DOI:
10.1039/C7LC00993C
(Paper)
Lab Chip, 2018,
18, 190-196
Fluorescence-activated droplet sorting of lipolytic microorganisms using a compact optical system†
Received
14th September 2017
, Accepted 29th November 2017
First published on 29th November 2017
Abstract
Lipases are ubiquitous enzymes of great physiological significance that have been used extensively in multiple industries. Environmental microorganisms are a major source for the discovery of novel lipases with high catalytic efficiency and selectivity. However, current plate-based screening of lipase-producing strains is time consuming, labour intensive and inefficient. In this study, we developed an ultra-high throughput screening pipeline for lipase-producing strains based on fluorescence-activated droplet sorting (FADS) using a compact optical system that could be easily set up in an alignment-free manner. The pipeline includes droplet generation, droplet incubation, picoinjection of the fluorescence probe, and sorting of droplets with a throughput of 2 × 106 drops per h. We applied the pipeline to screen samples collected from different locations, including sediments from a hot spring in Tibet, soils from the Zoige wetland, contaminated soils from an abandoned oilfield, and a Chinese Daqu starter. In total, we obtained 47 lipase-producing bacterial strains belonging to seven genera, including Staphylococcus, Bacillus, Enterobacter, Serratia, Prolinoborus, Acinetobacter, and Leclercia. We believe that this FADS-based pipeline could be extended to screen various enzymes from the environment, and may find wide applications in breeding of industrial microorganisms.
Introduction
Microorganisms are a major source for the discovery and production of various enzymes with wide industrial applications.1 Screening processes are routinely used to obtain new enzymes from natural microorganisms and for selection of target strains from mutant libraries.2 Currently, microtiter plate-based screening is the most common screening format; however, the throughput is limited to 103 to 104 per round.3 Fluorescence-activated cell sorting (FACS), which can screen individual cells or water/oil/water emulsions4–8 with extremely high throughput (∼107 h−1), has become an appealing alternative. However, the widespread use of the FACS-based method is limited by its high cost and the multiple restrictions on complex double emulsion generation and modification.9,10 Recently, fluorescence-activated droplet sorting (FADS) using microfluidic technologies has quickly emerged as a powerful tool for single cell screening with ultra-high throughput.11 Important progress has been made in generating,12 mixing,13 dividing,14 and sorting15 of picoliter droplets. In the past few years, FADS has been applied to screening of many different enzymes including β-galactosidase,9 cellulase,16 α-amylase,17 and aldolase.18 Additionally, screening methods have been developed to encapsulate single cells in droplets, incubate droplets under various conditions, set up quantitative assays and reactions in droplets, and sort droplets with extraordinary efficiency. The flexibility of droplet manipulation on microfluidic devices opens the way to designing more complex and versatile screening assays for enzymes, as well as other biomedical applications.19–22
Lipases are ubiquitous enzymes that catalyze the hydrolysis of lipids23 and are broadly used in the food, pharmaceutical, and petroleum industries, as well as for biocatalysis.24,25 In the past few years, there has been great interest in the application of lipases to the production of biodiesel as an alternative renewable and eco-friendly fuel.23 Nevertheless, new lipases with distinct substrate specificities and improved stabilities still hold great importance in industrial applications.23 Recently, in vitro compartmentalization-based FACS for directed evolution of lipase in a model strain has been realized using a double-emulsion generated by membrane-extrusion.7 Gel microdroplets (GMD) have also been used to screen metagenomic libraries for lipolytic enzymes based on digital enzymatic activity assays.26 However, the FADS-based workflow for screening of lipases in droplets has not yet been reported. The feasibility of FADS for sorting environmental microorganisms, which grow much slower than model strains, based on their lipolytic activity remains obscure. Herein, we introduce a high throughput pipeline for screening of environmental lipase-producing bacteria based on the FADS technique. The pipeline allowed single-cell droplet generation, long-term droplet incubation, picoinjection13 of a fluorogenic substrate, and sorting of droplets with a throughput of up to 2 × 106 drops per h. We applied this pipeline to sorting and recovery of lipolytic microorganisms sampled from various unique environments of great research interest and application potential. Moreover, we developed a compact optical system for FADS that could easily be set up in a common laboratory without the need for complicated optical alignment.
