DOI:
10.1039/C8NH00026C
(Communication)
Nanoscale Horiz., 2018,
3, 538-544
Highly monodisperse beta-cyclodextrin-covellite nanoparticles for efficient photothermal and chemotherapy†
Received
6th February 2018
, Accepted 22nd May 2018
First published on 23rd May 2018
Abstract
High quality covellite phase copper sulfide nanoparticles with excellent photothermal performance were obtained using an easy, non-hot injection method. Then, colloidal stable drug delivery vehicles were fabricated with an amphiphilic polymer and covalently linked with beta-cyclodextrin, which allows the integration of multiple target ligands and drugs, thus providing a versatile nano-platform for efficient photothermal and chemotherapy.
Conceptual insights
Nanomaterials with strong near-infrared (NIR) absorption have attracted more and more attention in the fields of cancer therapy, molecular imaging and even photocatalysis. Copper sulfide (Cu2−xS, 0 < x ≪ 1) represents an efficient species among them. However, their potency was not completely exploited due to their numerous stoichiometries, which greatly influence their localized surface plasmon resonance. Here, by choosing suitable copper and sulfide precursors, and carefully optimizing the reaction conditions, we were able to obtain high quality covellite phase copper sulfide nanoparticles using a facile non-hot injection method. With the maximized copper-deficient stoichiometry, the obtained nanoparticles exhibit strong localized surface plasmon resonance around 965 nm with an extremely high mass extinction coefficient up to 60 L g−1 cm−1. Together with their outstanding photothermal conversion efficiency, they can serve as efficient photoacoustic and photothermal agents. Monodisperse drug delivery vehicles were then fabricated by surface modification with beta-cyclodextrin conjugated amphiphilic polymer. Adamantine-modified RGD peptide and doxorubicin can be easily anchored on it for targeted chemotherapy. The improved therapeutic effect with the combination of photothermal and chemotherapy was demonstrated later. This design allows the integration of many other target ligands and drugs, and thus provides a versatile nano-platform for theranostic applications.
|
1. Introduction
Since the successful utilization of gold nanoshells for the near-infrared (NIR, >760 nm) thermal therapy of tumors,1 nanomaterials with NIR absorption such as gold nanostructures, graphene, MoS2, etc.,2–5 have attracted more and more interest from scientists in the fields of cancer therapy,6 molecular imaging,7 and even photocatalysis.8 These materials can transduce tissue penetrable NIR light into heat, and therefore have been widely explored as photothermal agents recently. Under the irradiation of a nanosecond pulsed laser, these materials can release ultrasound waves, which was applied as a brand new imaging technique named photoacoustic (PA) imaging to provide deeper depth profile (than optical based techniques) and greater spatial resolution (than ultrasound imaging).9,10 Very recently, these nanomaterials were introduced into photocatalysis as efficient light absorbers, since NIR light makes up most of the solar energy (54.3%).11
The extinction coefficient is one of the most important parameter for these NIR absorbers, which represents their ability to harvest light and can significantly influence their performance in photothermal therapy, photoacoustic imaging and other fields.12,13 The initial investigation of NIR absorbers for photothermal related purposes mainly focused on anisotropic gold nanostructures due to their extraordinarily high molar extinction coefficient which results from their surface plasmon absorption.14 However, in the last few years people started to realize that the mass extinction coefficient was actually more important for applications, which influences the dose required during light irradiation.12,15,16 Thus, gold nanostructures were not favored because of their large size and mass, which diminished their mass extinction coefficient.
Consequently, many other candidates were developed for photothermal related applications, including reduced graphene oxide, dye tagged proteins and many metal chalcogenide nanomaterials (such as MoS2, MnO2, WS2, and CoSe).12,17 Among them, copper sulfide (Cu2−xS, 0 < x ≪ 1) nanoparticles represent a class of efficient inorganic NIR absorbers, which were heavily explored as photothermal and photoacoustic agents recently.18,19 Our early work has revealed that the absorption of Cu2−xS in the near-infrared range was due to the localized surface plasmon resonances arising from the valence band free carriers in the nanocrystals,20 which guarantees their high extinction coefficient. Meanwhile, the plasmon resonance band of Cu2−xS was located in the NIR range resulting from their suitable free carrier density.21 Thus, no shape control was required to achieve NIR absorption for Cu2−xS nanoparticles. In addition, free carrier density in these semiconductor plasmonics was generated from copper-deficient copper(I) sulfides,20,21 and therefore all copper deficient Cu2−xS phases possess NIR absorption except non-oxidized Cu2S nanoparticles. As a result, it is very easy to prepare copper sulfide nanoparticles with strong NIR absorption, and therefore numerous studies on copper sulfide based photothermal therapy and photoacoustic imaging have been reported.7,18 However, on the other hand, due to the ease of obtaining NIR absorption for copper sulfide nanoparticles, less attention was paid to the optimization of their synthetic parameters and crystal structure which of course can significantly influence their optical property. Indeed, re-examining the data in the literature, we found that the mass extinction coefficients can be quite different from each other for copper sulfide nanoparticles, even when they were prepared using a similar approach.22,23 Based on the above mentioned theory, more copper-deficiency (increasing x) leads to an increased extinction coefficient. In other words, covellite (CuS, x = 1) represents the best NIR light absorber in the whole series of the copper sulfide family.
