DOI:
10.1039/C7FO01549F
(Paper)
Food Funct., 2018,
9, 636-642
An altered tissue distribution of flaxseed lignans and their metabolites in Abcg2 knockout mice†
Received
6th October 2017
, Accepted 9th December 2017
First published on 11th December 2017
Abstract
Lignans are dietary polyphenols, which are metabolized by gut microbiota into the phytoestrogenic metabolites enterolignans, mainly enterolactone and enterodiol. Breast Cancer Resistance Protein (BCRP/ABCG2) is an efflux transporter that affects the plasma and milk secretion of several drugs and natural compounds. We hypothesized here that Abcg2 could influence the levels of lignans and their derived metabolites in target tissues. Consequently, we aimed to evaluate the role of Abcg2 in the tissue distribution of these compounds. We used Abcg2−/− knockout and wild-type male mice fed with a lignan-enriched diet for one week and analysed their plasma, small intestine, colon, liver, kidneys and testicles. High levels of lignans as well as enterolignans and their glucuronide and sulfate conjugates in the small intestine and colon were detected, with higher concentrations of the conjugates in the wild-type compared with Abcg2−/− mice. Particularly relevant was the detection of 24-fold and 8-fold higher concentrations of enterolactone-sulfate and enterolactone-glucuronide, respectively, in the kidney of Abcg2−/− compared with wild-type mice. In conclusion, our study showed that lignans and their derived metabolites were in vivo substrates of Abcg2, which affected their plasma and tissue levels. These results highlight the role of Abcg2 in influencing the health-beneficial properties of dietary lignans.
Introduction
Lignans are polyphenols found in measurable quantities in a wide variety of plant foods, with biological activities encompassing many areas, including antioxidant, anti-inflammatory and antitumor properties.1 One of the richest dietary sources of lignans is flaxseed (Linum usitatissimum).2 Flaxseed-enriched diets have been associated with a protective role in several diseases, with reductions in risk of cardiovascular disease, osteoporosis, diabetes, renal disease, and prostate and breast cancer.1,3 The predominant lignan in flaxseed is secoisolariciresinol diglucoside (SDG), which is metabolized by the intestinal microbiota via secoisolariciresinol (SECO) into the phytoestrogenic enterolignans enterodiol (END) and enterolactone (ENL). These compounds are efficiently conjugated with glucuronic acid or, to a lesser extent, with sulfate groups in the intestinal epithelium and in the liver, with enterohepatic recycling.1,4
The human Breast Cancer Resistance Protein (BCRP/ABCG2) is a membrane transporter (named Abcg2 in mice) and constitutes one of the potential factors that may affect the distribution of these compounds in the organism. It is an efflux protein with an important capacity to limit the bioavailability of multiple compounds. Recently, its high resolution structure has been reported and the basis of its multidrug recognition revealed.5 It is expressed in enterocytes, in the canalicular bile epithelia, in the renal proximal tubular cells and in the endothelial cells of the blood capillaries in the brain and testis, protecting the organism from potentially toxic compounds, and thereby limiting their absorption and mediating their distribution and excretion.6 In addition to transporting drugs and toxins, ABCG2 affects the disposition of endogenous and natural compounds including phytoestrogens.7–9 Some of its genetic variants can lead to an impaired transport of its substrates, thus affecting their bioavailability. For instance, in addition to being linked to gout, due to affecting uric acid excretion,10 the Q141 K variant alters the pharmacokinetics of several marketed drugs that are ABCG2 substrates.11
Previous studies from our group have reported that ENL, END and SECO are in vitro substrates of murine Abcg2 and human ABCG2 in transepithelial transport experiments using MDCKII cells.12,13 Regarding the conjugates, enterolignan sulfates have been described as ABCG2 substrates,14 and recently, an important role of Abcg2/ABCG2 in the transport of phytoestrogen glucuronides has been reported.15 Our previous experiments using female wild-type and Abcg2−/− mice showed an important role of this transporter in the levels of enterolignans in plasma, milk and mammary gland.12,13
However, the effect of Abcg2/ABCG2 on the distribution of these compounds in other target organs, essential for their beneficial effects, remains unexplored. We aimed therefore to evaluate the plasma and tissue distribution of flaxseed lignans, their microbial-derived enterolignans ENL, END and SECO as well as their conjugated metabolites in wild-type and Abcg2−/− knockout male mice after the administration of a flaxseed-enriched diet for 7 days.