Experimental
Bacteria and samples
The Pseudoalteromonas lipolytica S29 strain was discovered with high lipolytic activity in a sediment sample collected from the northwest Indian Ocean (50°51′E, 37°38′S) at a depth of 2500 m. The strain was isolated on marine agar 2216E (Difco, Becton Dickinson) containing 1% olive oil at 16 °C. In this study, we cultivated S29 using marine broth 2216E at 37 °C. The cells were then spun down and suspended in 2216E to 7 × 106 cells per mL to make droplets for evaluation of system performance.
Four samples from different locations were used, a soil sample from the Zoige wetland (33°25′N, 102°52′E, Sichuan, China) provided by Dr. Juanli Yun from the Institute of Microbiology, Chinese Academy of Sciences, a petroleum-polluted soil sample collected from an abandoned oil field in Dongying (38°15′N, 118°5′E, Shandong, China), a sediment sample from the Lalong hot spring in Maizhokunggar, Lhasa (29°24′N, 92°6′E, Tibet, China) provided by Professor Bian Wu from the Institute of Microbiology, Chinese Academy of Sciences, and a Daqu starter sample of Gujing Tribute Liquor from Bozhou (32°51′N, 115°53′E, Anhui Province, China). The samples were stored at −20 °C without cryopreservation. Ten grams of each sample were suspended in 100 ml of sterile 1× PBS (pH = 7.4) and shaken for 30 min (160 rpm, 30 °C). Next, 5 ml of extract was inoculated in a flask with 200 mL of Luria Bertani broth (LB) (peptone 10 g L−1, yeast extract 5 g L−1, NaCl 10 g L−1, pH = 7) supplemented with 1% (v/v) tributyrin. The samples were then cultivated for three days (200 rpm, 37 °C), after which they were diluted to a concentration of ∼7 × 107 cells per mL with LB medium to make droplets. The sample from the Zoige wetland was also tested with FADS without cultivation by direct dilution of the extract with LB medium.
Device fabrication
Microfluidic devices were used for droplet generation, picoinjection, and sorting (Fig. S2†). The devices were designed and fabricated based on previously described procedures,13 and fabricated in poly(dimethyl-siloxane) (PDMS) using rapid-prototyping soft lithography.27 The devices had channel heights between 14 and 25 μm and holes that were punched for channel inlets and outlets. The devices for picoinjection and sorting contained electrodes fabricated by filling empty channels in the shape of electrodes with low melting-temperature liquid solder (Indium Co., Clinton, NY, USA). All devices were used without silanization.
Lipase screening
Bacterial suspensions were prepared in culture media with the cell density adjusted to approximately 7 × 107 cells per mL. The flow-focusing device generated 3–4 pL droplets at a rate of 2700 per second. The number of cells per droplet followed a Poisson distribution28 when the bacterial cells were introduced at a theoretical concentration of 0.3 cells per drop, resulting in 74% of the droplets being empty and 22% containing a single cell. The device was operated with a total aqueous flow rate of 40 μL h−1 and 80 μL h−1 fluorinated oil with a surfactant (Cat. No.186-4006, BioRad, Hercules, CA, USA). The droplets were incubated in a 20–30 cm long piece of Teflon tubing (Cat. No. AWG30, 0.010 inch i.d., 0.028 inch o.d., Zeus Inc., Orangeburg, SC, USA) at 37 °C, with both ends of the tubing sealed with capillary wax (Hampton Research, Aliso Viejo, CA, USA). The incubation time was 12 h for S29 and 3 days for the environmental samples unless otherwise stated.
After incubation, the droplets were loaded into the picoinjection device to introduce fluorescein dibutyrate (FDB) as a fluorogenic substrate for measurement of lipase activity. A 10 mM FDB stock solution in dimethyl sulfoxide (DMSO) was diluted 1
:
20 with 13 mM Tris–HCl buffer (pH = 7.4), then injected into the droplets. The final FDB concentration in the droplets was about 250 μM. A voltage between 0 and 200 V at a frequency of 30 kHz was applied to the electrodes to trigger the injection at a throughput of 300 droplets per second. The droplets were collected in the receiving Teflon tubing (20–30 cm in length), then incubated at 25 °C for 1 h to allow lipolysis of FDB.
