DOI:
10.1039/C7NR06295H
(Paper)
Nanoscale, 2018,
10, 366-377
Core hydrophobicity tuning of a self-assembled particle results in efficient lipid reduction and favorable organ distribution†
Received
23rd August 2017
, Accepted 21st November 2017
First published on 22nd November 2017
Abstract
Atherosclerosis, the deadliest disease in the United States, arises due to the build up of plaques in the arteries as a result of excessive cholesterol deposition and an impaired cholesterol removal process. High density lipoproteins (HDL), popularly known as “good cholesterol”, are naturally occurring nano-sized particles that, along with apolipoproteins, are deployed to maintain cholesterol homeostasis in the body. Both cholesterol efflux, from the fat-laden macrophages in the arteries, and intracellular lipid transport, to deliver cholesterol to the mitochondria of liver cells for metabolism, hold key responsibilities to maintain healthy lipid levels inside the body. We designed a library of nine mitochondria targeted polymer–lipid hybrid nanoparticles (NPs), comprised of completely synthetic yet biodegradable components, that are capable of performing HDL-like functions. Using this library, we optimized a superior mitochondria targeted NP candidate, which can show favourable organ distribution, therapeutic potential, and non-toxic properties. Two targeted NP formulations with optimum NP size, zeta potential, and cholesterol binding and release properties were identified. Lipid reduction and anti-oxidative properties of these two NPs demonstrated cholesterol removal ability. In vivo therapeutic evaluation of the targeted-NP formulations in apolipoprotein E knockout (apoE−/−) mice indicated lipid reduction and anti-inflammatory properties compared to non-targeted NPs. This synthetic targeted NP with potential abilities to participate in both extra- and intracellular cholesterol transport might potentiate therapeutic interventions for heart diseases.
Introduction
Atherosclerosis is a global threat to the human race.1 Ingestion of cholesterol by macrophages within the arteries and formation of foam cells initiate and drive the process of atherosclerotic plaque formation.2 Maladaptive inflammatory responses promote plaque progression; mitochondrial dysfunctions, oxidative stress, and pro-inflammatory signals. Macrophage derived foam cells filled with high cholesterol and cholesteryl ester (CE) influence the stability of plaques and their progression.3 Cholesterol removal from foam cells by cellular cholesterol efflux is an orchestrated pathway operated by ATP-binding cassette (ABC) transporters, apolipoproteins such as apoA-I and apoE, and high-density lipoproteins (HDL).4 The extracellular cholesterol efflux by ABC transporters, apoA-I, and HDL is well studied.5,6 However, efficient removal of cholesterol from foam cells depends on intracellular lipid transport and is not well understood.7 The intracellular cholesterol transport regulates the sterol contents in organelles such as mitochondria.7 The steroidogenic acute regulatory protein (StAR)-related lipid transfer domain with a hydrophobic lipid binding pocket participates in the maintenance of cellular cholesterol.8 Thus, the relative contribution of extra- and intracellular cholesterol plays a significant role in the removal of lipids from foam cells.
The cleavage of the cholesterol side chain by a P-450 side chain cleavage enzyme is the initiation step of steroid biosynthesis and this reaction takes place at the inner mitochondrial membrane (IMM).8,9 The rate of this reaction is determined by the efficiency of the StAR protein-mediated delivery of cholesterol to the mitochondria of liver cells.10,11 Under the conditions of atherosclerosis, the limited amounts of StAR proteins are incapable of tackling the enormous amounts of cholesterol. The mitochondrial matrix contains less cholesterol since the outer mitochondrial membrane (OMM) contains more cholesterol than the IMM.12 Thus, under atherosclerotic conditions, an external agent capable of delivering cholesterol directly to the mitochondria bypassing the StAR dependent pathway could significantly enhance the rate of cholesterol metabolism. Here, we report a completely synthetic, scalable, biodegradable, and mitochondria-targeted nanoparticle (NP) with potential abilities to maintain both extra and intracellular lipid homeostasis to provide a unique therapeutic strategy for coronary heart disease (CHD) (Scheme 1).
