DOI:
10.1039/C7FO01460K
(Paper)
Food Funct., 2018,
9, 614-623
Anti-diabetic activity of a polyphenol-rich extract from Phellinus igniarius in KK-Ay mice with spontaneous type 2 diabetes mellitus†
Received
21st September 2017
, Accepted 6th December 2017
First published on 6th December 2017
Abstract
The present study investigated the anti-diabetic activity and potential mechanisms of the polyphenol rich extract from Phellinus igniarius (PI-PRE) in vitro and in vivo. Four main phenolic compounds of PI-PRE were purified and identified as 7,8-dihydroxycoumarin, 3,4-dihydroxybenzalacetone, 7,3′-dihydroxy-5′-methoxyisoflavone and inoscavin C by the off-line semipreparative liquid chromatography-nuclear magnetic resonance protocol. In vitro, PI-PRE stimulated GLUT4 translocation by 2.34-fold and increased glucose uptake by 1.73-fold in L6 cells. However, the selective AMP-activated protein kinase (AMPK) inhibitor, compound C, completely reversed the PI-PRE-induced GLUT4 translocation. In vivo, KK-Ay mice treated with PI-PRE for four weeks had lower fasting blood glucose levels, as well as other blood–lipid indexes, compared with the vehicle control group. Mechanistic studies showed that the expressions of p-AMPKα and GLUT4 were significantly increased by treatment with PI-PRE in L6 cells. In KK-Ay mice, the expression of p-AMPKα was enhanced in the liver and skeletal muscle, and the expression of GLUT4 was increased in skeletal muscle. These findings suggest that PI-PRE possesses potential anti-diabetic effects including improving glucose tolerance, reducing hyperglycemia, and normalizing insulin levels. These effects are partly due to the activation of GLUT4 translocation via the modulation of the AMPK pathway.
Introduction
Diabetes mellitus (DM) is a serious chronic metabolic disorder that is prevalent worldwide.1 Based on a study published by the World Health Organization (WHO), the incidence of diabetes mellitus has increased rapidly in recent years. Currently, there are approximately 347 million people suffering from diabetes, and 90–95% of all diabetes patients have type 2 diabetes mellitus (T2DM).2,3 T2DM is a result of the ineffective use of insulin, or insulin resistance.3 Chronic hyperglycemia syndrome, which is a common complication of T2DM, can result in conditions such as obesity, kidney failure, fatty liver, nerve damage, hyperlipidemia, hypertension, and cardiovascular diseases. According to previous reports, the number of diabetes patients in China increased to 96 million as of 2014.2 Therefore, the development of strategies for the prevention and control of diabetes and its resulting complications is a growing need.
As a new trend in modern clinical medicine for the treatment of T2DM, functional fungi such as Ganoderma lucidum, Poria cocos Wolf, Shiraia bambusicola, Inonotus obliquus, and Grifola frondos have been attracting significant attention due to their efficacy and minimal side effects. These functional fungi have been reported to exhibit significant anti-diabetic effects.4–8 Therefore, natural remedies from functional fungi show great potential for the prevention and treatment of T2DM.
Phellinus igniarius (L.) Quail, a well-known functional fungus commonly referred to as “Sanghuang” in China, is widely consumed as a tea preparation, as an adjunct therapy or a preventive measure.9 It is a rich source of natural polyphenols, which has been used as a traditional functional food in the prevention or treatment of numerous diseases in Asia over the last two centuries.10,11 In several folk recipes, it is used to treat stomach aches and arthritis. Recent studies have demonstrated that polysaccharides isolated from P. igniarius possess anti-inflammatory, anti-tumor, anti-oxidant, and α-glucosidase inhibitory activities, and can facilitate the utilization of insulin and improve insulin resistance.12–14 Polyphenols from P. igniarius were found to exhibit anti-oxidant and cytotoxic properties, and to prevent ischemic stroke.15–17 However, the anti-diabetic effects and related mechanisms of lipophilic extracts from P. igniarius remain unclear.
Glucose transporter type 4 (GLUT4) is the major transporter in the mediation of glucose uptake in insulin sensitive cells. In the present study, a polyphenol-rich extract from P. igniarius (PI-PRE) displayed a clear stimulatory effect on GLUT4 translocation in stably transfected L6 cells expressing pIRAP-mOrange cDNA.18–20 Furthermore, PI-PRE significantly increased glucose uptake in L6 myotubes. These results suggest that PI-PRE possesses potential anti-diabetic activity. To further investigate the anti-diabetic effects of PI-PRE, this study examined the chemical profiles of the active components, and evaluated the anti-diabetic effects and the corresponding mechanisms of PI-PRE both in vitro and in vivo.
Materials and methods
Chemicals and reagents
α-Minimal essential medium (α-MEM), fetal bovine serum (FBS) and antibiotics (100 U mL−1 penicillin and 100 μg mL−1 streptomycin) were obtained from Hyclone (Logan, UT, USA). Compound C (6-[4-(2-piperidin-1-ylethoxy)phenyl]-3-pyridin-4-ylpyrazolo[1,5-a] pyrimidine, commonly used as an inhibitor of AMP-activated protein kinase (AMPK)), was purchased from Sigma-Aldrich (St Louis, MO, USA). The 2-[N-(7-nitrobenz-2-oxa-1,3-diaxol-4-yl)amino]-2-deoxyglucose (2-NBDG) assay kit was purchased from Cayman Chemical (Ann Arbor, Michigan USA). The insulin Elisa assay kits were obtained from Jiancheng Bioengineering Institute (Nanjing, Jiangsu Province, China). Triglycerides (TG), total cholesterol (TC), free fatty acid (FFA) kits were all purchased from Jiancheng Bioengineering Institute. The BCA protein quantification kit was purchased from Beyotime Biotechnology (Nantong, Jiangsu Province, China). Antibodies of β-Actin, GLUT4, AMPKα, p-AMPKα (Thr172), ACC, p-ACC and the corresponding secondary antibodies were obtained from Cell Signaling Technology (Danvers, MA, USA). The insulin and SREBP-1c antibody and the corresponding secondary antibody were obtained from Abcam (Cambridge, MA, USA). The enhanced chemiluminescence (ECL) kits were obtained from Amersham-Pharmacia (Piscataway, NJ, USA). The ECL reagent kits were from GE Healthcare BioSciences (Buckinghamshire, UK).