Previously, covellite nanoparticles for biomedical applications were always prepared in the aqueous phase, which leads to an irregular shape with low crystallinity and stability.24,25 Although high quality Cu9S5, Cu7S4 and Cu1.8S nanoparticles were prepared for photothermal related applications,26,27 the synthesis of high quality covellite (CuS) nanoparticles for photothermal therapy and photoacoustic imaging was still lacking.
Here, we present an easy, non-hot injection method for the preparation of high quality covellite (CuS) nanoparticles. After optimization, CuS nanoparticles of around 18 nm were obtained with an extremely high mass extinction coefficient up to 60 L g−1 cm−1 at 965 nm (Table S1, ESI†), which was maximal among all inorganic nanomaterials to the best of our knowledge. In order to introduce these CuS nanocrystals for biomedical applications, we further transferred these nanocrystals into aqueous solution with an amphiphilic polymer and covalently linked them with beta-cyclodextrin (Scheme 1). Taking advantage of the host–guest interaction, targeting ligands such as adamantine-modified RGD peptide can be further anchored on the nanoparticles for the recognition of integrin-positive cancer cells. Together with the high extinction coefficient and outstand photothermal conversion efficiency (determined to be higher than 40%), these CuS nanocrystals can serve as efficient photothermal and photoacoustic imaging agents. In addition, the anticancer drug doxorubicin can also be loaded onto the nanoparticles via hydrophobic and electrostatic interaction. This design allows the integration of many other target ligands and drugs, and thus can provide a versatile nano-platform for highly efficient photothermal and chemotherapy. As an excellent NIR absorber, these semiconductor covellite nanoparticles also hold promise for many other NIR light related purposes in biology and engineering.
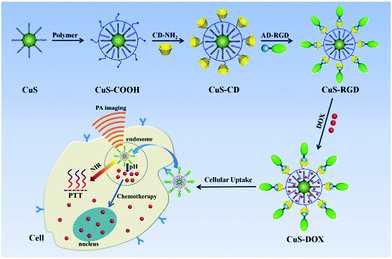 |
| Scheme 1 Functionalization of covellite nanoparticles for the photothermal and chemotherapy of cancer cells. | |
2. Results and discussion
2.1 Synthesis of covellite nanoparticles
Covellite (CuS) nanoparticles were prepared with Copper acetylacetonate (Cu(acac)2) as a copper source and sulfur powder as a sulfide source with a non-hot injection thermolysis method. Cu(acac)2 was dissolved in a mixture of oleylamine and 1-octadecene, and sulfur powder was dissolved in oleic acid. After these two solutions were mixed, a deep brown solution was obtained. Under the optimized Cu
:
S ratio, reaction temperature and reaction time, we were able to obtain high quality covellite nanoparticles with a diameter of ∼18 nm (Fig. 1 and Fig. S1, ESI†) and an intense absorption around 965 nm (Fig. 2A). It is important to mention that the ratio of Cu(acac)2 and sulfur powder plays an important role to obtain high quality covellite nanoparticles. At relatively high Cu
:
S ratio (1
:
0.5), the obtained copper sulfide nanoparticles were not covellite phase, and the absorption peak was at longer wavelength with a decreased extinction. This phenomenon is in accordance with the previous theory that decreased free carrier concentration leads to a decreased extinction coefficient and longer resonance wavelength (Fig. 2A).20 When the Cu
:
S ratio was smaller than 1, covellite nanoparticles were formed according to the XRD patterns (Fig. 3B, JCPDS: 003-1090). This result is in accordance with the Energy dispersive X-ray spectroscopy data (Fig. S2, ESI†). Accordingly, the absorbance of the obtained copper sulfide nanoparticles at NIR range increased with the decrease of Cu
:
S ratio until the Cu
:
S ratio reached 1
:
2. The reaction temperature and reaction time were also optimized based on their NIR absorption intensity. At low reaction temperature, irregular shaped nanoparticles were formed (Fig. S3, ESI†) with relatively low NIR absorption (Fig. S4, ESI†). On the other hand, the NIR absorption also decreased when the temperature is higher than 140 °C, which may be related to the formation of another stoichiometry at high temperature.28 The reaction time was found to have limited influence on the absorption intensity of the nanoparticles (Fig. S5, ESI†). The plasmonic absorption band was observed immediately after the temperature reached 120 °C, and the difference was negligible between 0.5 h and 2 h. Other organo-sulfur precursors prepared by dissolving sulfur powder in 1-octadecene and oleylamine were attempted in the synthesis as well, since the sulfur status can affect the reaction process.29,30 However, only non-uniform nanoparticles with relatively long plasmon resonance wavelength were obtained (Fig. S6, ESI†). Here, it is important to mention that obtaining the desired crystal phase for copper sulfide was difficult due to its numerous copper-deficient stoichiometries (there were 86 existing Cu2−xS structures). All kinds of factors such as reaction temperature, solvent, capping ligand, choice of the precursors and their ratios all affect the crystal phase and the properties of the final products. Therefore, our established method without complicated copper and sulfide precursors, provides a simple but effective way to obtain high quality covellite nanoparticles.
