 °C. For the determination of the particle size, over 100 particles were counted in multiple pictures from different areas of the TEM grid. UV-vis spectra were recorded with a UV-vis Shimadzu UV-2505 spectrometer using 1 cm-path length quartz cuvettes. The spectra were collected within the range of 400–800 nm. The Au concentration was measured using ICP-MS (Thermo Elemental Corporation) after being treated with aqua regia. Cell viability was measured with a microplate reader (MODEL 550; Bio-Rad) at an absorbance of 490 nm.
Results and discussion
Synthesis and characterization of AuNPs
Citrate-capped AuNPs were synthesized by a standard method. The diameter of the citrate-capped AuNPs was 15.8 nm with a plasmon band at 519 nm (Fig. S3 and S4†). EK10-protected Au-NPs (Au-EK10) were prepared by exchanging citrate with HS-EK10. The control, PEG-protected AuNPs (AuNP-PEG), was synthesized by the same method. The hydrodynamic diameter of AuNP-EK10 was 18.5 nm as measured by DLS (Fig. 1a). Meanwhile, the hydrodynamic diameter of AuNP-PEG was 20.6 nm, which was slightly larger than that of AuNP-EK10, which might be attributed to a much longer PEG chain (Fig. 1b). The surface charge of NPs is a very important factor for their biomedical applications. The zeta potential of AuNP-EK10 and AuNP-PEG was −6.3 mV and −3.8 mV at pH 7.4, respectively. Both of them were a little negatively charged, which is very beneficial for their in vivo applications.
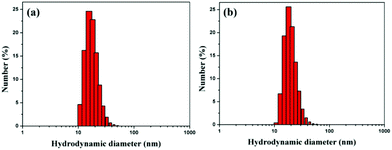 |
| Fig. 1 Hydrodynamic diameters of (a) AuNP-EK10 and (b) AuNP-PEG measured by DLS. | |
In vitro characterization of AuNPs
Excellent stability and biocompatibility are the necessary prerequisites for NPs in biomedical applications. The colloidal stability of AuNP-EK10 and AuNP-PEG was first investigated in PBS and fetal bovine serum (FBS) solution. As shown in Fig. 2, the hydrodynamic diameters of AuNP-EK10 and AuNP-PEG almost didn't change in PBS and FBS after 48 h, exhibiting outstanding colloidal stability. Furthermore, the cytotoxicity of AuNP-EK10 and AuNP-PEG against HUVEC cells was studied by MTT assay. The addition of AuNP-EK10 or AuNP-PEG almost didn't influence the viability of HUVEC cells (Fig. 3), suggesting excellent biocompatibility.
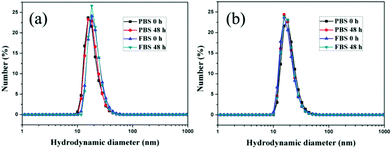 |
| Fig. 2 The hydrodynamic diameters of (a) AuNP-EK10 and (b) AuNP-PEG in PBS and FBS after incubation for 0 h and 48 h. | |
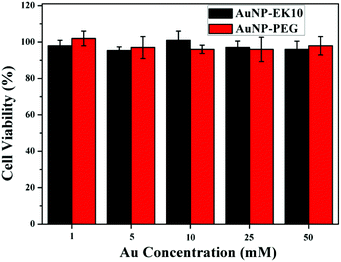 |
| Fig. 3 Cytotoxicity of AuNP-EK10 and AuNP-PEG against HUVEC cells after 48 h incubation (n = 4). | |
In vivo blood clearance evaluation
Repeated administration is always necessary in the clinic, especially in chemotherapy. The in vivo blood circulation of AuNPs was investigated after repeated administration. As is known, PEGylated NPs can induce the ABC phenomenon after repeated administration,37,38 leading to enhanced liver clearance and low tumor accumulation, which significantly restricts the therapeutic efficacy of PEGylated nanomedicine. More and more evidence shows that the ABC phenomenon can significantly influence the targeting ability and bioavailability of nanomedicine.