Preparation of PI-PRE
P. igniarius was purchased in July 2013 at Bozhou Herb Market, Bozhou, Anhui province, China, and identified by Professor Dingrong Wan of School of Pharmaceutical Sciences, South-Central University for Nationalities (SCUN), Wuhan, China. A voucher specimen (no. SC0215) is deposited in School of Pharmaceutical Sciences, SCUN. Air-dried fruiting bodies of P. igniarius (2.0 kg) were extracted sequentially by maceration at room temperature with 80% ethanol (4 × 10 L, 3 d each). The solvents were evaporated under reduced pressure and the residue (340 g) was dismissed to slurry with water (1
:
10). Then the slurry was extracted with hexane (4 × 2 L), dichloromethane (4 × 2 L), ethyl acetate (4 × 2 L) and n-BuOH (4 × 2 L), respectively. The solutions were concentrated under vacuum to yield 21 g of hexane fraction, 49 g of dichloromethane fraction, 65 g of ethyl acetate fraction and 88 g of n-BuOH fraction. The dichloromethane extract (45 g) was subjected to column chromatography of polyamide resin (450 g) eluted with 20, 40, 60, 80 and 95% aq. ethanol in a step by step manner. Different aq. ethanol elutes were combined and evaporated to dryness, which were subsequently subjected to GLUT4 translocation assay. The 80% aq. ethanol eluted fraction exhibited the strongest GLUT4 translocation assay among the collected five fractions as the purified polyphenol-rich extract (PI-PRE, 10 g). Total polyphenol content in PI-PRE was 476.8 ± 10.97 mg g−1 (gallic acid equivalents), determined according to the Folin–Ciocalteu method (see S3 in the ESI†).21 The separation of PI-PRE was carried by the procedures as follows. 100 mg of PI-PRE was dissolved in 2.0 mL of DMSO so that the concentration of PI-PRE was 50 mg mL−1, and then the solution was filtered. 200 μL of PI-PRE was subjected to semipreparative HPLC with a Waters Sunfire C18 5 μm (19 × 250 mm i.d.) HPLC column (acetonitrile in water with 0.1% formic acid from 10 to 100%, 25 min, 100% acetonitrile holding for the next 5 min). A total of 4 peak-based fractions were collected manually and 10 injections were repeated. Peaks 1, 3, and 4 were filtered through a Sephadex LH-20 column (350 × 10 mm, MeOH containing 0.1% formic acid) to yield 3 pure compounds, 1 (17.1 mg), 3 (13.1 mg), and 4 (26.8 mg). Peak 2 was purified by preparative TLC (CH2Cl2
:
MeOH
:
formic acid – 200
:
10
:
0.2) to yield compound 2 (10.3 mg). Compounds 1–4 were dissolved in MeOH-d4 or DMSO-d6 for NMR with the amount range of 4.0–6.0 mg for NMR tests.
Cell culture
α-MEM supplemented with 10% FBS and 1% antibiotics (100 U mL−1 penicillin and 100 μg mL−1 streptomycin) were used to culture L6 cells, at 37 °C within 5% CO2. After the growth up to 80 percent of culture dish, the medium were replaced with α-MEM supplemented with 2% FBS and 1% antibiotics at 37 °C within 5% CO2 to induce L6 cells to differentiate into myotubes. Myotubes were used for experiments after 5–7 days differentiation. During the culturing period, the medium was replaced every 48 h.
Fluorescence screening of GLUT4 translocation assay
L6 cells were transfected with pIRAP-mOrange cDNAs using Lipofectamine 2000 according to the manufacturers’ protocol. The methodology validation could be found in S1 in the ESI.† L6 cells stably expressing IRAP-mOrange were incubated with MEM-α supplied with 10% FBS and 1% antibiotics at 37 °C in 5% CO2. Before the experiment, L6 cells were seeded into 24-well plates for 12 h and then starved in serum-free MEM-α containing 2% fetal bovine serum and 1% antibiotics for 2 h. The cells were viewed with a confocal laser scanning microscope LSM 510 (CarlZeiss, Jena, Germany) to monitor IRAP-mOrange translocation. Images were obtained after the addition of insulin (10 nM), PI-PRE (30 μg mL−1), compound C (10 μM) or vehicle control (0.1% DMSO) for 30 min using a 555 nm excitation laser every 5 min in 30 min
Assay of glucose uptake
To determine the effects of PI-PRE in promoting the glucose uptake in L6 cells, we used a glucose uptake cell-based assay kit (Cayman Chemical, USA). All procedures were according to the manufacturer's protocols. To be brief, differentiated L6 myotubes were seeded in a 96-well black plate (1 × 104–5 × 104 cells per well) and incubated in 100 μL until confluence. Subsequently, cells were treated with different doses of PI-PRE (10, 20, 30 μg mL−1), metformin (100 μg mL−1), compound C (10 μM) or vehicle control (0.1% DMSO) in 100 μL glucose-free α-MEM medium containing 150 μg mL−1 2-NBDG. After 24 h incubation, plates were centrifuged for 5 minutes at 400g at room temperature. The supernatant was aspirated, and 200 μL of cell-based assay buffer was added into each well. This procedure was repeated twice to ensure that the cells were washed up. Then, 100 μL of assay buffer was added into each well. The 2-NBDG taken up by cells was detected with a fluorescence microplate reader (excitation/emission = 485/535 nm).
Animals and treatments
8-Week-old male KK-Ay mice (n = 62) and C57BL/6J mice (n = 10) were purchased from Beijing HFK Bioscience Co, Ltd. All animals were housed single at 22 ± 2 °C, 45–75% relative humidity with 12 h dark–light cycles and had free access to food and water under specific pathogen-free (SPF) conditions. All mice have been adapted to the laboratory conditions for one week. C57LB/6J mice (n = 10) as a normal control group were fed with a normal diet (Beijing HFK Bioscience Co., Ltd). For the sake of establishing a type 2-like diabetic mouse model, KK-Ay mice were fed with a high-fat diet which contains 40% (wt/wt) fat (Medicine Ltd, China) for 4 consecutive weeks (an average weight of 45 g). After four weeks, fasting blood glucose levels of the mice were tested. Fifty one mice whose fasted blood glucose levels were ≥11.1 mmol L−1 were classified as T2DM. Fifty one T2DM mice were randomly divided into five groups: vehicle group (n = 10), PI-PRE treatment group (40 mg per kg per day, n = 10), PI-PRE treatment group (80 mg per kg per day, n = 10), PI-PRE treatment group (160 mg per kg per day, n = 11) and metformin treatment (200 mg per kg per day, n = 10, China Associated Pharmaceutical Co., Ltd, Shenzen, China). PI-PRE was dissolved in 0.2% CMC-Na (carboxymethyl cellulose sodium) and administered to mice by oral gavage at a dose volume of 0.1 mL per 10 g body weight, whereas the mice in the vehicle group received 0.2% CMC-Na. Saline was administered orally to C57BL/6J mice as a normal control. KK-Ay mice were fed with a high-fat diet in a later study. The body weight and food intake of KK-Ay mice were measured daily.