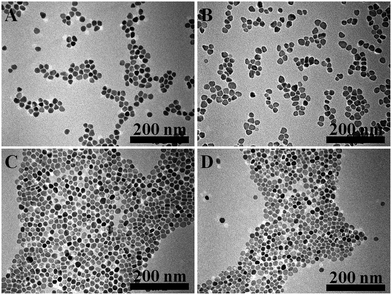 |
| Fig. 1 TEM images of copper sulfide nanoparticles synthesized with different Cu : S ratios, 1 : 0.5 (A); 1 : 1 (B);1 : 2 (C); 1 : 4 (D). | |
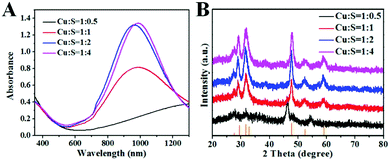 |
| Fig. 2 Absorption spectra (A) and XRD patterns (B) of copper sulfide nanoparticles synthesized with different Cu : S ratios. | |
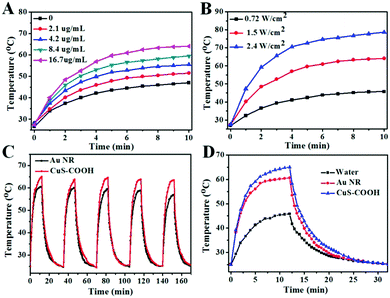 |
| Fig. 3 Temperature increase of covellite nanoparticle solutions after being irradiated with a 980 nm NIR laser with different concentrations (A) and laser power densities (B). (C) Temperature change of CuS–COOH and Au NR over 5 LASER (980 nm, 1.5 W cm−2) ON/OFF cycles. The absorbance of both materials was adjusted to 1.0 at 980 nm (∼16.7 μg mL−1) before irradiation. (D) The monitored temperature profiles of different materials irradiated by a laser for 12 min, followed by naturally cooling with the laser light turned off. A 980 nm laser with a power density of 1.5 W cm−2 was used. | |
The mass extinction coefficients of the as-prepared copper sulfide nanoparticles with different Cu
:
S ratios were calculated thereafter (Table S2, ESI†), and found to be as high as 60 L g−1 cm−1 at 965 nm for CuS nanoparticles prepared with a Cu
:
S ratio of 1
:
2 and 1
:
4. This value was almost double compared with the traditional gold nanostructures, other metal sulfides and 2D nanomaterials (Table S1, ESI†). Assuming that the density of the nanoparticles is the same as the bulk (4.6 g cm−3),23 the molar extinction coefficient of the obtained CuS nanoparticles was calculated to be 3.8 × 108 M−1 cm−1 at 965 nm, which is comparable with small gold nanorods.31 It is interesting to mention that the mass extinction coefficient of the CuS nanoparticles (Cu
:
S = 1
:
2) at 808 nm was 32.4 L g−1 cm−1, which was also higher than the above mentioned materials. Re-examining the data in the literature, we found that the mass extinction coefficient of CuS had already reached 45 L g−1 cm−1 (recalculated based on the data in the literature) in early work,23 however people only focused on molar extinction coefficient at that time. Therefore, it is not surprising to obtain higher extinction after careful optimization here. As a key parameter for NIR absorbers, we believe this material has great potential in photothermal therapy and photoacoustic imaging.
2.2 Photothermal performance of covellite nanoparticles
To examine their performance for biomedical applications, we first transferred these hydrophobic nanoparticles into an aqueous phase with a poly(maleic anhydride) based amphiphilic polymer.32 The negligible change in the absorption intensity and peak position before and after phase transfer indicates the limited effect of the solvents on the light extinction of CuS nanoparticles (Fig. S7, ESI†). It is important to mention that these highly negative charged (Table S3, ESI†) polymer encapsulated CuS nanoparticles with abundant carboxyl groups (CuS–COOH), maintain excellent colloidal stability in aqueous solution. The transparent green colored solution can be stored in the refrigerator for at least 3 weeks without any precipitate, and the absorbance was only slightly decreased during the storage (Fig. S8, ESI†).