In this research, two doses of AuNPs were injected into rats five days apart. When AuNP-PEG was injected, more than 20% of the injection dose (ID) of Au content was detected in the blood after 24 h of the first injection (Fig. 4a). However, when AuNP-PEG was injected once more at the same dose seven days after the first injection, very fast blood clearance was observed. Only 19.2% of ID g−1 Au content was detected after 1.5 h. There was only 2.1% of ID g−1 Au content in the blood after 24 h, which was only one-tenth of the first injection. What's more, the blood clearance of AuNP-PEG was even faster after the third injection. Therefore, AuNP-PEG suffered from a severe ABC phenomenon. Interestingly, when AuNP-EK10 was injected, we didn't find a significant difference in the blood circulation of AuNP-EK10 among the first injection, second injection, and third injection (Fig. 4b). There was still 16.4% of ID g−1 Au content in the blood 24 h after the third injection, compared to 19.6% of ID g−1 Au content in the blood 24 h after the first injection. Therefore, EK10-protected AuNPs could effectively avoid the ABC phenomenon, leading to a prolonged circulation time after repeated administration.
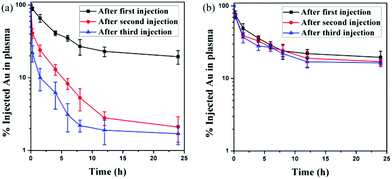 |
| Fig. 4
In vivo blood circulation curves of (a) AuNP-PEG and (b) AuNP-EK10 after three injections (n = 5). | |
Detection of the production of antibodies
It is believed that the production of antibodies is an important reason for the ABC phenomenon. Whether AuNP-EK10 would result in the production of antibodies was studied by repeated administration of AuNP-EK10 into SD rats. The plasma IgM level was investigated by ELISA five days post injection. As shown in Fig. 5a, the SD rats injected with AuNP-PEG produced a high level of IgM, which was 7.3 times higher than the control PBS group. In contrast, no noticeable increase of IgM was observed after the injection with AuNP-EK10 compared with the PBS group. These results indicated that the stealth zwitterionic EK10 coating would not stimulate the IgM production, thereby avoiding the ABC phenomenon for the following injection.
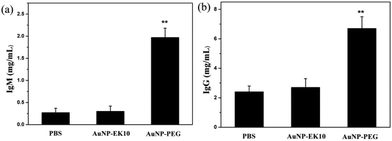 |
| Fig. 5 (a) The IgM level in plasma after injection of AuNP-EK10 and AuNP-PEG to SD rats five days later measured by ELISA. (b) The IgG level in plasma after two injections of AuNP-EK10 and AuNP-PEG to SD rats measured by ELISA. *p < 0.05, **p < 0.01 (n = 5). | |
The IgG level, which relies on IgM stimulation, is another important index of immune response when foreign materials are injected into the body. The IgG production was evaluated by ELISA 12 days after the second injection. As shown in Fig. 5b, the IgG level of the rats treated with AuNP-PEG was 2.9 times higher than that of the PBS group. However, the IgG level from the AuNP-EK10 group was almost the same as that from the PBS group, which was consistent with the IgM level discussed above. These results again proved that the zwitterionic EK10 coating could avoid the activation of the ABC phenomenon in vivo.
In order to validate whether the produced IgM could specifically bind to AuNP-PEG, AuNP-PEG and AuNP-EK10 were incubated with plasma obtained from SD rats administered with AuNP-PEG five days before. After centrifugation to remove AuNPs, the supernatant was collected to measure the IgM level. If IgM is bound to AuNPs, the IgM level in the supernatant after centrifugation will decrease. As shown in Fig. 6, after incubation with AuNP-PEG, IgM in the supernatant was significantly decreased, probably owing to the specific binding of IgM to AuNP-PEG. Meanwhile, compared to the serum treated with PBS, there was almost no difference of IgM after incubation of the serum with AuNP-EK10. These results further confirmed that the activated IgM could specifically bind to AuNP-PEG and thereby resulted in the ABC phenomenon. In contrast, AuNP-EK10 with a zwitterionic stealth surface cannot bind the produced IgM and could avoid the ABC phenomenon.