Fasting blood glucose levels were measured weekly by using a blood glucose meter (ONETOUCH, Ultra, Lifecan, USA). An oral glucose tolerance test of mice was performed after 12 h fasting on the 26th day of treatment. Blood samples were collected from the tip of the tail at 0, 30, 60, 90 and 150 min after 2.0 g kg−1 glucose oral administration to measure the blood glucose level using a blood glucose meter (One Touch Ultra, Lifescan Inc., Wayne, USA).19
Ethics statement
The research project was approved by the Ethical Committee at SCUN and all procedures for the use and care of animals for this research were carried out under the approval by the Ethical Committee of Experimental Animal Care at SCUN (approval no. 2016-SCUEC-AEC-0037).
Biochemical analysis in serum and tissues
At the end of the experiment, all animals were fasted for 12 h. Blood samples were collected from retro-orbital sinus puncture. Then, serum was separated by centrifuging blood samples at 3000g for 15 min. The serum biochemical indexes including TC, TG, low density lipoprotein cholesterol (LDLC), and high density lipoprotein cholesterol (HDLC) were evaluated by using an automatic biochemical analyzer (Hitachi 7180+ISE, Tokyo, Japan). FFA was determined by using the corresponding assay kits. Serum insulin levels were determined by using the corresponding Elisa kits. Finally, all the mice were euthanized and livers, skeletal muscles, pancreases, white adipose tissue (WAT) and other tissues were harvested. Parts of these tissues were immediately stored in a liquid nitrogen tank, and the rest of the parts were stored in 10% neutral buffered formalin to be fixed. The tissue TC, TG and FFA in livers and skeletal muscle were determined by the method described previously.22
Histological analysis in liver, WAT and pancreas
Partial liver, pancreas and WAT were fixed with 10% neutral-buffered formalin and embedded in paraffin. 5 μm thick sections were taken with a tissue processor, and stained with HE solution for microscopy. The stained tissues were photographed through an optical microscope (200×).
Western blotting analysis in L6 cells and tissues
Plasma membrane protein of the myotubes was obtained according to the method described by previous publications.23 L6 cells were pre-incubated with different dosages of PI-PRE (10, 20, 30 μg mL−1), insulin (10 nM), compound C (10 μM), or vehicle (0.1% DMSO) for 20 min and plasma membrane proteins were extracted for western blot analysis. To analyze the total proteins in L6 cells, the cells were incubated with different dosages of PI-PRE (10, 20, 30 μg mL−1) and metformin (100 μg mL−1) for 24 hours. Subsequently, the cells in each dish were ground and lysed in ice-cold lysis buffer for 30 min to extract total proteins in each group.
Proteins in the liver and muscle were extracted according to the methods described by us previously.18,19 The western blotting procedures were according to the methods reported in our previous publications.18,19 To be brief, the protein transferred electrophoretically to the polyvinylidene fluoride (PVDF) membrane. Subsequently, the PVDF membrane was incubated in blocking buffer (5% dry fat-free dry milk in TBST) for 2 h, and then incubated overnight at 4 °C with antibodies specific for GLUT4, AMPKα, p-AMPKα (Thr172), ACC, and p-ACC diluted in 1
:
1000–5000. The membranes were washed 3 times (10 min per wash) in TBST. Immune complexes were incubated with a peroxidase-conjugated antibody diluted 1
:
5000 for 1 h. The blots were washed 3 times (10 min per wash). They were incubated in enhanced chemiluminescence kits. Immunoreactive signals were detected and quantified with the Gel Image system (APLEGEN, INC, USA).
Statistical analysis
All data were expressed as means ± standard error (M ± SEM). The results were analyzed statistically using One-way ANOVA for multiple group comparisons followed by Tukey's post hoc test. P values <0.05 were considered statistically significant. The GraphPad Prism 5.0 software package was used for statistical analyses.
Results
Chemical characterization of PI-PRE
The semipreparative HPLC chromatogram was used for the large scale preparative isolation of 4 main compounds from PI-PRE with the optimized gradient conditions (Fig. 1A). Four main peaks were collected and subjected to further purification with a Sephadex LH-20 column and preparative TLC to yield 4 pure compounds for further NMR and MS spectroscopic analysis. The 4 compounds (Fig. 1B) were identified as 7,8-dihydroxycoumarin (1),24 3,4-dihydroxybenzalacetone (2),25 7,3′-dihydroxy-5′-methoxyisoflavone (3),26 and inoscavin C (4)27 by comparison with their NMR and MS spectra with published reference data. Spectroscopic data of compounds 1–4 could be found in S6–S17 in the ESI.†
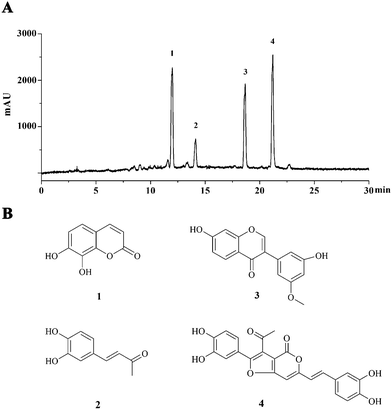 |
| Fig. 1 Major constituents of PI-PRE. (A) HPLC chromatogram of PI-PRE at 280 nm with peaks 1–4. (B) Chemical structures of compounds 1–4 purified from PI-PRE. | |
Effects of PI-PRE on GLUT4 translocation in L6 cells
We used a L6 cell line which stably expressed IRAP-mOrange to evaluate the effects of PI-PRE on GLUT4 translocation. Following incubation with PI-PRE, the intensity of fluorescence in L6 cell membranes increased steadily in a time-dependent manner. As shown in Fig. 2A and B, the fluorescence reached the greatest intensity (2.34-fold) at 20 minutes after the addition of PI-PRE. These results showed that PI-PRE significantly affected GLUT4 translocation in L6 cells. However, this effect was significantly inhibited by treatment with the AMPK inhibitor, compound C (Fig. 2B).