Next, the Teflon tubing with droplets was connected to the sorting device with a channel depth of 25 μm. The sorting process was operated with a flow rate of 10 μL h−1 droplets and 100 μL h−1 fluorinated oil without a surfactant (Cat. no.186-3004, BioRad, Hercules, CA, USA) to space the droplets. The fluorescence signal of each droplet was collected using a custom-made compact detection module mounted to the side port of an inverted microscope (IX81, Olympus, Japan). By defining the fluorescence threshold, droplets were sorted by dielectrophoresis.9 The FADS process was controlled using a program written in LabVIEW software (National Instruments, USA). Time series recordings were analysed using a program written in Matlab (MathWorks, Natick, MA, USA, the Matlab code is provided in the ESI†) to extract the fluorescence signal of each droplet. Before and after sorting, droplets were collected and imaged by fluorescence microscopy (Eclipse Ti, Nikon, Tokyo, Japan). Positive-sorted droplets were then collected in an Eppendorf tube with 100 μL LB medium. Next, 100 μL 1H,1H,2H,2H-perfluoro-1-octanol (PFO, Sigma Aldrich, USA) was added and vortexed to break the droplets and recover the bacterial cells in the medium. To validate the sorting efficiency, we also made a binary mixture of positive and negative ∼4 pL droplets. The positive droplets contained 25 μM FDB and 400 U mL−1 lipase (Aladdin, Shanghai, China), while the negative droplets contained 25 μM FDB without lipase. The mixed droplets were sorted directly after 1 hour incubation.
Lipase activity assays
The suspension containing recovered cells was plated on agar plates with LB medium, then incubated at 37 °C to allow growth of individual colonies. The colonies were selected and inoculated in 2 mL LB broth in culture tubes, then cultivated for 3 d (200 rpm, 37 °C). The lipase activities of the strains were evaluated with a plate assay as previously described.29 Briefly, holes of 8 mm were punched in agar plates containing LB broth supplemented with tributyrin as the substrate and Rhodamine B as the indicator. Next, 100 μL of cultivated bacteria in LB broth were added into the holes and the samples were then incubated for 2 days. Opaque halos developed around the holes filled with lipase-positive strains, and their sizes were measured for preliminary estimation of lipase activity. Next, selected strains were inoculated in 100 mL LB broth containing 1% tributyrin, then incubated for 60 h at 37 °C and 200 rpm. Following cultivation, the broth of each strain was centrifuged at 5000 rpm for 15 min. The supernatant was then filtered using a 0.2 μm pore size filter, after which it was subjected to ultrafiltration at 5500 rpm for 25 min at 4 °C in a Centricon 50 centrifuge filter with a 10 kDa cut-off membrane (Millipore, Bedford, MA, USA). The concentrated solutes were subsequently collected and adjusted to 1 mL, after which microgram quantities of proteins in the solutes were determined by the Bradford protein assay.30 Further analysis of extracellular lipase activity was conducted following the colorimetric method using p-nitrophenyl palmitate (p-NPP) as the substrate as previously described31 (experimental details can be found in the ESI†).
Results & discussion
Framework for FADS-based lipase screening
To establish a robust lipase screening pipeline, we divided our work into three phases. In the first phase, commercially available lipase was used to evaluate the properties of the fluorogenic substrate, including cytotoxicity, self-hydrolysis, and leakage in droplets. In the second phase, the sorting efficiency of FADS was optimized using commercially available lipase and a lipase-positive S29 strain. In the third phase, the pipeline was used in sorting and recovery of lipolytic microorganisms from different locations to assess the performance of the FADS system.
The optimized lipase screening pipeline was composed of four major steps as outlined in Fig. 1: i) microbial cell suspensions were prepared using samples collected from various sites, and droplets were then prepared using flow-focusing geometry (Fig. 1B); ii) the droplets were incubated for 0.5–3 d for secretion of lipase by the microbial cells; iii) the droplets were loaded into the picoinjection device to introduce FDB into each droplet as the fluorogenic substrate of lipase (Fig. 1C), after which the droplets were incubated for about 1 h to allow hydrolysis of FDB; iv) the droplets were sorted according to their fluorescence intensity using the sorting device2 (Fig. 1D). Finally, the positive-sorted droplets were demulsified, after which bacterial strains were retrieved and subjected to lipase activity assays.