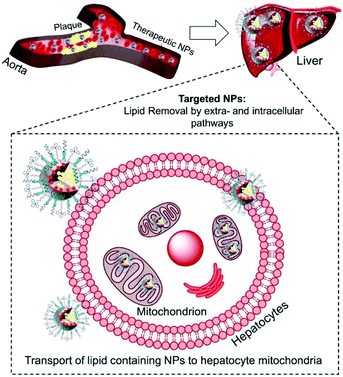 |
| Scheme 1 A pictorial representation of the potential action of a mitochondria-targeted biodegradable self-therapeutic NP with the ability to eliminate/reduce atherosclerosis by transporting lipids from plaques to the mitochondria of liver cells for metabolism. | |
HDLs are nano-sized lipoprotein particles composed of a hydrophobic lipid core that is surrounded by phospholipids and apolipoproteins.13 These lipoproteins which are known as “good cholesterol” perform reverse cholesterol transport (RCT).14 Recent studies explored the possibility of raising HDL levels in the body with the aim of reducing atherosclerosis symptoms. The use of reconstituted HDL (rHDL) in heart diseases has been extensively studied.15–22 Various other groups around the globe contributed towards the use of NPs to handle different aspects of atherosclerosis and other diseases.23–26 There are also a few examples of synthetic NPs which can potentially show HDL-like properties.27–29 To the best of our knowledge, we are the first to explore the possibility of using synthetic NPs with potential abilities to participate in both extracellular cholesterol removal and intracellular cholesterol metabolism pathways for atherosclerotic conditions. In 2013, we reported our preliminary studies on the design and synthesis of a mitochondria targeted nano-sized NP that can functionally mimic HDL.30 In this work, we describe complete composition optimization of such a NP platform for maximum therapeutic gain.
Results and discussion
Mitochondria targeted self-therapeutic NP
Based on the composition and design of naturally occurring HDLs, we recently developed a mitochondria-targeted HDL-mimicking polymer–lipid hybrid NP. This mitochondria targeted NP (T-HDL-NP) is composed of a hydrophobic core containing cholesteryl oleate (CO) and biodegradable and FDA approved poly(lactic-co-glycolic acid) (PLGA) polymer.30 This core is surrounded by a 1,2-distearoyl-sn-glycero-3-phosphoethanolamine (DSPE)–polyethylene glycol (PEG) lipid layer embedded with apoA-I mimetic L-4F peptide with the Ac-FAEKFKEAVKDYFAKFWD sequence.31 The phospholipid shell also contained stearyl-triphenylphosphonium (TPP) bromide (stearyl-TPP). The TPP cation utilizes its delocalized lipophilic positive charge to enable the construct to demonstrate mitochondria association property by taking advantage of the significant negative mitochondrial membrane potential (Δψm) that exists across the mitochondrial membranes (Fig. 1).30,32,33 For non-targeted control NP (NT-HDL-NP), poly vinyl alcohol (PVA) was used instead of stearyl-TPP. These NPs were in the size range of 100–150 nm as determined by dynamic light scattering (DLS), a range which is ∼10 times higher than that of natural HDL. The larger size of these synthetic NPs can be advantageous to avoid rapid clearance. As a result, these NPs demonstrated a long circulation lifetime with favorable biodistribution (bioD) and pharmacokinetic (PK) parameters.30 Furthermore, studies demonstrated that larger HDL might be able to lower the risk of cardiovascular diseases due to enhanced cholesterol efflux properties.34 T-HDL-NPs demonstrated unique abilities to target mitochondria of macrophages and within the mitochondria, T-HDL-NPs were localized mainly in the mitochondrial matrix and the intermembrane space (IMS)30 indicating that this T-HDL-NP has the potential to be able to participate in the intracellular cholesterol transport pathway. Preliminary binding studies with 22-(N-(7-nitrobenz-2-oxa-1,3-diazol-4-yl)amino)-23,24-bisnor-5-cholen-3β-ol or NBD-cholesterol indicated that T-HDL-NPs were capable of binding cholesterol with high efficiency.30