Materials and methods
General experimental procedures
SDG, SECO, ENL and END were purchased from Sigma-Aldrich (St Louis, MO, USA). The flaxseed hull extract (LinumLife®EXTRA, Frutarom, Ltd, Herzeliya, Israel) was provided by Tradichem S.L. (Madrid, Spain) and the mice diet was formulated by Research Diets, Inc (New Brunswick, NY, USA). All the other chemicals were of analytical grade and obtained from commercial sources.
Animal experiment
Animals were housed and handled according to institutional guidelines complying with European legislation (2010/63/EU). Experimental procedures were approved by the Animal Care and Use Committee of the University of Leon and the Junta de Castilla y Leon (ULE_001_2015). Abcg2−/− and wild-type male mice aged between 9 and 13 weeks, all of them >99% FVB genetic background (n = 4–5), were kindly provided by Dr. A. H. Schinkel (The Netherlands Cancer Institute, Amsterdam, the Netherlands), and were kept in a temperature-controlled environment with a 12-hour light/12-hour dark cycle. Mice were fed normal, nutritionally balanced rodent free-of-soybean meal feed for 7 days. This diet was supplemented with 1% of lignan-rich extract from flaxseed hulls (LinumLife®EXTRA). The SDG content was 2 mg g−1 diet, and the mean ingestion of SDG was approximately 10 mg per mouse each day. LinumLife®EXTRA does not contain SECO, ENL or END. Other lignans present were matairesinol (MAT), isolariciresinol (isoLARI), pinoresinol (PINO), and lariciresinol (LARI). Phenolic compounds including p-coumaric acid, ferulic acid, sinapic acid, caffeic acid and their glucosides, as well as the flavonoids herbacetin diglucoside (HDG) and kaempferol diglucoside (KDG) have also been identified.
After 7 days, blood was collected by cardiac puncture under anesthesia with isoflurane and the organs were harvested after euthanasia by cervical dislocation. Heparinized blood samples were centrifuged immediately at 1000g for 10 min. Samples were stored at −20 °C until their extraction and further UPLC-QTOF-MS analysis.
Sample extraction
Tissue samples were homogenized with sodium acetate buffer (pH 5): liver, testis and kidney samples at 1 g per 4 mL, small intestine and colon samples at 1 g per 2 mL, and small intestinal content samples at 1 g per 12 mL. Plasma (200 μL) was mixed with 200 μL of the same buffer. This mixture and 200 μL of homogenized tissue samples were treated with 200 mM of hydrochloric acid in methanol (400 μL and 800 μL, respectively), following the method of Bolca et al.16 After vortexing for 10 min and centrifugation at 18
000g for 10 min, the supernatant was evaporated with N2 and the dry residue was re-dissolved in methanol (200 μL) and filtered (0.22 μm) prior to injection into the LC-MS system.
UPLC-qTOF-MS analysis
Metabolite analyses were carried out using an Agilent 1290 Infinity LC system coupled to a 6550 Accurate-Mass qTOF (Agilent Technologies, Waldbronn, Germany) and using an electrospray interface (Jet Stream Technology). The detailed separation and detection are described elsewhere.13 Briefly, separation was achieved on a Poroshell 120 EC-C18 column (3 × 100 mm, 2.7 μm) (Agilent Technologies) at 30 °C. Elution solvents were water (A) and acetonitrile (B), both with 0.1% formic acid with the following gradient: 5–25% B at 0–10 min, 25–40% B at 10–20 min; 40–90% B at 20–24 min; 90%–5% at 25–26 min and the column re-equilibrated for 4 min. The flow rate was 0.4 mL min−1 and the injected volume was 3 μL. Nitrogen was used as a nebulizing (9 L min−1, 35 psi) and drying gas (280 °C). Experiments were performed in the negative ion mode with a scan range of 100–1100. Data were processed using the Mass Hunter Qualitative Analysis software (B.06.00). A targeted analysis was performed to look for specific metabolites of lignans (SECO, END and ENL) as well as their glucuronide and sulfate conjugates. Screening was based on mass filtering at the exact mass using a narrow mass extraction window (0.01 m/z). SDG, SECO, END and ENL were quantified by peak area integration of their extracted ion chromatograms (EIC) using calibration curves with their corresponding authentic standards (linearity until 0.5 μM for END and ENL and 2 μM for SDG and SECO).13 LODs were 2.10, 2.80, 0.13 and 0.05 nM for SDG, SECO, END and ENL, respectively, and LOQs were 7.0, 9.3, 0.42 and 0.18 nM, respectively. Due to the absence of available standards for glucuronide and sulfate conjugates, an accurate quantification was not possible. In this case, the area under each extracted ion chromatogram (EIC) (peak area) was used. The corresponding values were corrected taking into account the weight of the sample, extraction volume and dilution.