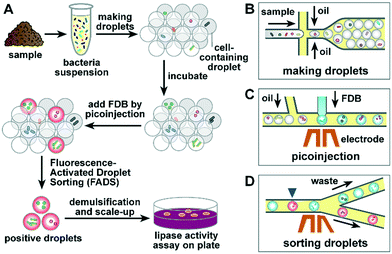 |
| Fig. 1 (A) The fluorescence-activated droplet sorting (FADS) pipeline for lipase discovery. (B–D) Schematics showing microfluidic devices for generation of single cell-encapsulated droplets, picoinjection of the substrate into the droplets, and FADS triggered by dielectrophoresis. | |
To simplify the procedure and eliminate the loss of droplets because of extra transfer steps, we used a piece of thin-wall Teflon tubing connected to the outlets of the PDMS devices to receive and store the droplets between different steps. At a length of 30 cm, the Teflon tubing can store more than 2.5 × 106 4 pL droplets, which requires 1 h of sorting time under the current settings. The droplets were then incubated in the Teflon tubing by sealing the two ends with capillary wax. The cells in droplets could receive sufficient oxygen supply because of the excellent gas permeability of Teflon.32 Following the removal of the sealing wax, the Teflon tubing was simply connected to the picoinjection device or sorting device for direct loading of droplets into the microfluidic channels.
Setup of the compact optical system for FADS
In contrast to the rapid progress regarding the new assays developed based on FADS, only limited work has been conducted to investigate the development of an integrated and portable detection and controlling system for FADS,33 which is critical to widespread adoption of FADS in common laboratories. Therefore, we designed an integrated optical system to help common users overcome the optical alignment challenges associated with fluorescence-activated droplet sorting34 (Fig. S1†). The system consisted of a compact optical module to measure the fluorescence of droplets, a high voltage module to deliver a high voltage–high frequency electric field to solder injected electrodes, a high-speed CCD camera to capture images and videos, and a power supply module to control the microscope and FADS system (Fig. S1A and B†).
The detection module (160 mm × 143 mm × 54 mm in size) contained a high-power diode laser, three photomultipliers (PMT) for detection of three fluorescence signals, and a 2 MHz analog-to-digital conversion circuit (Fig. S1C†). The wavelength of the laser and detectors can be flexibly customized to enable simultaneous detection of multiple fluorogenic probes (Table S1†). The V-mount of the detection module allowed easy connection with the side port of a standard inverted microscope. The optical path was aligned and die bonded in the detection module; thus, no further alignment was required. We also made a low-cost and compact high voltage module that was designed specifically for FADS. This optical system is alignment-free and features a compact size, light-weight portability, quick installation, and high robustness.
Measurements of lipase activity in droplets
Lipase can catalyse the hydrolysis of FDB to release fluorescein with green fluorescence (Fig. 2A). However, FADS-based lipase screening has not yet been reported, and the suitability of FDB for FADS-based sorting has not yet been evaluated. Moreover, when compared to previously used model strains such as Escherichia coli or Saccharomyces cerevisiae,7,26 a long incubation time for cell-encapsulated droplets is required to screen uncultivated bacteria, which grow much slower in laboratory media. To investigate the long-term cytotoxic effects of FDB, we measured the growth (OD600) of S29 with or without FDB (250 μM) using standard well-plate assays. We found that addition of FDB had no significant effect on the growth of S29 (Fig. 2B). However, the fluorescence data showed that the hydrolysis of FDB occurred spontaneously without S29 cells added (Fig. 2C). Therefore, FDB should not be added with bacterial cells during droplet generation as previously described,26 and the picoinjection step is essential to the quantitative measurement of the lipase activity of environmental bacteria in the droplets.