With a broad absorption peak around 965 nm, the photothermal effect can be simply conducted with a commonly used 980 nm laser. As shown in Fig. 3A, the temperature of the CuS–COOH solution increased significantly under laser irradiation. Benefitting from their high mass extinction coefficient, a relatively low concentration of nanoparticles was required here to obtain the same temperature increase compared with previous reports.25,33 Meanwhile, higher temperature can be achieved with increased power density (Fig. 3B). Fast response and large temperature separation between the nanoparticles and the control (water, Fig. S9B, ESI†) can also be achieved with high power density, which is crucial for photothermal therapy. Here we choose a laser power density of 1.5 W cm−2 in the following experiments in consideration of the potential toxicity of the laser (Fig. S9A, ESI†). With a broad surface plasmon peak, the photothermal effect can be efficiently triggered under 808 nm irradiation (Fig. S10, ESI†). In addition, a 1064 nm laser may also be used to perform photothermal therapy in the second near-infrared region.
Photothermal conversion efficiency is another important parameter for photothermal therapy, which represents the ability to convert the harvested light into heat. Here the as-prepared covellite nanoparticles were first compared with gold nanorods (Au NR, Fig. S11, ESI†), which are commonly used for photothermal treatment.34 Both of the materials exhibited high photothermal stability during 5 laser ON/OFF cycles (Fig. 3C). However, the Au NRs exhibited a lower temperature increase with a photothermal conversion efficiency of 22.6% calculated according to the well-establish method,35 which is in accordance with the previous report,36,37 although some early work with Au NRs under different measurement conditions showed that it can be up to 95%.38,39 The photothermal conversion efficiency of the CuS nanoparticles was calculated to be 41.9%, which was much higher than that of the Au NRs. Although not the best, this value was outstanding among most inorganic nanomaterials.40,41 Besides, the covellite nanoparticles exhibited a smaller size and broader absorption peak (Fig. S11, ESI†) which are superior for photothermal therapy.42 Basically, the photothermal performance of the material can be determined by the product of the mass extinction coefficient (ability of light harvest) and photothermal conversion efficiency (ability of energy conversion). Thus, the obtained covellite nanoparticles with both superior mass extinction coefficient (60 L g−1 cm−1) and photothermal conversion efficiency (41.9%) can be excellent photothermal agents.
To investigate the photothermal effect of the obtained CuS–COOH nanoparticles toward cancer cells, HeLa cells incubated with and without nanoparticles were exposed to a 980 nm laser for 4 min. The media temperature without nanoparticles should be below 40 °C (Fig. 3D), which is safe for cells in a short period of time,43 therefore no photo damage was observed (Fig. 4). While for the one incubated with CuS–COOH nanoparticles, dramatic cellular death can be observed after laser irradiation due to the intense photothermal effect. Thus the combination of CuS nanoparticles with the confined beam of the laser can provide a remote-controlled and site-specific photothermal treatment.
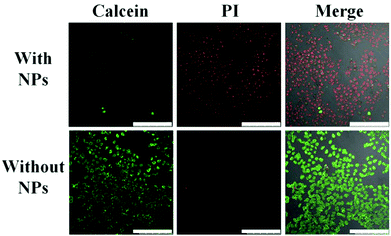 |
| Fig. 4 Cell viability of HeLa cells incubated with CuS–COOH under NIR laser treatment (980 nm, 1.5 W cm−2). Viable cells were stained with calcein (green) and dead cells were stained with PI (red). The scale bar is 250 μm. | |
2.3 Photoacoustic imaging with covellite nanoparticles
Under a pulsed laser, the periodic temperature increase of the material can induce an initial pressure increase due to thermoelastic expansion. The pressure wave can be detected with an ultrasonic transducer to produce a photoacoustic signal.6 Therefore, photothermal performance is absolutely a key factor for PA imaging, and the obtained covellite nanoparticles should be an excellent candidate as PA contrast agents. Here, gold nanorods, CuS nanoparticles and pure water were filled in a polyethylene tube, and then the photoacoustic signals were acquired under a 1064 nm pulsed laser (Fig. 5).44 We found that water itself did not generate PA signals, and the PA signal of the CuS nanoparticles was about 60% higher than that of the Au NRs under the same conditions. This phenomenon strongly emphasizes the potential of the obtained covellite nanoparticles in photoacoustic imaging.