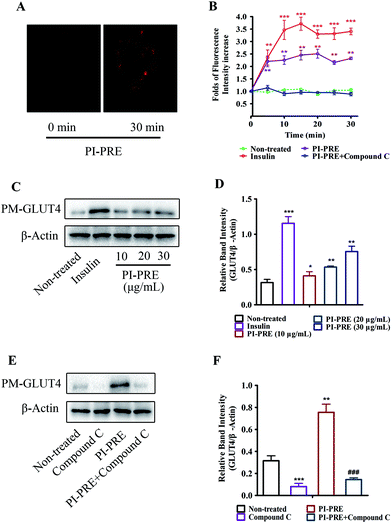 |
| Fig. 2 Effects of PI-PRE on stimulating GLUT4 translocation in L6 cells. (A) Images of PI-PRE stimulating the GLUT4 translocation in L6 cells. (B) Data represent the fold increase in fluorescence induced by different test samples between 0 and 30 min (**P < 0.01, compared with non-treated groups; ***P < 0.001, compared with non-treated groups). (C) Effects of PI-PRE on PM-GLUT4 levels in L6 cells. (D) Relative band intensity of PM-GLUT4 in the image (C) (*P < 0.05, compared with non-treated groups; **P < 0.01, compared with non-treated groups; ***P < 0.001, compared with non-treated groups). (E) Effects of compound C on inhibiting the PM-GLUT4 level in L6 cells. (G) Relative band intensity of GLUT4 in each group (**P < 0.01, compared with non-treated groups; ***P < 0.001, compared with the non-treated group; ###P < 0.001, compared with the PI-PRE treated group). | |
In addition, the results of western blotting for GLUT4 protein expression in the plasma membrane (PM-GLUT4) showed that PI-PRE exhibited the same enhancing effects for GLUT4 translocation activity. As shown in Fig. 2C and D, the levels of PM-GLUT4 were obviously increased following stimulation with different concentrations of PI-PRE. At a dose of 30 μg mL−1, PI-PRE displayed the strongest stimulatory effect on GLUT4 translocation to the plasma membrane (PM). Consistent with the fluorescence screening results in Fig. 2A and B, the increase in PM-GLUT4 induced by PI-PRE was reversed by the addition of compound C (Fig. 2E and F).
Effects of PI-PRE on glucose uptake, GLUT4 expression, and AMPK phosphorylation in L6 cells
As shown in Fig. 3A and B, PI-PRE increased GLUT4 expression in L6 cells. The GLUT4 expression level was increased by 3.6-fold at 30 μg mL−1 compared to non-treated cells. When L6 cells were exposed to PI-PRE and metformin, the ratio of p-AMPKα and AMPK was significantly up-regulated in comparison with non-treated cells. The ratio of p-AMPKα/AMPK increased in a dose-dependent manner with respect to PI-PRE treatment. At a dosage of 30 μg mL−1, PI-PRE exhibited the greatest effect on increasing the ratio of p-AMPKα/AMPK (approximately 2.7-fold compared with non-treated cells).
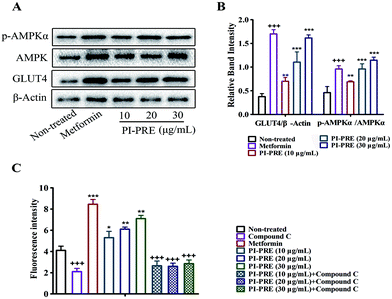 |
| Fig. 3 (A) Effects of PI-PRE on GLUT4 expression and AMPK phosphorylation in L6 myotubes. (B) Relative band intensity of GLUT4 and p-AMPKα (+++P < 0.001, compared with the non-treated group; **P < 0.01, compared with non-treated groups; ***P < 0.001, compared with the non-treated group). (C) Effects of PI-PRE on glucose uptake in L6 cells (+++P < 0.001, compared with the non-treated group; *P < 0.05, compared with non-treated groups; **P < 0.01, compared with non-treated groups; ***P < 0.001, compared with the non-treated group). | |
Metformin induced a significant increase in glucose uptake in L6 cells, as is evidenced by the increasing intensity of fluorescence compared to untreated cells (Fig. 3C). L6 cells treated with PI-PRE also showed a significant increase in glucose uptake, the highest dose (30 μg mL−1) increased glucose uptake by 1.73-fold. Interestingly, these effects were inhibited by the addition of compound C. These combined in vitro results suggest that PI-PRE may enhance GLUT4 expression and glucose uptake in L6 cells by targeting the AMPK signaling pathway.
Effects of PI-PRE on body weight, food intake, fasting blood glucose, and oral glucose tolerance in KK-Ay mice
The anti-diabetic effects of PI-PRE were investigated in vivo using KK-Ay mice, a model for type 2 diabetes. Prior to the experiment, blood glucose levels and body weights of KK-Ay mice were measurably higher than those of normal C57BL/6J mice. After 4 weeks of treatment, the body weights and fasting blood glucose levels were significantly reduced in the PI-PRE treated group, indicating that PI-PRE improved glucose and lipid metabolism in KK-Ay mice (Fig. 4A and B). During the 4 weeks of PI-PRE administration, there was no significant difference in food intake between the vehicle control group and the PI-PRE treated groups (Fig. 4C). On the 26th day of treatment, the oral glucose tolerance test (OGTT) was carried out. As shown in Fig. 4D and E, oral glucose tolerance in mice treated with PI-PRE or metformin has been normalized.