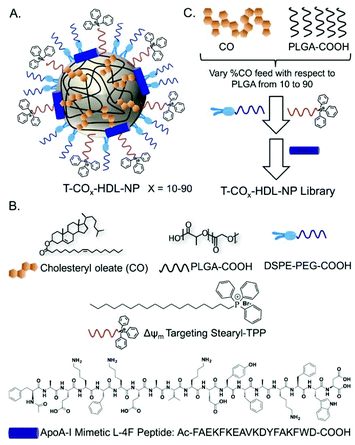 |
| Fig. 1 (A) Schematic representation of possible structural orientation of T-HDL-NPs. (B) Various components and their structures used in the construction of synthetic T-HDL-NPs. (C) A cartoon demonstrating variation in the components to generate a library of T-HDL-NPs. | |
Each of the components in the T-HDL-NP construct serves a unique purpose, which can result in the therapeutic potential of these NPs. Thus, we attempted to determine the optimal composition in order to tune and achieve maximum therapeutic potential. We synthesized a library of T-HDL-NPs by varying the ratio of CO to PLGA (Fig. 1A). This library of NPs follows a trend in terms of CO loading, size, zeta potential, and cholesterol binding efficiencies (Fig. 2A). As the percentage feed of CO was increased, % CO loading increased, and % CO encapsulation efficiency (EE) decreased (Fig. 2A, Table S1†). The size and zeta potential trends of this NP library indicated that only by adding a suitable amount of PLGA (0.5 mg in total NP), we are able to achieve stable NPs with suitable size and charge (Fig. 2A, Table S1†). At a low percentage feed of CO such as NPs synthesized with 10–20% CO feed, a large diameter and heterogeneous population were demonstrated (Table S1, Fig. 2A and Fig. S1†). Further, transmission electron microscopy (TEM)-based morphological analyses also indicated that NPs at a low CO percentage feed demonstrated some degree of aggregation (Fig. S2†). A library of NT-HDL-NPs was constructed from PLGA-COOH, CO, PVA, DSPE-PEG-COOH, and 4F peptide through a modified nanoprecipitation method as described for T-HDL-NPs by varying the ratio of PLGA to CO (Table S2, Fig. S1†). NT-HDL-NPs demonstrated similar size range as seen with T-HDL-NPs and had a negative zeta potential. The morphologies of T-HDL-NPs and NT-HDL-NPs were confirmed by TEM (Fig. 2B, Fig. S2 and S3†). A comparison of the morphologies of T and NT-HDL NPs indicated a similar pattern. Stability studies performed with T-CO40-HDL and T-CO70-HDL NPs by storing the aqueous solutions at 37 °C and analyses of the NPs in terms of their size and zeta potential indicated that these are highly stable at least over a period of 3 weeks (Fig. S4†).
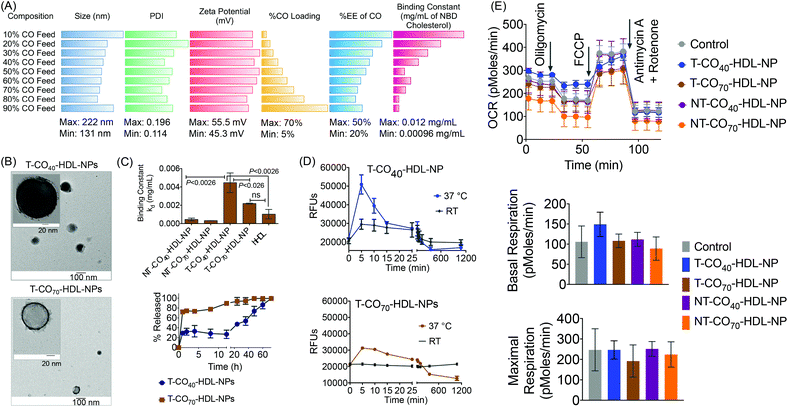 |
| Fig. 2 (A) Diameter, PDI, and zeta potential as determined by dynamic light scattering; % loading and % EE of CO in T-HDL-NP library as determined by the Amplex Red assay, and cholesterol binding constants in the library of HDL mimicking NPs constructed by varying the ratio of CO to PLGA in the core. (B) TEM of 40% and 70% CO feed T-HDL-NPs. (C) Top: Comparison of NBD-cholesterol binding constants at 6 h of T-CO40-HDL-NP, T-CO70-HDL-NP, NT-CO40-HDL-NP, NT-CO70-HDL-NP, and hHDL. Bottom: Release of bound NBD-cholesterol from T-CO40-HDL-NPs and T-CO70-HDL-NPs under the physiological pH of 7.4 at 37 °C. (D) Comparison of NBD-cholesterol binding kinetics with T-CO40-HDL-NPs and T-CO70-HDL-NPs at room temperature and 37 °C. (E) The effect of T/NT-CO40-HDL-NPs and T/NT-CO70-HDL-NPs (20 μg mL−1) on the mitochondrial respiration of H9C2 cardiomyocytes as determined by the MitoStress assay using a Seahorse Analyzer. | |