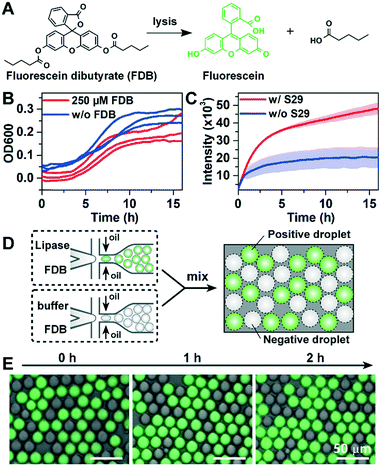 |
| Fig. 2 Lipase activity measurements using fluorescein dibutyrate (FDB). (A) Hydrolysis of FDB releases fluorescein, which renders the droplet flourescent; (B) plate-based OD600 measurements show that the addition of FDB didn't affect the growth of S29 cells. In the test group (red curves), FDB was added at a concentration of 250 μM; in the control group (blue curves), no FDB was added. (C) Plate-based fluorescence measurements indicate spontaneous hydrolysis of FDB (250 μM) without S29 cells added. In the test group (red curve), 100 μL of 500 μM FDB and 100 μL of S29 cells in 2216E medium (OD = 1.25) were added; in the control group (blue curve), 100 μL of 500 μM FDB and 100 μL sterile 2216E were added. (D) Evaluation of “leakage” of FDB using a binary mixture of droplets with or without lipase, the concentration of FDB is 250 μM; (E) time-lapse fluorescence imaging of the binary droplet mixture demonstrates that there is no leakage between neighboring droplets after 2 h incubation. | |
The leakage of reagents in water-in-oil droplets35,36 is a major compromise to the precision of droplet-based screening. To determine whether the measurement of droplet-based lipase activity is prone to leakage in BioRad droplet generation oil, two types of droplets were generated: “positive” droplets containing FDB and commercially-available lipase (40 U mL−1), and “negative” droplets containing FDB only. The dye leakage among droplets at different times was studied by mixing the positive and negative droplets (Fig. 2D). As shown in Fig. 2E, there was no detectable increase in fluorescence in the negative droplets during 2-h incubation, indicating that the leakage was insignificant (see the detailed method in the ESI†).
Sorting droplets based on lipase activity
To optimize the sorting condition, a binary mixture of positive and negative droplets (∼4 pL in volume) with a 20
:
19 mixing ratio was produced using either 400 U mL−1 lipase or Tris–HCl and 25 μM FDB. Sorting parameters including flow rates, frequency and magnitude of AC voltage pulses applied on electrodes and trigger delays were investigated and optimized (see Fig. S3 in the ESI† for a typical time series recording of the detected fluorescence signal). The positive and negative sorted droplets were collected separately, imaged and counted by fluorescence microscopy to calculate the sorting efficiency (Fig. S3†). A high enrichment factor was achieved with a speed of ∼2 × 106 drops per h, and the optimized sorting condition was adopted in the later sorting experiments for bacterial cells and real samples.
To further optimize the performance of the FADS-based lipase pipeline for bacterial samples, we encapsulated S29 cells in droplets of 2216E medium, and tested the effects of FDB concentration on sorting efficiency in the range of 50–250 μM. We did not test higher concentrations since precipitation occurred when the concentration of FDB exceeded 250 μM (Fig. S4A†). Before sorting, the droplets were incubated for 12 h to allow lipase production and secretion from the S29 cells. FDB was added to the droplets by picoinjection, and then incubated for 1 h before sorting. An optimal FDB concentration of 250 μM for bacterial cell sorting was obtained based on discrimination between droplets that did not contain S29 cells (negatives) and those that did (positives) (Fig. 3, Fig. S4†).