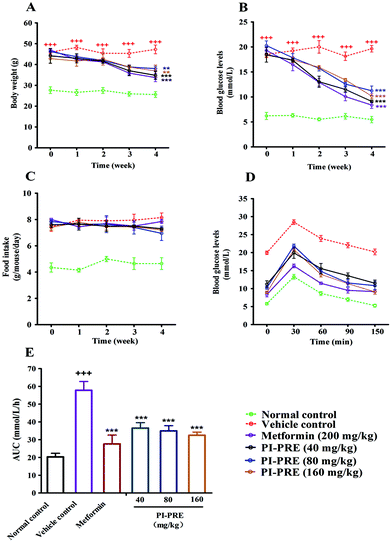 |
| Fig. 4 Effects of PI-PRE on body weight, food intake, fasting blood glucose, and oral glucose tolerance in KK-Ay mice. (A) Effects of PI-PRE on reducing the body weight. (B) Effects of PI-PRE on food intake. (C) Effects of PI-PRE on reducing fasting blood glucose levels. (D) Effects of PI-PRE on oral glucose tolerance. (+++P < 0.001, ++P < 0.01, +P < 0.05, compared to normal control; ***P < 0.001, **P < 0.01, *P < 0.05, compared to vehicle control). | |
Effects of PI-PRE on serum and tissue lipid levels in KK-Ay mice
Insulin resistance and T2DM are accompanied by dyslipidemia, which is characterized by high LDLC, TG, TC, and FFA as well as low HDLC. Many studies have emphasized that hyperinsulinemia and dyslipidemia are significant causes of T2DM.28
To investigate the effects of PI-PRE on lipid metabolism and insulin resistance, blood samples and tissues were collected to measure the related lipid content and serum insulin levels. Compared with the vehicle group, PI-PRE significantly decreased LDL-C, TG, TC, and FFA levels in a dose-dependent manner from 40 to 160 mg kg−1 (Fig. 5A–C and E). In addition, HDL-C levels increased in a dose-dependent manner following PI-PRE treatment (Fig. 5D), and serum insulin levels were decreased after the treatment of PI-PRE and metformin, in comparison with the vehicle control (Fig. 5F).
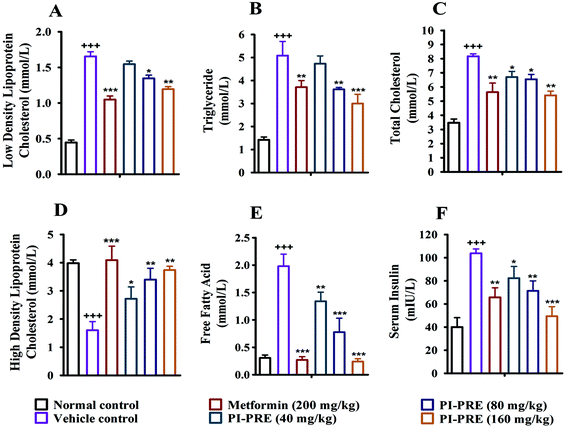 |
| Fig. 5 Effects of PI-PRE on serum glycolipid metabolism. (A–E) Effects of PI-PRE on LDLC, TG, TC, HDLC, and FFA in the serum of mice. (F) Effects of PI-PRE on serum insulin concentration (+++P < 0.001, ++P < 0.01, +P < 0.05, compared to normal control. ***P < 0.001, **P < 0.01, *P < 0.05, compared to the vehicle group). | |
In addition, the contents of TC, TG and FFA in the liver and skeletal muscle tissues were also analyzed. As shown in Fig. 6, the TG, TC, and FFA levels in the liver and skeletal muscle in the vehicle group with T2DM were measurably higher than in normal controls. Nevertheless, the contents of these lipids in the liver and skeletal muscle were decreased following PI-PRE treatment. These data show that PI-PRE possesses the capability to normalize lipid metabolism and decrease insulin resistance.
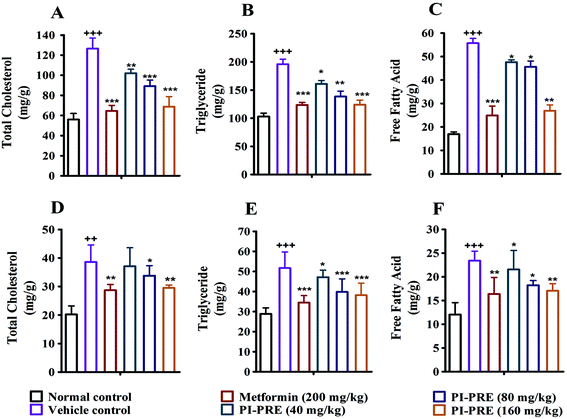 |
| Fig. 6 Tissue lipid content in the liver and muscle of KK-Ay mice was measured after treatment for 4 weeks. TC (A), TG (B) and FFA (C) levels in mice liver; TC (D), TG (E) and FFA (F) levels in mice skeletal muscle (+++P < 0.001, ++P < 0.01, +P < 0.05, compared to normal control; ***P < 0.001, **P < 0.01, *P < 0.05, compared to vehicle control). | |
Histological changes in the pancreas, WAT, and liver of KK-Ay mice
To determine whether PI-PRE has the capacity to protect the pancreas, adipose tissue, and liver in KK-Ay mice, we performed histological examination in these tissues. As shown in Fig. 7A, the livers of KK-Ay mice in the vehicle group presented with hepatic steatosis and empty lipid vacuoles. After oral administration of PI-PRE for 4 weeks, hepatic steatosis and liver damage were reversed, particularly in the 160 mg kg−1 PI-PRE group. As shown in Fig. 7B, adipose cells were larger and of abnormal shape in KK-Ay mice when compared with the normal control group. After 4 weeks of treatment with PI-PRE, the size of adipocyte cells in WAT was obviously reduced in KK-Ay mice. Additionally, PI-PRE alleviated the abnormality of pancreatic β-cells caused by diabetes in KK-Ay mice (Fig. 7C). These results indicate that PI-PRE can effectively alleviate diabetic complications in KK-Ay mice.