Statistical analysis
The Mann–Whitney U test was used to analyze the statistical significance of differences between the mean values of variables. All analyses were carried out on the assumed significance level of α = 0.05 using Statistica 10.0 software. The results are shown as mean ± standard deviation (SD).
Results and discussion
Flaxseed-enriched diets contain elevated concentrations of lignans, including SDG, which guarantee the production of high concentrations of the gut microbiota-derived enterolignans in the organism.1 Concentrations achieved in plasma and target tissues are essential for their biological activities. Our data analysis of male mice fed with the flaxseed-enriched diet for 7 days identified ENL, END, SECO, SDG and different enterolignan metabolites by UPLC-qTOF-MS in plasma and tissues (Table 1 and Fig. 1–3). Glucuronide and sulfate conjugates were the predominant metabolites, although enterofurans and their glucuronide metabolites were also detected (Table 1). Since no authentic standards are available, conjugated metabolites were not quantified. In this case, peak areas were calculated as a measure of the relative abundance of each conjugate. However, while the same conjugate metabolite can be compared between different tissues, different conjugates cannot be compared because of their dissimilar response in ionization when using ion mass spectrometry.4
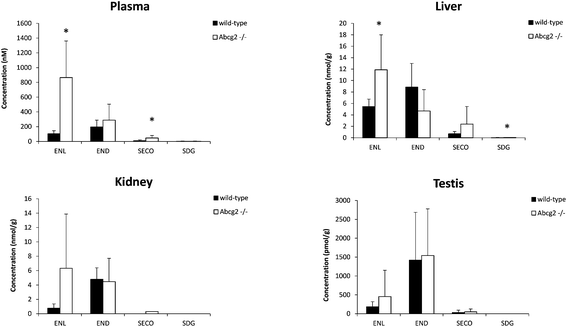 |
| Fig. 1 Concentration of lignans (SDG and SECO) and enterolignans (END and ENL) in the plasma, liver, kidney and testis of wild-type and Abcg2−/− male mice (n = 4–5) fed with LinumLife®Extra 1% for 7 days. Data are presented as means ± SD. *P < 0.05 versus wild-type. | |
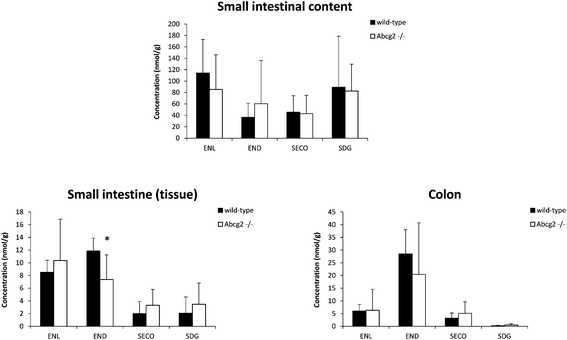 |
| Fig. 2 Concentration of lignans (SDG and SECO) and enterolignans (END and ENL) in the small intestinal content, small intestine, and colon of wild-type and Abcg2−/− male mice (n = 4–5) fed with LinumLife®Extra 1% for 7 days. Data are presented as means ± SD. *P < 0.05 versus wild-type. | |
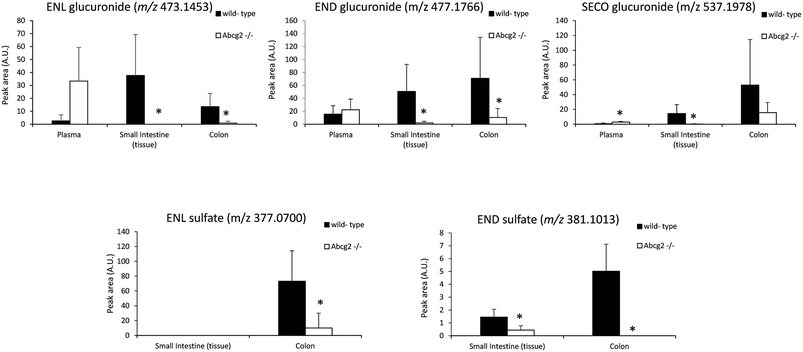 |