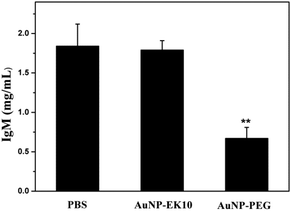 |
| Fig. 6 The IgM level in plasma incubated with AuNP-EK10 and AuNP-PEG. The plasma was obtained from SD rats administered with AuNP-PEG five days before. The supernatant was collected to measure the IgM level after centrifugation using ELISA. *p < 0.05, **p < 0.01 (n = 5). | |
Biodistribution of AuNPs in tumor-bearing nude mice
Owing to the ABC phenomenon, AuNP-PEG would undergo fast clearance from blood circulation. In order to investigate how AuNPs were cleared from blood circulation and how it would influence the accumulation in tumor sites, the biodistribution of AuNP-EK10 and AuNP-PEG in the HeLa tumor-bearing nude mice was investigated after two injections of AuNPs at a dose of 2 mg per kg body weight. Since the blood volume in nude mice is very limited, we didn't study the pharmacokinetics of the AuNPs in nude mice. The IgM and IgG levels in the tumor-bearing nude mice were investigated and the results are shown in Fig. 7. The increase of IgM and IgG levels was observed as well after repeated injection of AuNP-PEG to the tumor-bearing nude mice. Meanwhile, compared to the results in SD rats, the IgM and IgG levels in the tumor-bearing nude mice were much lower after repeated injection of AuNP-PEG, which might be ascribed to the T cell deficiency and tumor burden in nude mice. However, the IgM and IgG levels almost didn't change after repeated injection of AuNP-EK10 to the tumor-bearing nude mice, which was consistent with the results in SD rats. Furthermore, as shown in Fig. 8, we didn't find any significant difference in the biodistribution of AuNP-EK10 and AuNP-PEG in the main organs and tumors after the first injection. Interestingly, after the second injection, the hepatic accumulation of AuNP-PEG was significantly increased, which was about 1.4 times higher than that of AuNP-EK10. More importantly, since AuNP-EK10 could avoid the ABC phenomenon, the accumulation of AuNP-EK10 in the tumor site was 1.7 times higher than that of AuNP-PEG. Therefore, compared to AuNP-PEG, more AuNP-EK10 can be accumulated in tumor tissues. The selective accumulation of NPs in tumor tissues is extremely important for cancer therapy since it can achieve improved therapeutic efficacy as well as reduced side effects.
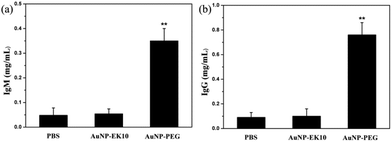 |
| Fig. 7 (a) The IgM level in plasma after injection of AuNP-EK10 and AuNP-PEG to tumor-bearing nude mice five days later measured by ELISA. (b) The IgG level in plasma after two injections of AuNP-EK10 and AuNP-PEG to tumor-bearing nude mice measured by ELISA. *p < 0.05, **p < 0.01 (n = 5). | |
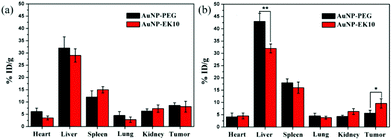 |
| Fig. 8 The biodistribution of AuNP-EK10 and AuNP-PEG in tumor-bearing nude mice after (a) the first injection and (b) the second injection. *p < 0.05, **p < 0.01 (n = 5). | |
Conclusions
In summary, the zwitterionic peptide EK10 was used as a nonfouling coating to prepare stealth NPs (AuNP-EK10). AuNP-EK10 exhibited excellent colloidal stability and biocompatibility. Different from AuNP-PEG which could induce the ABC phenomenon, AuNP-EK10 could effectively avoid the ABC phenomenon after repeated administration. In addition, AuNP-EK10 would not induce immune response. However, the repeated administration of AuNP-PEG would increase the plasma IgM level and IgG level, which is a characteristic sign of the activation of immune response. Therefore, compared to AuNP-PEG, AuNP-EK10 exhibited prolonged blood circulation, reduced liver clearance, and enhanced tumor accumulation, thus it can be expected to show a better therapeutic efficacy. The zwitterionic peptide EK10 showed superior performance in vivo over its counterpart PEG, which might have great potential as a versatile coating in biomedical applications.