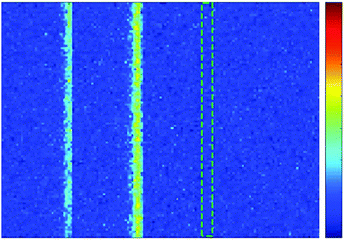 |
| Fig. 5 Photoacoustic imaging of AuNR (left), CuS–COOH (middle) and water (right) packed in a polyethylene tube. | |
2.4 Photothermal and chemotherapy with covellite nanoparticles
Taking advantage of the abundant carboxyl groups on the nanoparticles, CuS–COOH nanoparticles were first covalently linked with amino group modified beta-cyclodextrin through amide reaction (CuS–CD). With high water solubility, good biocompatibility and inclusion ability, the modification of beta-cyclodextrin enables further supramolecular decoration of the nanoparticles and was widely applied in drug delivery systems.45 Here, commercially available adamantine-modified cyclo(Arg-Gly-Asp-D-Phe-Lys) peptide was anchored on the CuS nanoparticles (CuS–RGD) for the recognition of integrin-positive cancer cells, via the strong host–guest interaction between cyclodextrin and adamantine.46 Other adamantine modified targeting ligands can also be used as required for the recognition of specific cell lines. With a hydrophobic layer and highly negative charged surface resulting from the carboxyl groups, various drugs (such as methylene blue, ICG, chlorin e6 etc.) can be further loaded on the nanoparticles through hydrophobic interaction or electrostatic attractions for therapeutic purposes.47 Here, a commonly used anticancer drug, doxorubicin hydrochloride (DOX), was employed to examine the potential of the as-prepared nanoparticles as drug delivery vehicles. Dox can be loaded on nanoparticles via both hydrophobic interaction47 and electrostatic attraction.48 Around 250 mg of DOX was incorporated per gram of the CuS nanoparticles (CuS–DOX), which was sufficient for cancer therapy (around 2700 DOX molecules per nanoparticle). Note that this was not the maximal value for drug loading; this concentration was adopted because the nanoparticles can still retain a negatively charged surface (Table S3, ESI†) and colloidal stability in aqueous solution which are crucial for drug delivery. Too high a DOX payload will lead to the aggregation of the nanoparticles. From the photographs in Fig. 6A, we can find that the color of the DOX loaded CuS nanoparticles turned from dark green into grey, but still maintained excellent colloid stability in aqueous solution. Both characteristic absorption of DOX and CuS were also observed after drug loading (Fig. 6A). The red-shift of the absorption peak of DOX may be related to their intermolecular interaction on the nanoparticles. The relatively weak absorption intensity of DOX was due to the high extinction of CuS nanoparticles. The surface modification process was monitored with TEM images (Fig. S12, ESI†), FTIR spectra (Fig. S13, ESI†), dynamic light scattering (Fig. S14, ESI†) and zeta potential measurements (Table S3, ESI†). CuS nanoparticles maintain excellent colloidal stability and monodispersity during the modification process. In addition, the obtained CuS nanoparticles exhibited high stability in physiological solutions (Fig. S15, ESI†). It is interesting to see that the fluorescence of DOX was almost completely quenched after loading due to the high extinction of CuS nanoparticles even at 550 nm (Fig. 6B). Thus, one can monitor the release of DOX by measuring the emission intensity of a diluted suspension of CuS–DOX. Negligible fluorescence increase can be observed under pH 7.4 within one day, indicating the stability of the nanocomposites under neutral pH (Fig. 6C). While a dramatic increase of emission intensity appears under pH 5, indicating a fast drug release. This phenomenon may be related to the improved water solubility of DOX and decreased surface charge of the nanoparticles due to the protonation of carboxyl groups at low pH, thus result in a weakened interaction between the nanoparticles and DOX. The pH dependent release of DOX can be beneficial for drug delivery due to the low pH in a tumor microenvironment. Since the nanoparticles enter the cells mainly through endocytosis and are accumulated within acidic endosomes and lysosomes,42 the DOX release can also be triggered when the nanocomposites get into the cells.
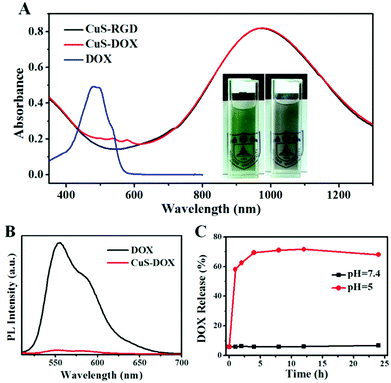 |
| Fig. 6 (A) Absorption spectra of CuS–RGD nanoparticles before and after DOX loading. The inset shows the corresponding photographs. (B) Fluorescence spectra of DOX loaded CuS nanoparticles and free DOX at an equivalent DOX concentration. (C) Drug release from CuS–DOX nanoparticles under different pHs. | |
The cytotoxicity of all these nanoparticles was evaluated using a standard MTT method (Fig. 7B). Polymer coated CuS nanoparticles and CD modified CuS nanoparticles did not show obvious cytotoxicity. However, RGD modified CuS nanoparticles exhibited a slightly higher cytotoxicity which may be related to the increased cell uptake of the nanoparticles due to the target effect. For the DOX loaded nanoparticles, dramatic cytotoxicity toward HeLa cells was observed when the drug concentration reached 2.5 μg mL−1 (10 μg mL−1 for CuS), demonstrating the chemotherapy effect of the nanocomposites.