| Fig. 3 Determination of the peak areas (which is a measure of their relative abundance) of glucuronide and sulfate conjugated metabolites of enterolignans in the plasma, small intestine and colon of wild-type and Abcg2−/− male mice (n = 4–5) fed with LinumLife®Extra 1% for 7 days. Data are presented as means ± SD. *P < 0.05 versus wild-type. | |
Table 1 Enterolignan metabolites identified in the plasma and tissues from wild-type and Abcg2−/− male mice
Compound |
Retention time (min) |
m/z experimental |
Molecular formula |
Occurrence |
P, plasma; T, testis, L, liver; K, kidney, SIT, small intestine (tissue); SIC, small intestine (content); C, colon. Different retention times for the same compound (same molecular mass) indicate the presence of isomers. |
SECO glucuronidea |
8.30/9.05/10.08/12.36 |
537.1978 |
C26H34O12 |
P, T, L, K, SIT, C, SIC |
SECO sulfatea |
8.93/9.16 |
441.1225 |
C20H26O9S |
C, SIC |
END glucuronide |
10.37 |
477.1766 |
C24H30O10 |
P, T, L, K, SIT, C, SIC |
END sulfatea |
10.88/11.25 |
381.1013 |
C18H22O7S |
T, K, SIT, C, SIC |
END sulfo-glucuronide |
8.66 |
557.1334 |
C24H30O13S |
SIC |
ENL glucuronidea |
12.38 |
473.1453 |
C24H26O10 |
P, T, L, K, SIT, C, SIC |
ENL sulfatea |
13.09 |
377.07 |
C18H18O7S |
K, C, SIC |
ENL sulfo-glucuronide |
9.95 |
553.1021 |
C24H26O13S |
SIC |
Enterofuran (ENF)a |
16.31/19.18 |
283.1340 |
C18H26O10 |
P, T, L, K, SIT, C, SIC |
ENF glucuronidea |
13.77/12.31 |
459.1661 |
C24H28O9 |
P, T, L, K, SIT, C, SIC |
ENF sulfate |
14.45 |
363.0908 |
C18H20O6S |
C, SIC |
ENF sulfo-glucuronide |
9.64 |
539.1229 |
C24H28O12S |
K, SIC |
Effect of Abcg2 on the plasma concentrations of ENL, END, SECO, SDG and their conjugates
Plasma levels confirmed that ENL, END and SECO are Abcg2 in vivo substrates, since plasma concentrations were higher in Abcg2−/− than in wild-type mice, although significant differences (P < 0.05) were observed only for ENL and SECO (Fig. 1). The difference in the case of ENL is particularly important, with an 8-fold increase in the plasma concentration in Abcg2−/− compared with wild-type mice (866.2 ± 495.1 vs. 102.9 ± 43.1 nM, respectively). SDG concentrations were extremely low (2.2 ± 2.5 nM for Abcg2−/− mice and 2.1 ± 3.3 nM for wild-type mice).