Optimization of self-therapeutic NPs for cholesterol binding and efflux
An in vitro cholesterol-binding assay using NBD-cholesterol with NP libraries provided insight pertaining to the optimized CO
:
PLGA ratio so as to maximize the efficiency of cholesterol binding ability of T-HDL-NPs (Fig. 2C and Fig. S5†). A time dependent cholesterol binding assay indicated that at low % CO feed with respect to PLGA, the T-HDL-NPs demonstrated the highest cholesterol binding ability. As the % CO feed was increased, the cholesterol-binding affinity of the NPs was reduced (Fig. S6†). Binding constants (kd) were calculated by analyzing the binding curves obtained from the T-HDL-NP library at a constant time point of 6 h with “one site total binding” function in GraphPad Prism 5.0 software using the equation: RFU = (Bmax × [NBD-cholesterol])/(kd + [NBD-cholesterol]). We used incremental concentrations of NBD-cholesterol keeping the concentration of NPs constant. The high kd values obtained for the NPs with low CO feed imply that NPs were capable of binding large amounts of NBD-cholesterol. Moderately high values for kd were obtained for the NPs in the range of 40–70% CO feed (Fig. 2C and Fig. S6†). The NBD-cholesterol binding ability of human HDL (hHDL) was also studied to serve as a positive control (Fig. 2C and Fig. S7†). The binding constant for hHDL, when compared to that of T-CO40-HDL-NP, indicated that the binding affinity of NBD-cholesterol towards 40% CO feed T-HDL-NP is significantly higher (P < 0.0026). The kd value for the T-CO70-HDL-NPs was comparable to that for hHDL and significantly lower than the T-CO40-HDL-NP (P < 0.026).
The NT-HDL-NPs, on the other hand, demonstrated significantly reduced cholesterol binding properties compared to T-HDL-NPs (Fig. S8 and S9†). This observation led us to pose a question whether the negatively charged NBD-cholesterol is getting adsorbed onto the positively charged surface of T-NPs. Control binding experiments performed with a cationic molecule TPP-(CH2)5-COOH and micelles constructed from stearyl-TPP demonstrated that neither stearyl-TPP nor the micelles bind to NBD-cholesterol efficiently (Fig. S10†). This led us to conclude that the positive surface charge on the NPs is not the sole factor that determines efficacious cholesterol binding. We believe that the positive charge plays significant role in attracting the cholesterol followed by incorporation of cholesterol into the hydrophobic core and lipid layer of this NP. Thus, it is the combination of positive charge, core hydrophobicity, and unique composition comprising of a lipid layer and apoA-I mimetic peptide which contributes to efficacious cholesterol binding abilities of T-HDL-NPs. NPs with 40–70% CO feed showed optimum NP size and differential cholesterol binding kinetics; therefore taking these parameters into account, we decided to use NPs with 40% (T-CO40-HDL-NP) and 70% (T-CO70-HDL-NP) CO feed to carry out in vitro and in vivo therapeutic studies. The corresponding non targeted NPs were used as controls.