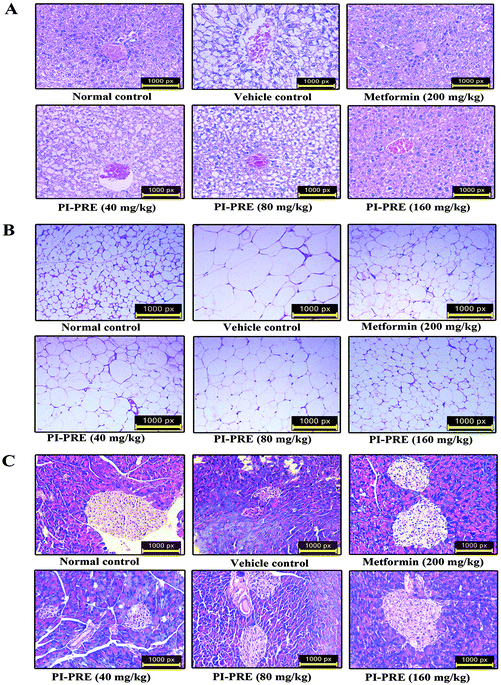 |
| Fig. 7 Effects of PI-PRE on the histological changes of related tissues in KK-Ay mice. Optical microscopy: HE (200×). (A) Effects of PI-PRE on the morphological features of the liver. (B) Effects of PI-PRE on the morphological features of WAT. (C) Effects of PI-PRE on the morphological features of the pancreas. | |
Western blot analysis on tissues
The ability of PI-PRE to enhance GLUT4 translocation and glucose uptake through the AMPK-GLUT4 pathway was studied in L6 cells (Fig. 2 and 3). To investigate whether the hypoglycemic effect of PI-PRE was modulated through the AMPK-GLUT4 pathway in vivo, expression levels of key proteins related to the regulation of glucose metabolism in muscle and liver tissues were analyzed by western blotting. The expression of GLUT4 and the phosphorylation of AMPK in skeletal muscle, as well as the expression of phosphorylated AMPK (p-AMPK) and phosphorylated acetyl-Co-A carboxylase (p-ACC) in the liver were determined. Following four weeks of treatment, PI-PRE significantly increased the expression of GLUT4 and p-AMPK in the skeletal muscles of T2DM mice in a dose-dependent manner, compared to the vehicle control group (Fig. 8A and B). In addition, the 4 week-treatment of PI-PRE dose-dependently up-regulated the phosphorylation of AMPK and ACC in the liver, compared with the vehicle control group (Fig. 8C). Furthermore, the ratio of p-ACC/ACC was also significantly increased in the liver (Fig. 8D). Altogether, these results indicate that PI-PRE improved glucose metabolism via the AMPK-GLUT4 signaling pathway, and promoted lipid metabolism via the AMPK-ACC signaling pathway.
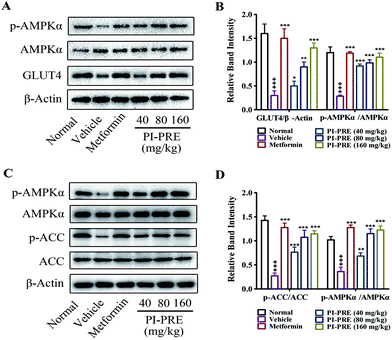 |
| Fig. 8 Effects of PI-PRE on related proteins in the liver and skeletal muscle of KK-Ay mice. (A) Effects of PI-PRE on the expressions of p-AMPKα and GLUT4 in skeletal muscle. (B) Relative band intensity of GLUT4 and p-AMPKα in the skeletal muscle. (C) Effects of PI-PRE on the expressions of p-AMPKα and p-ACC in the liver. (D) Relative band intensity of p-AMPKα and p-ACC in the liver (+++P < 0.001, compared to normal control; ***P < 0.001, **P < 0.01, *P < 0.05, compared to vehicle control). | |
Discussion
T2DM is a common form of diabetes which is caused by insufficient use of insulin, and eventually leads to hyperglycemia.1 Hyperglycemia not only influences normal functions in the body, but also results in micro-vascular and macro-vascular dysfunction in diabetics.29 Currently available anti-diabetic agents include sulphonylureas and the rapidly-acting secretagogues which stimulate insulin secretion, biguanides which reduce hepatic glucose production, α-glucosidase inhibitors which delay digestion and absorption, and thiazolidinediones which improve insulin action. Although there are a growing number of related drugs to treat T2DM, many of these result in drug tolerance and severe secondary side-effects such as hepatic disease.30,31Many studies have shown that functional fungi often exhibit beneficial effects on glucose and lipid metabolism in experimental diabetic models and have minimal side effects.4–8
Phellinus igniarius, an edible and medicinal fungus consumed mainly as a tea preparation, has been long used as a traditional Chinese medicine for the treatment of a variety of diseases.32 In the present study, the antidiabetic activity of the P. igniarius extract was investigated in vitro and in vivo. In vitro, PI-PRE enhanced GLUT4 translocation and glucose uptake in L6 cells. In vivo, the type 2 diabetic phenotype of the KK-Ay diabetic mouse model was significantly alleviated by PI-PRE treatment. These antidiabetic effects included significantly decreased fasting glucose levels and body weight, which were observed in the PI-PRE groups during the 4-week experiment. These data suggest that PI-PRE possesses potential antidiabetic effects.
Insulin resistance is a key characteristic of T2DM, and ameliorating the state of insulin resistance is beneficial for individuals with T2DM.33,34 In the current study, KK-Ay mice exhibited symptoms of hyperinsulinemia, indicating that the mice developed insulin resistance. Interestingly, PI-PRE treatment significantly reduced serum insulin levels and improved oral glucose tolerance in diabetic mice. As a result, PI-PRE-treated KK-Ay mice had significantly lower blood glucose levels than those in the vehicle group at each time point during the OGTT (Fig. 4E and F). Furthermore, PI-PRE treatment had a significant protective effect on pancreatic β-cells in KK-Ay mice (Fig. 7C). These results suggest that PI-PRE improved carbohydrate metabolism in diabetic KK-Ay mice by ameliorating insulin resistance.
GLUT4 is a key regulator or mediator of glucose removal from the circulation and a key regulator of whole-body glucose homeostasis.36 GLUT4 is mainly expressed in adipose tissue and skeletal muscle and can catalyze hexose transport across cell membranes.28 There is evidence that increasing the translocation and expression of GLUT4 can reduce insulin resistance and promote glucose uptake.37 In the in vitro study, PI-PRE significantly promoted GLUT4 translocation to the PM in L6 cells (Fig. 2). PI-PRE significantly increased GLUT4 expression and glucose uptake in L6 cells (Fig. 3). These data revealed a possible mechanism for PI-PRE-induced amelioration of insulin resistance. Specifically, PI-PRE may increase GLUT4 translocation and expression to improve the symptoms of insulin resistance. To confirm this assumption, GLUT4 expression in the skeletal muscle of KK-Ay mice after 4 weeks of treatment with PI-PRE was analyzed. The in vivo results were consistent with the in vitro data. During the 4-week treatment with PI-PRE, GLUT4 expression in skeletal muscle was increased significantly compared with the vehicle control group (Fig. 8A and B). The in vitro and in vivo results confirm the previous hypothesis that PI-PRE can alleviate insulin resistance by increasing GLUT4 expression and translocation in diabetic KK-Ay mice.