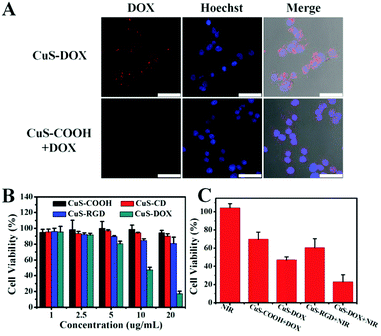 |
| Fig. 7 (A) CLSM images of HeLa cells incubated with DOX loaded CuS–RGD and DOX loaded CuS–COOH nanoparticles with a DOX concentration of 5 μg mL−1 for 2 h. The scale bar is 75 μm. (B) Cell viabilities after incubation with different concentrations of CuS–COOH, CuS–CD, CuS–RGD and CuS–DOX nanoparticles. (C) Cell viabilities of drug loaded CuS nanoparticles with and without NIR irradiation (980 nm, 1.5 W cm−2) at an equivalent CuS concentration of 10 μg mL−1. The standard deviations in (B and C) were obtained from at least three independent experiments carried out in triplicate. | |
To investigate the drug release behavior and the targeting ability of the nanoparticles toward integrin-positive HeLa cells, drug loaded CuS–COOH and CuS–RGD were incubated with cancer cells respectively. The emission of DOX mainly comes from the released portion since their emission was quenched on CuS nanoparticles. Obvious strong emission of DOX can be observed when cancer cells were incubated with RGD modified CuS nanoparticles (Fig. 7A), which indicates the improved therapeutic effect of the target ligand modified nanoparticles. In accordance with the CLSM images, MTT data also demonstrated that DOX loaded nanoparticles with RGD modification provided higher cytotoxicity (Fig. 7C and Fig. 16, ESI†). The photothermal therapy of CuS nanoparticles alone was evaluated then with RGD modified CuS nanoparticles under a 980 nm laser, which also can induce an obvious decreased cell viability. Since higher temperature can help release the drug (Fig. S17, ESI†), the photothermal effect of CuS should also be beneficial for chemotherapy. When exposing the CuS–DOX treated cells to NIR laser, much higher cytotoxicity can be observed than any single therapeutic method alone. The dramatically increased cytotoxicity demonstrates the high therapeutic effect from the combination of photothermal therapy and chemotherapy.
3. Conclusions
In summary, by choosing suitable copper and sulfide precursors, and carefully optimizing the reaction conditions, we were able to obtain high quality covellite phase (Cu2−xS, x = 1) copper sulfide nanoparticles. Due to the maximized copper-deficient stoichiometry, strong localized surface plasmon resonance absorption around 965 nm was achieved with an extremely high mass extinction coefficient up to 60 L g−1 cm−1. Together with their outstanding photothermal conversion efficiency (41.9%), these CuS nanocrystals can serve as efficient photothermal and photoacoustic imaging agents. As a proof of concept, we transferred these nanocrystals into aqueous solution with an amphiphilic polymer and covalently linked them with beta-cyclodextrin. Targeting ligands such as adamantine-modified RGD peptide can be further anchored on the nanoparticles via an easy host–guest interaction. In addition, anticancer drug doxorubicin can also be loaded onto the nanoparticles for chemotherapy. This design allows the integration of many other target ligands and drugs, and thus can provide a versatile nano-platform for the combination of photothermal and chemotherapy. As an excellent NIR absorber, these semiconductor plasmonic nanoparticles also hold promise for many other applications in biology and engineering.
Conflicts of interest
There are no conflicts to declare.
Acknowledgements
This work was sponsored by the National Natural Science Foundation of China (21335004, 21673108, and 21605088), the Natural Science Foundation of Jiangsu Province (BK20160884), and the Natural Science Foundation of the Jiangsu Higher Education Institutions (16KJB150031). We also acknowledge the support from the International Cooperation Foundation from the Ministry of Science and Technology (2016YFE0130100).
Notes and references
- L. R. Hirsch, R. J. Stafford, J. A. Bankson, S. R. Sershen, B. Rivera, R. E. Price, J. D. Hazle, N. J. Halas and J. L. West, Proc. Natl. Acad. Sci. U. S. A., 2003, 100, 13549–13554 CrossRef PubMed.
- W. Y. Yin, J. Yu, F. T. Lv, L. Yan, L. R. Zheng, Z. J. Gu and Y. L. Zhao, ACS Nano, 2016, 10, 11000–11011 CrossRef PubMed.
- X. Z. Zhu, H. K. Yip, X. L. Zhuo, R. B. Jiang, J. L. Chen, X. M. Zhu, Z. Yang and J. F. Wang, J. Am. Chem. Soc., 2017, 139, 13837–13846 CrossRef PubMed.
- X. X. Yao, X. X. Niu, K. X. Ma, P. Huang, J. Grothe, S. Kaskel and Y. F. Zhu, Small, 2017, 13, 11 Search PubMed.