With regard to conjugated metabolites, sulfate conjugates were not detected in plasma. However, differences between both groups of mice were observed in the case of glucuronide conjugates in plasma (Fig. 3), although a statistically significant difference (P < 0.05) was achieved only for SECO glucuronide. These differences probably partially reflect the dissimilarities in plasma concentrations reported for parent enterolignans (Fig. 1). The higher plasma levels in Abcg2−/− compared with wild-type male mice for ENL, SECO, SECO glucuronide, and to a lesser extent for END and ENL glucuronide, reveal that Abcg2 is an important determinant of their systemic distribution. A previous study using female mice fed with the same lignan-enriched diet13 showed significant higher plasma levels of END, END-glucuronide and SECO-glucuronide in Abcg2−/− as compared to wild-type female mice. Furthermore, the 5-fold higher plasma level of END was detected in female mice (both wild-type and Abcg2−/−) compared with the male mice used in the present study. Similarly, sex-dependent bioavailability of lignans has been previously reported in rats,17,18 with higher values for the female animals, which was in agreement with Kirkman et al., who reported human sex differences in the excretion of lignan metabolites as men excreted more ENL and less END than women.19 However, it should be noted that other reports in humans have found a similar pattern of excretion and conjugation for both sexes.20,21 In the same regard, no sex-related differences were observed in the human gut microbiota metabolism of lignans.22 Therefore, sex-dependent bioavailability and metabolism of lignans could occur in rodents but has not been observed in humans so far. Thus, independent of the sex, an important finding in our present study is that Abcg2 decreases the systemic exposure of enterolignans and their metabolites.
Effect of Abcg2 on tissue distribution of ENL, END, SECO, SDG and their conjugates
Liver and kidney analyses revealed higher levels of ENL in Abcg2−/− compared with wild-type animals, with statistically significant differences only for the liver (Fig. 1), which could be attributable to the higher plasma levels in Abcg2−/− mice (Fig. 1). SECO and SDG concentrations were very low in these organs. No differences were found between both groups of mice for glucuronide metabolites in the liver (ESI Table 1†). Sulfated metabolites were not detected in the liver, in accordance with the low sulfation pattern of previously reported lignans.21 Notably, ENL-sulfate concentration was 24-fold higher in the kidney of Abcg2−/− mice compared with wild-type animals (ESI Table 1†), which suggests an impaired elimination in urine of this compound and consequently its accumulation in the kidney from the animals without the transporter. This result suggests a probable role of Abcg2 in the tubular secretion of this compound. The involvement of Abcg2 in the transport and urinary excretion of sulfates has been previously reported23,24 and an important involvement of Abcg2 in the in vivo transport of phytoestrogen sulfates, including ENL-sulfate, has been previously suggested.14 Regarding testes, considerable END accumulation was detected in this organ (Fig. 1). No significant differences were found between both groups of mice for these compounds, except for SECO glucuronide (ESI Table 1†).
Relatively high accumulation of ENL, END, SECO, SDG and their conjugates was detected in the small intestinal content and colon (Fig. 2, 3 and ESI Table 2†), as expected after exposure to a flaxseed-enriched diet and the role of colon microbiota in the metabolism of these compounds.4,25,26 These results are also in agreement with the significant fecal excretion that, together with low systemic levels, suggests poor oral bioavailability of lignans.27,28 This impaired oral bioavailability has been suggested to be due to poor intestinal permeation characteristics or an extensive pre-systemic metabolism.28 In this regard, our present study adds Abcg2 as a new factor responsible for this phenomenon.
The levels of enterolignan glucuronides and sulfates in the small intestine and colon were considerably higher in wild-type as compared to Abcg2−/− animals (Fig. 3). Moreover, the levels of enterolignan glucuronides in the small intestinal content were also increased in wild-type animals (ESI Table 2†). These data indicate that intestinal excretion due to the enterohepatic circulation of these compounds is clearly dependent on the Abcg2 transporter. Therefore, wild-type animals showed probably a higher excretion of these compounds in the intestinal lumen, resulting in higher levels in wild-type animals compared with Abcg2−/− (Fig. 3).