GLUT4 is mainly regulated by two signaling pathways, AMPK and phosphatidylinositol-3 kinase (PI3K).35 In our study, proteins upstream of GLUT4 were investigated to determine which pathway is involved in the PI-PRE-induced upregulation of GLUT4 expression and the increase in GLUT4 translocation. The results showed that PI-PRE-induced GLUT4 translocation was completely inhibited by compound C, suggesting that PI-PRE stimulated GLUT4 translocation via the AMPK pathway (Fig. 2B, E and F). Furthermore, western blotting analysis showed that PI-PRE promoted AMPK phosphorylation not only in L6 cells but also in the liver and skeletal muscle of KK-Ay mice. It can be concluded that PI-PRE improved insulin resistance in part at least, by activating the AMPK-GLUT4 pathway.
Lipid plays an important role in the pathogenesis of T2DM.28 Lipid metabolism disorders including obesity, hyperlipidemia, and non-alcoholic fatty liver disease are always accompanied by T2DM.37 In this study, elevated body weight, serum lipids (TG, TC, LDL-C, FFA) and reduced HDL-C were observed in diabetic KK-Ay mice. Following 4 weeks of treatment with PI-PRE, the body weight and serum TG, TC, LDL-C and FFA levels in KK-Ay mice were reduced while HDL-C levels were significantly increased, compared with the vehicle group (Fig. 4A and 5). In addition, lipid accumulation in the liver and the adipocyte size in WAT were significantly reduced in the PI-PRE-treated groups (Fig. 7A and B). TG, TC, and FFA levels in the liver and skeletal muscle were significantly reduced in a dose-dependent manner by PI-PRE treatment (Fig. 6). These data show that PI-PRE improved lipid metabolism and reduced fatty liver in a mouse model of T2DM. Regarding the molecular mechanism through which PI-PRE improved lipid metabolism, the activation of AMPK contributed to this effect.
AMP-activated protein kinase (AMPK) is a metabolic sensor that plays an important role in whole body energy balance, such as food intake, body weight, glucose uptake and lipid metabolism.38 The activation of AMPK may result in the increased levels of GLUT4 and enhanced phosphorylation of ACC, which is a key biosynthetic precursor to fatty acids as well as a potent inhibitor of fatty acid oxidation in the mitochondria.39,40 ACC is inhibited by AMPK through phosphorylation, which leads to a decrease in malonyl-CoA content and triglyceride synthesis, and an increase in β-oxidation.41 The present study showed that the expression levels of p-ACC were increased measurably in the livers of PI-PRE treated mice compared with those of the vehicle group (Fig. 8B). Comprehensive consideration of the increased phosphorylation of ACC and AMPK in the liver suggests that PI-PRE activated the AMPK-ACC signaling pathway, thereby suppressing the ACC activity and further reducing the free fatty acid synthesis in the liver, resulting in improved hepatic lipid metabolism.
In conclusion, these results indicate that PI-PRE activates the AMPK/GLUT4 and the AMPK/ACC signaling pathways, thereby alleviating insulin resistance and improving glucose and lipid metabolism in type 2 diabetes.
Conclusion
PI-PRE, a polyphenol rich extract from P. igniarius, exhibited anti-diabetic effects in vitro and in vivo. In vitro, PI-PRE significantly stimulated GLUT4 translocation by 2.34-fold and significantly increased glucose uptake by 1.73-fold in L6 cells. In vivo, PI-PRE significantly improved oral glucose tolerance and decreased insulin resistance, increased serum HDL-C, and reduced the body weight, blood glucose levels, and serum TC, TG, FFA, and LDL-C levels. The levels of TG, TC and FFA in the liver and skeletal muscle were markedly reduced after 4-weeks of treatment. The mechanism of the antidiabetic activity of PI-PRE was determined to be through the modulation of the AMPK-GLUT4 and AMPK-ACC signaling pathways. These results suggest that PI-PRE has the potential to become an effective agent in the therapy of T2DM.
Conflicts of interest
The authors declare that they have no conflict of interest.
Acknowledgements
The work was financially supported by the National Natural Science Foundation of China (grants 81573561 and 81774000), the Wuhan Applied Basic Research Program of Science and Technology (No. 2017060201010217), and the Fundamental Research Funds for the Central Universities, South-Central University for Nationalities (CZP17060).
References
- C. M. Clark Jr. and L. D. Anthony, N. Engl. J. Med., 1995, 332, 1210–1217 CrossRef CAS PubMed.
- L. Guariguata, D. R. Whiting, I. Hambleton, J. Beagley, U. Linnenkamp and J. E. Shaw, Diabetes Res. Clin. Pract., 2014, 103, 137–149 CrossRef CAS PubMed.
- X. Z. Yang, J. Yang, C. Xu, M. Huang, Q. Zhou, J. N. Lv, X. H. Ma, C. Q. Ke, Y. Ye, G. W. Shu and P. Zhao, J. Ethnopharmacol., 2015, 171, 161–170 CrossRef CAS PubMed.
- C. Xiao, Q. Wu, J. Zhang, Y. Xie, W. Cai and J. Tan, J. Ethnopharmacol., 2017, 196, 47–57 CrossRef CAS PubMed.
- T. H. Li, C. C. Hou, C. L. Chang and W. C. Yang, Evid. Based Complement. Alternat. Med., 2011, 2011 DOI:10.1155/2011/128402.
- M. Huang, P. Zhao, M. R. Xiong, Q. Zhou, S. J. Zheng, X. H. Ma, C. Xu, J. Yang, X. Z. Yang and T. C. Zhang, J. Ethnopharmacol., 2016, 191, 71–81 CrossRef CAS PubMed.
- Y. M. Ying, L. Y. Zhang, X. Zhang, H. B. Bai, D. E. Liang, L. F. Ma, W. G. Shan and Z. J. Zhan, Phytochemistry, 2014, 108, 171–176 CrossRef CAS PubMed.
- C. Xiao, Q. Wu, Y. Xie, J. Zhang and J. Tan, Food Funct., 2015, 6, 3567–3575 CAS.