We investigated release kinetics of bound NBD-cholesterol from the T-HDL-NPs (Fig. 2C, bottom). Under physiologically relevant conditions (pH 7.4 and 37 °C temperature), the release of bound NBD-cholesterol from T-CO40-HDL-NPs, which had a lower CO content, was found to follow a more controlled fashion compared to T-CO70-HDL-NPs that had 70% CO feed (Fig. 2C). From the cholesterol release kinetics, it was clear that these NPs release bound cholesterol in a controlled fashion at 37 °C; hence we next assessed the cholesterol binding kinetics at this temperature and compared the binding efficiencies with room temperature (RT) (Fig. 2D). It was evident that both the formulations demonstrate enhanced cholesterol binding and better release of bound cholesterol at 37 °C when compared to room temperature.
Effect of T-HDL-NPs in cardiomyoblasts
We previously reported the mitochondrial association properties of T-HDL-NPs.30 The current formulations, T-CO40-HDL-NP and T-CO70-HDL-NP, were tested in H9C2 cardiomyoblasts for determination of their effects on mitochondrial respiration (Fig. 2E and Fig. S11†). H9C2 cells are rat ventricular cardiomyoblasts that have hyperpolarized mitochondrial membrane potential. MitoStress analyses on H9C2 cells in the presence of T/NT-CO40-HDL-NP or T/NT-CO70-HDL-NP using the Seahorse Analyzer suggested that these NPs do not cause any inhibition of mitochondrial respiration up to a concentration of 20 μg mL−1 (Fig. 2E). At a higher concentration of 50 μg mL−1, T-CO40-HDL-NPs demonstrated some degree of inhibition of mitochondrial respiration (Fig. S11†).
Therapeutic potential of targeted self-therapeutic NPs using macrophage derived foam cells
Cholesterol accumulation in the arterial wall macrophages resulting in foam cell formation is one of the initial steps of atherogenesis. During foam cell formation, the balance between cholesterol influx and efflux in macrophages becomes dysfunctional.35 Under a well-maintained cholesterol homeostasis environment, there exists a balanced equilibrium between cholesterol influx through scavenger receptors and efflux through RCT. We investigated the athero-protective effects of T-HDL-NPs on RAW 264.7 macrophage-derived foam cells36–38 formed by oxidized LDL (oxLDL). RAW macrophages were treated with oxLDL (100 μg mL−1) to induce foam cell formation (Fig. 3 and Fig. S12†).36 Foam cells derived from macrophages in the presence of oxLDL demonstrate inflammatory signaling, cholesterol accumulation, and oxidative stress similar to the hallmark of early atherosclerotic lesions. To examine the therapeutic potential, T-CO40-HDL-NPs and T-CO70-HDL-NPs were used, and NP treatment was carried out prior to or after the addition of oxLDL to gain insight about both the preventive and therapeutic potential of these NPs. As controls, hHDL isolated from blood bank produced human plasma was used under both therapeutic and preventive settings. For preventative treatment, T-CO40-HDL-NPs, T-CO70-HDL-NPs, or hHDL were added and allowed to internalize for 6 h. The media was changed and fresh RPMI supplemented with oxLDL was added. The cells were further incubated for 24 h. For therapeutic foam cell treatment, after 24 h of treatment with lipid deprived media, the media was replaced with RPMI supplement with oxLDL and the cells were incubated for 12 h. After that, the media was removed and NPs (T-CO40-HDL-NPs, T-CO70-HDL-NPs) or hHDL were added. Effects on total cholesterol, triglyceride, and anti-oxidative effects of these NPs and hHDL on foam cells were studied (Fig. 3 and 4).