Conflicts of interest
There are no conflicts to declare.
Acknowledgements
Financial support from the National Natural Science Foundation of China (21774110 and 51573160) is gratefully acknowledged.
Notes and references
- V. P. Torchilin, Nat. Rev. Drug Discovery, 2014, 13, 813–827 CrossRef CAS PubMed.
- L. K. Bogart, G. Pourroy, C. J. Murphy, V. Puntes, T. Pellegrino, D. Rosenblum, D. Peer and R. Lévy, ACS Nano, 2014, 8, 3107–3122 CrossRef CAS PubMed.
- S. Augustine, J. Singh, M. Srivastava, M. Sharma, A. Das and B. D. Malhotra, Biomater. Sci., 2017, 5, 901–952 RSC.
- Y. Ma, J. Huang, S. Song, H. Chen and Z. Zhang, Small, 2016, 12, 4936–4954 CrossRef CAS PubMed.
- S. Wang, P. Huang and X. Chen, ACS Nano, 2016, 10, 2991–2994 CrossRef CAS PubMed.
- Y. Matsumura and H. Maeda, Cancer Res., 1986, 46, 6387–6392 CAS.
- T. X. Nguyen, L. Huang, M. Gauthier, G. Yang and Q. Wang, Nanomedicine, 2016, 11, 1169 CrossRef CAS PubMed.
- G. Storm, S. O. Belliot, T. Daemen and D. D. Lasic, Adv. Drug Delivery Rev., 1995, 17, 31–48 CrossRef CAS.
- J. V. Jokerst, T. Lobovkina, R. N. Zare and S. S. Gambhir, Nanomedicine, 2011, 6, 715–728 CrossRef CAS PubMed.
- C. Chen, P. Zheng, Z. Cao, Y. Ma, J. Li, H. Qian, W. Tao and X. Yang, Biomater. Sci., 2016, 4, 412–417 RSC.
- H. Otsuka, Y. Nagasaki and K. Kataoka, Adv. Drug Delivery Rev., 2012, 64, 246–255 CrossRef.
- C. Y. Sun, S. Shen, C. F. Xu, H. J. Li, Y. Liu, Z. T. Cao, X. Z. Yang, J. X. Xia and J. Wang, J. Am. Chem. Soc., 2015, 137, 15217–15224 CrossRef CAS PubMed.
- A. Szlagatys-Sidorkiewicz, A. Borkowska, K. Popińska, E. Toporowska-Kowalska, U. Grzybowska-Chlebowczyk, A. Wernicka, E. Hapyn, M. Sibilska, B. Gębora-Kowalska, S. Więcek, P. Zagożdżon and J. Kierkuś, Adv. Med. Sci., 2016, 61, 1–5 CrossRef PubMed.
- T. Ishida, R. Maeda, M. Ichihara, K. Irimura and H. Kiwada, J. Controlled Release, 2003, 88, 35–42 CrossRef CAS PubMed.
- Y. Zhao, L. Wang, M. Yan, Y. Ma, G. Zang, Z. She and Y. Deng, Int. J. Nanomed., 2012, 7, 2891–2900 CAS.
- P. Zhang, F. Sun, S. Liu and S. Jiang, J. Controlled Release, 2016, 244, 184–193 CrossRef CAS PubMed.
- T. Ishihara, T. Maeda, H. Sakamoto, N. Takasaki, M. Shigyo, T. Ishida, H. Kiwada, Y. Mizushima and T. Mizushima, Biomacromolecules, 2010, 11, 2700–2706 CrossRef CAS PubMed.
- L. Rao, L. Bu, J. Xu, B. Cai, G. Yu, X. Yu, Z. He, Q. Huang, A. Li, S. Guo, W. Zhang, W. Liu, Z. Sun, H. Wang, T. Wang and X. Zhao, Small, 2015, 11(46), 6225–6236 CrossRef CAS PubMed.