- Z. T. Shi and H. Y. Bao, Chin. J. Exp. Tradit. Med. Formulae, 2016, 22, 197–202 Search PubMed.
- Y. Wang, X. Shang, S. Wang, S. Mo, S. Li, Y. C. Yang, F. Ye, J. G. Shi and L. He, J. Nat. Prod., 2007, 70, 296–299 CrossRef CAS PubMed.
- Y. Wang, S. J. Wang, S. Y. Mo, S. Li, Y. C. Yang and J. G. Shi, Org. Lett., 2005, 7, 4733–4736 CrossRef CAS PubMed.
- P. Suabjakyong, K. Nishimura, T. Toida and L. J. Van Griensven, Food Funct., 2015, 6, 2834–2844 CAS.
- L. Chen, J. Pan, X. Li, Y. Zhou, Q. Meng and Q. Wang, Int. Immunopharmacol., 2011, 11, 255–259 CrossRef CAS PubMed.
- C. Xiao, Q. P. Wu, J. B. Tan, W. Cai, X. B. Yang and J. M. Zhang, J. Med. Plants Res., 2011, 32, 6963–6967 Search PubMed.
- I. K. Lee and B. S. Yun, J. Antibiot., 2011, 64, 349–359 CrossRef CAS PubMed.
- M. Y. Lung, J. C. Tsai and P. C. Huang, J. Food Sci., 2010, 75, E18–E24 CrossRef CAS PubMed.
- P. Suabjakyong, R. Saiki, L. J. Van Griensven, K. Higashi, K. Lgarashi and T. Toida, PLoS One, 2015, 10, e0122733, DOI:10.1371/journal.pone.0122733.
- X. Z. Yang, S. H. Deng, M. Huang, J. L. Wang, L. Chen, M. R. Xiong, J. Yang, S. J. Zheng, X. H. Ma, P. Zhao and Y. J. Feng, Bioorg. Med. Chem. Lett., 2017, 27, 1463–1466 CrossRef CAS PubMed.
- M. Huang, S. H. Deng, Q. Q. Han, P. Zhao, Q. Zhou, S. J. Zheng, X. H. Ma, C. Xu, J. Yang and X. Z. Yang, Front. Pharmacol., 2016, 7, 288, DOI:10.3389/fphar.2016.00288.
- C. Wang, J. Yang, P. Zhao, Q. Zhou, Z. N. Mei, G. Z. Yang, X. Z. Yang and Y. J. Feng, Bioorg. Med. Chem. Lett., 2014, 24, 3096–3099 CrossRef CAS PubMed.
- M. Quiñones, M. Miguel, B. Muguerza and A. Aleixandre, Food Funct., 2011, 11, 649–653 Search PubMed.
- Y. S. Lee, W. S. Kim, K. H. Kim, M. J. Yoon, H. J. Cho, Y. Shen, J. M. Ye, C. H. Lee, W. K. Oh, C. T. Kim, C. Hohnen-Behrens, A. Gosby, E. W. Kraegen, D. E. James and J. B. Kim, Diabetes, 2006, 55, 2256–2264 CrossRef CAS PubMed.
- S. Nishiumi and H. Ashida, Biosci., Biotechnol., Biochem., 2007, 71, 2343–2346 CrossRef CAS PubMed.
- B. Ma, H. F. Guo and H. X. Lou, Helv. Chim. Acta, 2007, 90, 58–62 CrossRef CAS.
- Y. J. Kim, J. Park, B. S. Min and S. H. Shim, J. Korean Soc. Appl. Biol. Chem., 2011, 54, 287–294 CAS.
- X. Z. Yang, J. N. Liu, C. Xu, Q. X. Lin, J. Yang, C. Wang and P. Song, J. Yunnan. Univ., 2015, 37, 134–139 Search PubMed.
- L. F. Zan, H. Y. Bao, T. Bau and Y. Li, Nat. Prod. Commun., 2015, 10, 315–316 Search PubMed.
- R. A. DeFronzo and E. Ferrannini, Diabetes Care, 1991, 14, 173–194 CrossRef CAS PubMed.
- UK Prospective Diabetes Study Group, Br. Med. J., 1998, 317(7160), 703–713 CrossRef.
- D. L. Browne, L. Avery, B. C. Turner, D. Kerr and D. A. Cavan, Diabetic Med., 2000, 17, 528–531 CrossRef CAS PubMed.
- S. Betsy, R. Debra, C. Betty and S. Bonnie, Med. Care, 1999, 37, 1169–1173 CrossRef.
- Y. Lv, J. Wang, Y. Li, M. Du, F. Qin, K. Gao and X. H. Song, Sci. Seric., 2009, 35, 204–210 Search PubMed.
- A. R. Saltiel and C. R. Kahn, Nature, 2001, 414, 799–806 CrossRef CAS PubMed.
- B. J. Goldstein, Am. J. Cardiol., 2002, 90, 3–10 CrossRef.
- S. Huang and M. P. Czech, Cell Metab., 2007, 5, 237–252 CrossRef CAS PubMed.
- A. Biswas, S. Bhattacharya, S. Dasgupta, R. Kundu, S. S. Roy, B. C. Pal and S. Bhattacharya, Mol. Cell. Biochem., 2010, 336, 977–107 CrossRef PubMed.
- G. Karimi, M. R. Sabran, R. Jamaluddin, K. Parvaneh, N. Mohtarrudin, Z. Ahmad, H. Khazaai and A. Khodavandi, Food Nutr. Res., 2015, 59, 29273, DOI:10.3402/fnr.v59.29273.
- D. G. Hardie, J. W. Scott, D. A. Pan and E. R. Hudson, FEBS Lett., 2003, 546, 113–120 CrossRef CAS PubMed.
- N. B. Ruderman, A. K. Saha and E. W. Kraegen, Endocrinology, 2003, 44, 5166–5171 CrossRef PubMed.
- E. J. Kurth-Kraczek, M. F. Hirshman, L. J. Goodyear and W. W. Winder, Diabetes, 1999, 148, 1667–1671 CrossRef.
- C. A. Carlson and K. Kim, J. Biol. Chem., 1973, 248, 378–380 CAS.
Footnotes |
† Electronic supplementary information (ESI) available. See DOI: 10.1039/c7fo01460k |
‡ Both authors contributed equally to this paper. |
|
This journal is © The Royal Society of Chemistry 2018 |
Click here to see how this site uses Cookies. View our privacy policy here.