Overall, these data indicate that the increased plasma levels of these compounds in Abcg2−/− as compared to wild-type animals could be due to an impaired intestinal and urinary excretion related to the lack of the transporter in knockout animals. Abcg2 affects plasma and tissue levels of these compounds, probably interfering with their enterohepatic circulation (intestinal and hepatic expression of the transporter) and elimination (renal expression). Nevertheless, the involvement of other factors such as different transporters, Phase-II enzyme polymorphisms or different gut microbiota compositions/functionalities cannot be ruled out. In fact a role for ENL excretion as a surrogate for a gut microbial profile associated with metabolic processes has been recently suggested.29 Compensatory changes in the expression of genes coding for proteins involved in metabolism and transport in Abcg2−/− mice that could affect tissue distribution of these compounds cannot be completely excluded. However, indications for such disturbing effects in previous studies have never been shown. In general, the lack of the Abcg2 transporter does not seem to change the expression of important genes of potential interest in this context.30–32
In our experiment, the dietary lignan content was 10 mg d−1 and this value ranged from 0.4 to 13.8 mg d−1 in some human populations.25 In the human colon lumen, a concentration of 10–1000 μM can be achieved.33 In this regard, our in vivo data may be biologically relevant and have therapeutic significance for enterolignans because any change in the activity of the Abcg2 transporter due to food–drug interactions, inhibitions or polymorphisms could affect plasma and tissue distribution of these compounds, thus decreasing for instance their presence in the intestine.
Although conjugation has been suggested as a very important mechanism by which the organism can control the level of active versus inactive metabolites,21 phytoestrogen conjugates may retain the biological activities of their parent molecules.34 Of special relevance in this context could be the change of ENL levels in the liver depending on the activity of Abcg2 (Fig. 1) since hepatoprotective effects have also been associated with lignans in a flaxseed supplemented diet.35 Moreover, the effect of the Abcg2 transporter in the kidney levels of some enterolignan metabolites (ESI Table 1†) constitutes an important outcome when taking into account the protective effect of dietary flaxseed on nephrotoxicity and oxidative damage in the kidney.36 Therefore, changes in the concentrations of enterolignans and their conjugated metabolites could affect their biological effects as antioxidants, antiangiogenic, antitumor and others in the different target organs, which could have important health consequences.
Our findings are especially important in the case of males because ENL is a relatively abundant constituent of prostatic fluid as well as human semen,37 and ENL and, to a lesser extent, END inhibit 5α-reductase that catalyzes the conversion of testosterone into 5α-dihydrotestosterone, the most active androgen; this could explain the health benefits of flaxseed in a rat model of benign prostatic hypertrophy.38 In addition, the cancer cell proliferation biomarker Ki67 decreased in prostate cancer patients after supplementation of a flaxseed-rich diet for 30 days.39 Enterolignans have also been reported to increase plasma androstanediol glucuronide in men through interactions with polymorphisms in the CYP19 gene.40 In our study, END was the most abundant enterolignan found in testes (Fig. 1).
Conclusions
Overall, our results support the fact that the ABCG2 transporter affects the tissue distribution of lignans and their microbial-derived enterolignans after a repeated flaxseed administration, which could have relevant consequences in the role of these compounds in the prevention of chronic diseases.
Conflicts of interest
Dr José Angel Marañon is employed by Tradichem, SL. The other authors declare no conflict of interest.
Acknowledgements
This work was supported by the Spanish Ministry of Economy and Competitiveness and the European Regional Development Fund (MINECO/FEDER, UE) [AGL2015-65626-R and AGL2015-64124-R], the Junta de Castilla y Leon (Consejería de Educación) [LE059U14] and the Spanish Ministry of Education, Culture and Sport [FPU14/05131 grant to DGM].
The Abcg2−/− mice were kindly supplied by Dr AH Schinkel from The Netherlands Cancer Institute. We are grateful to Prof. James McCue for assistance in language editing.
References
- J. W. Lampe, C. Atkinson and M. A. J. Hullar, J. AOAC Int., 2006, 89, 1174–1181 CAS.
- K. D. R. Setchell, N. M. Brown, L. Zimmer-Nechemias, B. Wolfe, P. Jha and J. E. Heubi, Food Funct., 2014, 5, 491–501 CAS.
- R. B. Teponno, S. Kusari and M. Spiteller, Nat. Prod. Rep., 2016, 33, 1044–1092 RSC.
- A. Quartieri, R. García-Villalba, A. Amaretti, S. Raimondi, A. Leonardi, M. Rossi and F. Tomàs-Barberàn, Mol. Nutr. Food Res., 2016, 60, 1590–1601 CAS.