- L. Zhang, Y. Liu, G. Liu, D. Xu, S. Liang, X. Zhu, Y. Lu and H. Wang, Nano Res., 2016, 9, 2424–2432 CrossRef CAS.
- Y. Li, R. Liu, Y. Shi, Z. Zhang and X. Zhang, Theranostics, 2015, 5, 583–596 CrossRef CAS PubMed.
- W. Yang, S. Liu, T. Bai, A. J. Keefe, L. Zhang, J. R. Ella-Menye, Y. Li and S. Jiang, Nano Today, 2014, 9, 10–16 CrossRef CAS.
- R. Matsuno and K. Ishihara, Nano Today, 2011, 6, 61–74 CrossRef CAS.
- Q. Jin, Y. Chen, Y. Wang and J. Ji, Colloids Surf., B, 2014, 124, 80–86 CrossRef CAS PubMed.
- J. Wang, S. Yuan, Y. Zhang, W. Wu, Y. Hu and X. Jiang, Biomater. Sci., 2016, 4, 1351–1360 RSC.
- R. Sun, X. Du, C. Sun, S. Shen, Y. Liu, X. Yang, Y. Bao, Y. Zhu and J. Wang, Biomater. Sci., 2015, 3, 1105–1113 RSC.
- C. M. Qiao, J. D. Liu, J. Yang, Y. Li, J. Weng, Y. M. Shao and X. Zhang, Biomaterials, 2016, 85, 1–17 CrossRef CAS PubMed.
- C. Qiao, J. Yang, L. Chen, J. Weng and X. Zhang, Biomater. Sci., 2017, 5, 1603–1611 RSC.
- X. J. Chen, S. McRae, S. Parelkar and T. Emrick, Bioconjgate Chem., 2009, 20, 2331–2341 CrossRef CAS PubMed.
- W. Li, Q. Liu, P. Zhang and L. Liu, Acta Biomater., 2016, 40, 254–262 CrossRef CAS PubMed.
- P. J. Bonitatibus Jr., A. S. Torres, B. Kandapallil, B. D. Lee, G. D. Goddard, R. E. Colborn and M. E. Marino, ACS Nano, 2012, 6, 6650–6658 CrossRef PubMed.
- X. Liu, H. Li, Y. Chen, Q. Jin, K. Ren and J. Ji, Small, 2014, 3, 1439–1447 CAS.
- A. K. Nowinski, F. Sun, A. D. White, A. J. Keefe and S. Y. Jiang, J. Am. Chem. Soc., 2012, 134, 6000–6005 CrossRef CAS PubMed.
- A. K. Nowinski, A. D. White, A. J. Keefe and S. Y. Jiang, Langmuir, 2014, 30, 1864–1870 CrossRef CAS PubMed.
- J. Wu, H. Han, Q. Jin, Z. Li, H. Li and J. Ji, ACS Appl. Mater. Interfaces, 2017, 9, 14596–14605 CAS.
- J. Wu, Y. Lin, H. Li, Q. Jin and J. Ji, J. Colloid Interface Sci., 2017, 485, 251–259 CrossRef CAS PubMed.
- C. Katherine, R. Griffith, B. Michael and J. Michael, Anal. Chem., 1995, 67, 735–743 CrossRef.
- T. Ishida, K. Atobe, X. Wang and H. Kiwada, J. Controlled Release, 2006, 115, 251–258 CrossRef CAS PubMed.
- P. Laverman, M. G. Carstens, O. C. Boerman, E. T. M. Dams, W. J. G. Oyen, N. van Rooijen, F. H. M. Corstens and G. Storm, J. Pharmacol. Exp. Ther., 2001, 298, 607–612 CAS.
Footnotes |
† Electronic supplementary information (ESI) available. See DOI: 10.1039/c7bm00747g |
‡ These authors contributed equally. |
|
This journal is © The Royal Society of Chemistry 2018 |
Click here to see how this site uses Cookies. View our privacy policy here.