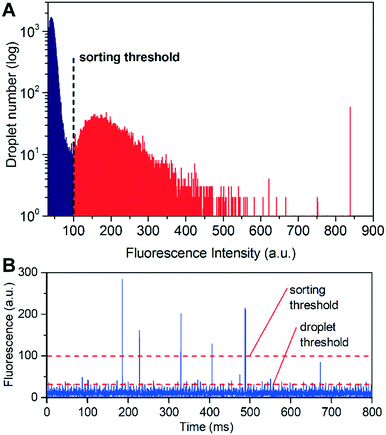 |
| Fig. 3 (A) Histogram showing the distribution of droplet fluorescence intensities for sorting of P. lipolytica S29 with 250 μM FDB (final concentration) as the substrate. Fluorescent droplets passing the sorting threshold (gray dashed line) were sorted and collected for cultivation. (B) A typical time series recording of the detected fluorescence signal of S29 sorting. A droplet detection threshold of 32 and a sorting threshold of 100 were used. | |
Lipase screening from various sampling sites
We applied the lipase screening method for the discovery of lipase-producing bacteria from various locations (see Fig. 4A for geographical locations), including the Zoige wetland, the Lalong hot spring in Tibet, an abandoned oil field in Dongying, and the Daqu starter of Gujin liquor from Anhui Province. These samples were selected either for their representation of untamed or extreme environments where novel types of lipase-producing microorganisms might exist, or for their great application potential in the liquor and petroleum industries. We extended the incubation time of environmental samples to 3 d, since their growth rates are usually slower and it may take a much longer time for them to adapt to laboratory cultivations. Using the optimized conditions, similar sorting histograms were obtained (Fig. S6D†), and those positive droplets with signals above the threshold were recovered and spread on LB agar plates to allow growth (Fig. S5 and S6†). Colonies were collected and transferred to liquid cultures, after which their lipolytic activities were evaluated via the tributyrin plate assays (Fig. S7†). The phylogenetic tree for positive lipolytic species based on 16S rRNA sequences and the number of strains isolated is shown in Fig. 4B. Among the 47 colonies identified with lipase activity, 11 bacterial species belonging to seven genera were identified, including Staphylococcus, Bacillus, Enterobacter, Serratia, Prolinoborus, Acinetobacter, and Leclercia (see Table S2† for the full list of sorted strains). These results suggest that our FADS-based lipase screening pipeline is robust and can be successfully applied to various samples from different environments.
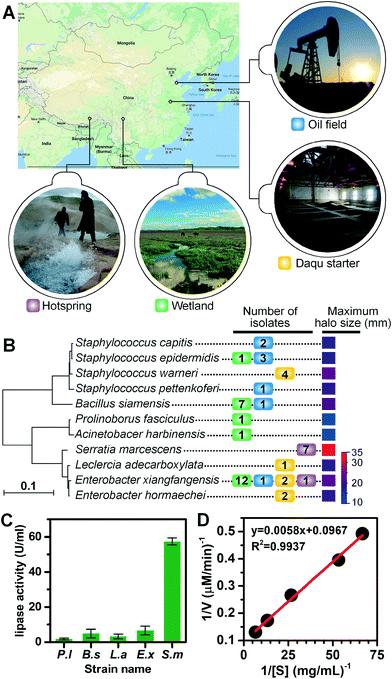 |
| Fig. 4 Lipase screening from environmental samples. (A) The geographical locations of the sampling sites (source: Google map) include: an oil field (blue, Dongying, Shandong), a Daqu starter (orange, Bozhou, Anhui), the Lalong hot spring (magenta, Lhasa, Tibet), and the Zoige wetland (green, Sichuan). (B) The phylogenetic tree shows the members of lipase-producing species we screened and the largest opaque halo sizes from related species. Numbers of isolates are colored corresponding to the color markers of sampling sites as shown in panel A. (C) The activity of extracellular crude lipases of P. lipolytica S29 (P.l), Bacillus siamensis ZF4 (B.s), Leclercia adecarboxylata J11 (L.a), Enterobacter xiangfangensis Z1 (E.x), and Serratia marcescens Y2 (S.m), measured using p-nitrophenyl palmitate (p-NPP) assays. (D) Lineweaver–Burk plot of extracellular crude lipase of S. marcescens Y2 obtained by p-NPP assays. | |
Four strains, Serratia marcescens strain Y2, Bacillus siamensis ZF4, Leclercia adecarboxylata J11, and Enterobacter xiangfangensis Z1, were selected for further study of lipase activity because they developed large halos on agar plates. The extracellular crude lipase activity of P. lipolytica S29 was also measured as the control. S29 cells were cultivated using 2216E culture medium instead of LB. The crude enzymes in culture supernatants were purified and concentrated 100 times by ultrafiltration to measure the lipolytic activity of crude enzymes in supernatants based on the p-NPP assay. One unit of enzyme activity (U) was defined as the amount of enzyme releasing 1 μmol p-nitrophenol (p-NP) from the substrate per min at 37 °C. As shown in Fig. 4C, Y2 exhibited the highest extracellular lipase activity (57.34 U mL−1) among the four strains. The Michaelis constant (Km) value and the specific activity of Y2 were calculated to be 158.9 μM and 2926.7 U mg−1 from the Lineweaver–Burk plot constructed using activity values depending on p-NPP concentrations. The linear plot indicated that the hydrolysis of p-NPP by the extracellular lipases of Y2 followed Michaelis–Menten kinetics (Fig. 4D). Further study is underway to clone, express and purify lipases from the obtained strains to enable more quantitative analysis of lipase activity.