- N. M. I. Taylor, I. Manolaridis, S. M. Jackson, J. Kowal, H. Stahlberg and K. P. Locher, Nature, 2017, 546, 504–509 CAS.
- S. Durmus, J. J. Hendrikx and A. H. Schinkel, Adv. Cancer Res., 2015, 125, 1–41 CrossRef PubMed.
- Q. Mao and J. D. Unadkat, AAPS J., 2015, 17, 65–82 CrossRef CAS PubMed.
- M. A. Versiani, T. Diyabalanage, R. Ratnayake, C. J. Henrich, S. E. Bates, J. B. McMahon and K. R. Gustafson, J. Nat. Prod., 2011, 74, 262–266 CrossRef CAS PubMed.
- A. González-Sarrías, V. Miguel, G. Merino, R. Lucas, J. C. Morales, F. Tomás-Barberán, A. I. Álvarez and J. C. Espín, J. Agric. Food Chem., 2013, 61, 4352–4359 CrossRef PubMed.
- O. M. Woodward, Am. J. Physiol.: Renal Physiol., 2015, 309, F485–F488 CrossRef CAS PubMed.
- C. McLean, A. Wilson and R. B. Kim, J. Clin. Pharmacol., 2016, 56, S40–S58 CrossRef CAS PubMed.
- V. Miguel, J. A. Otero, R. Garcia-Villalba, F. Tomas-Barberan, J. C. Espin, G. Merino and A. I. Alvarez, Drug Metab. Dispos., 2014, 42, 943–946 CrossRef PubMed.
- D. García-Mateos, R. García-Villalba, J. A. Marañón, J. C. Espín, G. Merino and A. I. Álvarez, J. Funct. Foods, 2017, 35, 648–654 CrossRef.
- K. van de Wetering and S. Sapthu, FASEB J., 2012, 26, 4014–4024 CrossRef CAS PubMed.
- S. Ge, Y. Wei, T. Yin, B. Xu, S. Gao and M. Hu, Mol. Pharm., 2017, 14, 2884–2898 CrossRef CAS PubMed.
- S. Bolca, M. Urpi-Sarda, P. Blondeel, N. Roche, L. Vanhaecke, S. Possemiers, N. Al-Maharik, N. Botting, D. De Keukeleire, M. Bracke, A. Heyerick, C. Manach and H. Depypere, Am. J. Clin. Nutr., 2010, 91, 976–984 CrossRef CAS PubMed.
- H. Xu, J. Gan, X. Liu, R. Wu, Y. Jin, M. Li and B. Yuan, J. Ethnopharmacol., 2013, 147, 224–231 CrossRef CAS PubMed.
- F. Hu, J. An, W. Li, Z. Zhang, W. Chen, C. Wang and Z. Wang, J. Ethnopharmacol., 2015, 169, 145–155 CrossRef CAS PubMed.
- L. M. Kirkman, J. W. Lampe, D. R. Campbell, M. C. Martini and J. L. Slavin, Nutr. Cancer, 1995, 24, 1–12 CrossRef CAS PubMed.
- C. L. Frankenfeld, Eur. J. Clin. Nutr., 2013, 67, 887–889 CrossRef CAS PubMed.
- N. P. Nørskov, C. Kyrø, A. Olsen, A. Tjønneland and K. E. Bach Knudsen, J. Proteome Res., 2016, 15, 1051–1058 CrossRef PubMed.
- P. Gaya, M. Medina, A. Sánchez-Jiménez and J. Landete, Molecules, 2016, 21, 1034 CrossRef PubMed.
- M. Suzuki, H. Suzuki, Y. Sugimoto and Y. Sugiyama, J. Biol. Chem., 2003, 278, 22644–22649 CrossRef CAS PubMed.
- N. Mizuno, T. Takahashi, H. Kusuhara, J. D. Schuetz, T. Niwa and Y. Sugiyama, Drug Metab. Dispos., 2007, 35, 2045–2052 CrossRef CAS PubMed.
- Q. Nie, M. Xing, J. Hu, X. Hu, S. Nie and M. Xie, Crit. Rev. Food Sci. Nutr., 2015, 57, 2432–2454 CrossRef PubMed.