- L. S. Lin, X. Y. Yang, Z. J. Zhou, Z. Yang, O. Jacobson, Y. J. Liu, A. Yang, G. Niu, J. B. Song, H. H. Yang and X. Y. Chen, Adv. Mater., 2017, 29, 1606681 CrossRef PubMed.
- W. S. Chen, J. Ouyang, H. Liu, M. Chen, K. Zeng, J. P. Sheng, Z. J. Liu, Y. J. Han, L. Q. Wang, J. Li, L. Deng, Y. N. Liu and S. J. Guo, Adv. Mater., 2017, 29, 7 Search PubMed.
- J. Weber, P. C. Beard and S. E. Bohndiek, Nat. Methods, 2016, 13, 639–650 CrossRef PubMed.
- J. B. Cui, Y. J. Li, L. Liu, L. Chen, J. Xu, J. W. Ma, G. Fang, E. B. Zhu, H. Wu, L. X. Zhao, L. Y. Wang and Y. Huang, Nano Lett., 2015, 15, 6295–6301 CrossRef PubMed.
- L. H. V. Wang and S. Hu, Science, 2012, 335, 1458–1462 CrossRef PubMed.
- L. M. Nie and X. Y. Chen, Chem. Soc. Rev., 2014, 43, 7132–7170 RSC.
- T. T. Zhuang, Y. Liu, Y. Li, Y. Zhao, L. Wu, J. Jiang and S. H. Yu, Angew. Chem., Int. Ed., 2016, 55, 6396–6400 CrossRef PubMed.
- J. N. Xuan, Z. Q. Wang, Y. Y. Chen, D. J. Liang, L. Cheng, X. J. Yang, Z. Liu, R. Z. Ma, T. Sasaki and F. X. Geng, Angew. Chem., Int. Ed., 2016, 55, 14569–14574 CrossRef PubMed.
- K. Pu, J. G. Mei, J. V. Jokerst, G. S. Hong, A. L. Antaris, N. Chattopadhyay, A. J. Shuhendler, T. Kurosawa, Y. Zhou, S. S. Gambhir, Z. N. Bao and J. H. Rao, Adv. Mater., 2015, 27, 5184–5190 CrossRef PubMed.
- K. Saha, S. S. Agasti, C. Kim, X. N. Li and V. M. Rotello, Chem. Rev., 2012, 112, 2739–2779 CrossRef PubMed.
- K. Yang, G. B. Yang, L. Chen, L. Cheng, L. Wang, C. C. Ge and Z. Liu, Biomaterials, 2015, 38, 1–9 CrossRef PubMed.
- G. Y. Liu, J. H. Zou, Q. Y. Tang, X. Y. Yang, Y. W. Zhang, Q. Zhang, W. Huang, P. Chen, J. J. Shao and X. C. Dong, ACS Appl. Mater. Interfaces, 2017, 9, 40077–40086 Search PubMed.
- Y. Wang, T. Yang, H. T. Ke, A. J. Zhu, Y. Y. Wang, J. X. Wang, J. K. Shen, G. Liu, C. Y. Chen, Y. L. Zhao and H. B. Chen, Adv. Mater., 2015, 27, 3874–3882 CrossRef PubMed.
- S. Goel, F. Chen and W. B. Cai, Small, 2014, 10, 631–645 CrossRef PubMed.
- C. Coughlan, M. Ibanez, O. Dobrozhan, A. Singh, A. Cabot and K. M. Ryan, Chem. Rev., 2017, 117, 5865–6109 CrossRef PubMed.
- Y. X. Zhao, H. C. Pan, Y. B. Lou, X. F. Qiu, J. J. Zhu and C. Burda, J. Am. Chem. Soc., 2009, 131, 4253–4261 CrossRef PubMed.
- J. M. Luther, P. K. Jain, T. Ewers and A. P. Alivisatos, Nat. Mater., 2011, 10, 361–366 CrossRef PubMed.
- G. Ku, M. Zhou, S. L. Song, Q. Huang, J. Hazle and C. Li, ACS Nano, 2012, 6, 7489–7496 CrossRef PubMed.
- M. Zhou, R. Zhang, M. A. Huang, W. Lu, S. L. Song, M. P. Melancon, M. Tian, D. Liang and C. Li, J. Am. Chem. Soc., 2010, 132, 15351–15358 CrossRef PubMed.
- M. Zhou, G. Ku, L. Pageon and C. Li, Nanoscale, 2014, 6, 15228–15235 RSC.
- X. J. Liu, B. Li, F. F. Fu, K. B. Xu, R. J. Zou, Q. Wang, B. J. Zhang, Z. G. Chen and J. Q. Hu, Dalton Trans., 2014, 43, 11709–11715 RSC.
- G. S. Song, Q. A. Wang, Y. Wang, G. Lv, C. Li, R. J. Zou, Z. G. Chen, Z. Y. Qin, K. K. Huo, R. G. Hu and J. Q. Hu, Adv. Funct. Mater., 2013, 23, 4281–4292 CrossRef.