Conclusions
In summary, we developed a FADS-based pipeline for high throughput screening of lipolytic microorganisms which consisted of droplet generation, incubation, introduction of a substrate using picoinjection, and sorting of droplets based on their fluorescence intensities. We investigated factors that might influence the sorting efficiency and concluded that picoinjection was indispensable for quantitative sorting of environmental samples because it prevented the interference induced by spontaneous hydrolysis of FDB after long-term incubation. We applied the pipeline to samples collected from various sites and successfully obtained 47 lipolytic bacterial strains, confirming the high effectiveness and robustness of the FADS method. Furthermore, we built a compact optical system that consisted of a laser light source, multi-channel fluorescence detectors, and FADS controlling circuits. The optical system can be easily coupled with standard microscopes for FADS without the need for optical alignments, which might significantly promote the wider adoption of FADS.
We believe that the FADS-based lipase screening pipeline can be widely applied to high throughput sorting of various environmental samples or mutant libraries. It will also be a useful tool for functional metagenomic screening based on in vitro gene expression systems.37 Future studies will be directed toward extending the workflow to other enzymes with industrial importance, and providing a highly efficient, low reagent consumption, and low-cost alternative to current screening technologies.
Conflicts of interest
There are no conflicts to declare.
Acknowledgements
We thank Mr. Guangji Sheng for help in construction of the optical system. We are grateful to Dr. Wanghui Xu and Dr. Shufang Zhao from Novozymes (Beijing) for fruitful discussions. We thank Professor Bian Wu from the Institute of Microbiology, Chinese Academy of Sciences for providing hot spring samples from Tibet, China. This study was supported by the Science and Technology Service Network Initiative of the Chinese Academy of Sciences (KFJ-SW-STS-165), the Key Program of Frontier Sciences of the Chinese Academy of Sciences (QYZDB-SSW-SMC008), the Strategic Priority Research Program of the Chinese Academy of Sciences (XDB15040102), and the National Key Research and Development Program of China (2016YFC0100904, 2016YFE0205800).
References
- J. L. Adrio and A. L. Demain, Biomolecules, 2014, 4, 117–139 CrossRef PubMed.
- R. Martinez and U. Schwaneberg, Biol. Res., 2013, 46, 395–405 CrossRef PubMed.
- H. Xiao, Z. Bao and H. Zhao, Ind. Eng. Chem. Res., 2015, 54, 4011–4020 CrossRef CAS PubMed.
- A. Aharoni, G. Amitai and K. Bernath,
et al.
, Chem. Biol., 2005, 12, 1281–1289 CrossRef CAS PubMed.
- K. Bernath, M. Hai and E. Mastrobattista,
et al.
, Anal. Biochem., 2004, 325, 151–157 CrossRef CAS PubMed.
- F. Ma, Y. Feng and G. Yang, Prog. Biochem. Biophys., 2012, 39, 299–306 CrossRef CAS.
- F. Ma, Y. Xie and C. Huang,
et al.
, PLoS One, 2014, 9, e89785 Search PubMed.
- E. Mastrobattista, V. Taly and E. Chanudet,
et al.
, Chem. Biol., 2005, 12, 1291–1300 CrossRef CAS PubMed.
- J. C. Baret, O. J. Miller and V. Taly,
et al.
, Lab Chip, 2009, 9, 1850–1858 RSC.
- A. Sciambi and A. R. Abate, Lab Chip, 2015, 15, 47–51 RSC.
- M. T. Guo, A. Rotem, J. A. Heyman and D. A. Weitz, Lab Chip, 2012, 12, 2146–2155 RSC.
- S. L. Anna, N. Bontoux and H. A. Stone, Appl. Phys. Lett., 2003, 82, 364–366 CrossRef CAS.