- C. L. Frankenfeld, Mol. Nutr. Food Res., 2017, 61, 1500900 Search PubMed.
- T. Clavel, J. Doré and M. Blaut, Nutr. Res. Rev., 2006, 19, 187–196 CrossRef CAS PubMed.
- J. K. Mukker, D. Michel, A. D. Muir, E. S. Krol and J. Alcorn, J. Nat. Prod., 2014, 77, 29–34 CrossRef CAS PubMed.
- F. L. Miles, S. L. Navarro, Y. Schwarz, H. Gu, D. Djukovic, T. W. Randolph, A. Shojaie, M. Kratz, M. A. J. Hullar, P. D. Lampe, M. L. Neuhouser, D. Raftery and J. W. Lampe, Food Funct., 2017, 8, 3209–3218 CAS.
- B. Zhou, S. Zong, Y. Ney, P. A. Nair, G. Stewart and C. F. Sorrentino, Blood, 2005, 105, 2571–2576 CrossRef PubMed.
- J. S. Lagas, R. A. B. van Waterschoot, R. W. Sparidans, E. Wagenaar, J. H. Beijnen and A. H. Schinkel, Mol. Cancer Ther., 2010, 9, 319–326 CrossRef CAS PubMed.
- S. Dallas, L. Salphati, D. Gomez-Zepeda, T. Wanek, L. Chen, X. Chu, J. Kunta, M. Mezler, M.-C. Menet, S. Chasseigneaux, X. Decleves, O. Langer, E. Pierre, K. DiLoreto, C. Hoft, L. Laplanche, J. Pang, T. Pereira, C. Andonian, D. Simic, A. Rode, J. Yabut, X. Zhang and N. Scheer, Mol. Pharmacol., 2016, 89, 492–504 CrossRef CAS PubMed.
- E. Corsini, M. Dell'Agli, A. Facchi, E. De Fabiani, L. Lucchi, M. S. Boraso, M. Marinovich and C. L. Galli, J. Agric. Food Chem., 2010, 58, 6678–6684 CrossRef CAS PubMed.
- K. Beekmann, L. H. J. de Haan, L. Actis-Goretta, R. Houtman, P. J. van Bladeren and I. M. Rietjens, J. Steroid Biochem. Mol. Biol., 2015, 154, 245–253 CrossRef CAS PubMed.
- S. J. Hemmings and L. Barker, Cell Biochem. Funct., 2004, 22, 113–121 CrossRef CAS PubMed.
- S. Rizwan, A. Naqshbandi, Z. Farooqui, A. A. Khan and F. Khan, Food Chem. Toxicol., 2014, 68, 99–107 CrossRef CAS PubMed.
- B. Raffaelli, A. Hoikkala, E. Leppälä and K. Wähälä, J. Chromatogr., B: Anal. Technol. Biomed. Life Sci., 2002, 777, 29–43 CrossRef CAS.
- J.-F. Bisson, S. Hidalgo, R. Simons and M. Verbruggen, J. Med. Food, 2014, 17, 650–656 CrossRef CAS PubMed.
- W. Demark-Wahnefried, T. J. Polascik, S. L. George, B. R. Switzer, J. F. Madden, M. T. Ruffin, D. C. Snyder, K. Owzar, V. Hars, D. M. Albala, P. J. Walther, C. N. Robertson, J. W. Moul, B. K. Dunn, D. Brenner, L. Minasian, P. Stella and R. T. Vollmer, Cancer Epidemiol. Biomarkers Prev., 2008, 17, 3577–3587 CrossRef CAS PubMed.
- Y.-L. Low, J. I. Taylor, P. B. Grace, M. Dowsett, E. Folkerd, D. Doody, A. M. Dunning, S. Scollen, A. A. Mulligan, A. A. Welch, R. N. Luben, K.-T. Khaw, N. E. Day, N. J. Wareham and S. A. Bingham, J. Nutr., 2005, 135, 2680–2686 CAS.
Footnote |
† Electronic supplementary information (ESI) available. See DOI: 10.1039/c7fo01549f |
|
This journal is © The Royal Society of Chemistry 2018 |
Click here to see how this site uses Cookies. View our privacy policy here.