- J. B. Cui, R. Jiang, S. Y. Xu, G. F. Hu and L. Y. Wang, Small, 2015, 11, 4183–4190 CrossRef PubMed.
- Y. Xie, A. Riedinger, M. Prato, A. Casu, A. Genovese, P. Guardia, S. Sottini, C. Sangregorio, K. Miszta, S. Ghosh, T. Pellegrino and L. Manna, J. Am. Chem. Soc., 2013, 135, 17630–17637 CrossRef PubMed.
- Z. Li, Y. J. Ji, R. G. Xie, S. Y. Grisham and X. G. Peng, J. Am. Chem. Soc., 2011, 133, 17248–17256 CrossRef PubMed.
- X. Liu, X. L. Wang, B. Zhou, W. C. Law, A. N. Cartwright and M. T. Swihart, Adv. Funct. Mater., 2013, 23, 1256–1264 CrossRef.
- H. H. Chang and C. J. Murphy, Chem. Mater., 2018, 30, 1427–1435 CrossRef.
- C. A. J. Lin, R. A. Sperling, J. K. Li, T. Y. Yang, P. Y. Li, M. Zanella, W. H. Chang and W. G. J. Parak, Small, 2008, 4, 334–341 CrossRef PubMed.
- B. Li, K. C. Ye, Y. X. Zhang, J. B. Qin, R. J. Zou, K. B. Xu, X. J. Huang, Z. Y. Xiao, W. J. Zhang, X. W. Lu and J. Q. Hu, Adv. Mater., 2015, 27, 1339–1345 CrossRef PubMed.
- N. S. Abadeer and C. J. Murphy, J. Phys. Chem. C, 2016, 120, 4691–4716 Search PubMed.
- C. M. Hessel, V. P. Pattani, M. Rasch, M. G. Panthani, B. Koo, J. W. Tunnell and B. A. Korgel, Nano Lett., 2011, 11, 2560–2566 CrossRef PubMed.
- B. B. Ding, C. Yu, C. X. Li, X. R. Deng, J. X. Ding, Z. Y. Cheng, B. G. Xing, P. A. Ma and J. Lin, Nanoscale, 2017, 9, 16937–16949 RSC.
- Y. Lyu, C. Xie, S. A. Chechetka, E. Miyako and K. Y. Pu, J. Am. Chem. Soc., 2016, 138, 9049–9052 CrossRef PubMed.
- L. M. Maestro, P. Haro-Gonzalez, A. Sanchez-Iglesias, L. M. Liz-Marzan, J. G. Sole and D. Jaque, Langmuir, 2014, 30, 1650–1658 CrossRef PubMed.
- H. J. Chen, L. Shao, T. A. Ming, Z. H. Sun, C. M. Zhao, B. C. Yang and J. F. Wang, Small, 2010, 6, 2272–2280 CrossRef PubMed.
- Q. W. Tian, F. R. Jiang, R. J. Zou, Q. Liu, Z. G. Chen, M. F. Zhu, S. P. Yang, J. L. Wang, J. H. Wang and J. Q. Hu, ACS Nano, 2011, 5, 9761–9771 CrossRef PubMed.
- Y. Chen, L. Z. Wang and J. L. Shi, Nano Today, 2016, 11, 292–308 CrossRef.
- F. Lu, J. F. Wang, L. Yang and J. J. Zhu, Chem. Commun., 2015, 51, 9447–9450 RSC.
- S. P. Sherlock, S. M. Tabakman, L. M. Xie and H. J. Dai, ACS Nano, 2011, 5, 1505–1512 CrossRef PubMed.
- X. Zhang, X. Q. Qian, C. Tao and X. J. Liu, Ultrasound Med. Biol., 2018, 44, 1110–1118 CrossRef PubMed.
- G. Gonzalez-Gaitano, J. R. Isasi, I. Velaz and A. Zornoza, Curr. Pharm. Des., 2017, 23, 411–432 CrossRef PubMed.
- J. Zhang, Z. F. Yuan, Y. Wang, W. H. Chen, G. F. Luo, S. X. Cheng, R. X. Zhuo and X. Z. Zhang, J. Am. Chem. Soc., 2013, 135, 5068–5073 CrossRef PubMed.
- C. Wang, L. Cheng and Z. Liu, Biomaterials, 2011, 32, 1110–1120 CrossRef PubMed.
- X. K. Jia, J. J. Yin, D. G. He, X. X. He, K. M. Wang, M. Chen and Y. H. Li, J. Biomed. Nanotechnol., 2013, 9, 2063–2072 CrossRef PubMed.
Footnote |
† Electronic supplementary information (ESI) available. See DOI: 10.1039/c8nh00026c |
|
This journal is © The Royal Society of Chemistry 2018 |
Click here to see how this site uses Cookies. View our privacy policy here.