- A. R. Abate, T. Hung and P. Mary,
et al.
, Proc. Natl. Acad. Sci. U. S. A., 2010, 107, 19163–19166 CrossRef CAS PubMed.
- A. R. Abate and D. A. Weitz, Lab Chip, 2011, 11, 1911–1915 RSC.
- J. J. Agresti, E. Antipov and A. R. Abate,
et al.
, Proc. Natl. Acad. Sci. U. S. A., 2010, 107, 4004–4009 CrossRef CAS PubMed.
- M. Najah, R. Calbrix and I. P. Mahendra-Wijaya,
et al.
, Chem. Biol., 2014, 21, 1722–1732 CrossRef CAS PubMed.
- S. L. Sjostrom, Y. Bai and M. Huang,
et al.
, Lab Chip, 2014, 14, 806–813 RSC.
- R. Obexer, A. Godina and X. Garrabou,
et al.
, Nat. Chem., 2017, 9, 50–56 CAS.
- H. N. Joensson and S. H. Andersson, Angew. Chem., Int. Ed., 2012, 51, 12176–12192 CrossRef CAS PubMed.
- S. Mashaghi, A. Abbaspourrad, D. A. Weitz and A. M. van Oijen, TrAC, Trends Anal. Chem., 2016, 82, 118–125 CrossRef CAS.
- A. Reece, B. Xia and Z. Jiang,
et al.
, Curr. Opin. Biotechnol., 2016, 40, 90–96 CrossRef CAS PubMed.
- Z. Zhu and C. J. Yang, Acc. Chem. Res., 2017, 50, 22–31 CrossRef CAS PubMed.
- K. G. Daiha, R. Angeli, S. D. de Oliveira and R. V. Almeida, PLoS One, 2015, 10, e131624 Search PubMed.
- K. E. Jaeger and M. T. Reetz, Trends Biotechnol., 1998, 16, 396–403 CrossRef CAS PubMed.
- R. D. Schmid and R. Verger, Angew. Chem., Int. Ed., 1998, 37, 1609–1633 CrossRef CAS.
- M. Hosokawa, Y. Hoshino and Y. Nishikawa,
et al.
, Biosens. Bioelectron., 2015, 67, 379–385 CrossRef CAS PubMed.
- D. C. Duffy, J. C. McDonald, O. J. Schueller and G. M. Whitesides, Anal. Chem., 1998, 70, 4974–4984 CrossRef CAS PubMed.
- D. J. Collins, A. Neild and A. DeMello,
et al.
, Lab Chip, 2015, 15, 3439–3459 RSC.
- M. Samad, C. Razak and A. Salleh,
et al.
, J. Microbiol. Methods, 1989, 9, 51–56 CrossRef CAS.
- M. M. Bradford, Anal. Biochem., 1976, 72, 248–254 CrossRef CAS PubMed.
- U. K. Winkler and M. Stuckmann, J. Bacteriol., 1979, 138, 663–670 CAS.
- J. E. Kreutz, A. Shukhaev and W. Du,
et al.
, J. Am. Chem. Soc., 2010, 132, 3128–3132 CrossRef CAS PubMed.
- L. Mazutis, J. Gilbert and W. L. Ung,
et al.
, Nat. Protoc., 2013, 8, 870–891 CrossRef CAS PubMed.
- G. Sheng, J. Zhu, Y. Fan and A. Li, Chin. J. Biomed. Eng., 2016, 35, 370–374 Search PubMed.
- Y. Skhiri, P. Gruner and B. Semin,
et al.
, Soft Matter, 2012, 8, 10618–10627 RSC.
- Y. Chen, G. A. Wijaya and S. K. Tang, Lab Chip, 2012, 12, 5093–5103 RSC.
- G. Zubaite, K. Simutis and R. Galinis,
et al.
, Micromachines, 2017, 8, 62 CrossRef.
Footnotes |
† Electronic supplementary information (ESI) available: Additional figures and details on materials and methods. See DOI: 10.1039/c7lc00993c |
‡ Present address: Department of Biomedical Engineering, University of California, Davis 95616, USA. |
|
This journal is © The Royal Society of Chemistry 2018 |
Click here to see how this site uses Cookies. View our privacy policy here.