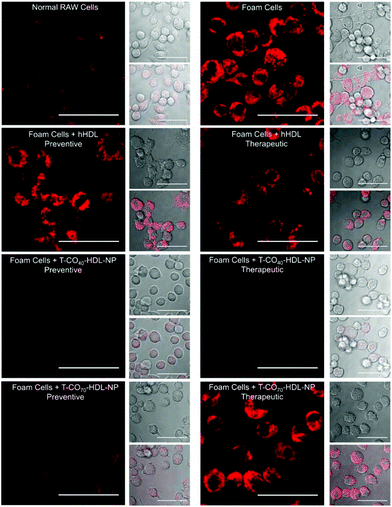 |
| Fig. 3 Intracellular lipid modulation by T-CO40-HDL-NPs and T-CO70-HDL-NPs in foam cells under preventive and therapeutic settings using live cell imaging. For foam cell formation, RAW 264.7 macrophages were treated with oxLDL. Treatment with hHDL, T-CO40-HDL-NPs, or T-CO70-HDL-NPs was carried out before the addition of oxLDL under preventive setting and after treatment with oxLDL under the therapeutic setting. Intracellular lipids were stained using AdipoRed and live cell imaging was performed. Representative AdipoRed stained (red) images showed the formation of foam cells from RAW 264.7 macrophages upon treatment with oxLDL. Scale bar: 25 μm. | |
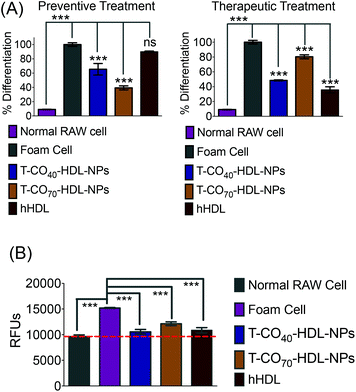 |
| Fig. 4 Lipid reduction by T-CO40-HDL-NPs and T-CO70-HDL-NPs in foam cells under preventive and therapeutic settings using the AdipoRed assay. For foam cell formation, RAW 264.7 macrophages were treated with oxLDL. (A) Treatment with hHDL, T-CO40-HDL-NPs, and T-CO70-HDL-NPs were carried out before the addition of oxLDL under preventive settings and after treatment with oxLDL under therapeutic settings. Intracellular triglyceride contents were stained using AdipoRed and quantified using a plate reader. (B) Increased ROS levels after foam cell formation and subsequent reduction in oxidative stress after treatment with T-CO40-HDL-NPs or T-CO70-HDL-NPs or hHDL. *, **, ***P value 0.01–0.05, 0.001–0.01, and <0.001, respectively. ns: nonsignificant. | |
Intracellular triglyceride contents were stained using AdipoRed and live cell imaging was performed to analyze the lipid droplets in foam cells in the absence and presence of T-HDL-NPs or hHDL (Fig. 3). Both methods of treatment indicated that T-CO40-HDL-NPs have the ability to reduce intracellular lipid levels in macrophages (Fig. 3). The lipid reduction abilities of T-CO70-HDL-NP were found to be better under preventive settings compared to the abilities seen under therapeutic treatment (Fig. 3). Native hHDL demonstrated more efficient lipid reduction profiles under therapeutic treatment (Fig. 3). Quantification of intracellular triglyceride contents using the AdipoRed assay was used to calculate the extent of macrophage transformation into foam cells in the presence of oxLDL (Fig. 4A). Both methods of treatment indicated that T-CO40-HDL-NPs and T-CO70-HDL-NPs have the abilities to reduce macrophage derived foam cell formation (Fig. 4A). Native hHDL was able to reduce this transformation only under therapeutic settings (Fig. 4A). Quantification of reactive oxygen species (ROS) in the foam cells demonstrated elevated oxidative stress compared to normal macrophages (Fig. 4B). Treatment of foam cells with T-CO40-HDL-NPs or T-CO70-HDL-NPs or hHDL attenuated the increased ROS levels in foam cells. The extent of ROS reduction by the NPs was comparable to the effects shown by natural hHDL (Fig. 4B).
Cholesterol efflux property of mitochondria targeted self-therapeutic NPs in RAW 264.7 and smooth muscle cells
RCT is a process of cholesterol transport from lipid-laden macrophages to the liver tissues.39 Thus, the cholesterol efflux capacity is an important factor to evaluate the therapeutic efficacy of HDL-mediated atheroprotection via RCT.40,41 NBD-cholesterol undergoes hydrolysis by about 8% in 24 h.42,43 Thus, NBD-cholesterol can be used for the analysis of cholesterol efflux capacity when conducted within 24 h.42,44 We conducted a cholesterol efflux study in RAW 264.7 macrophages in the presence of T/NT-CO40/70-HDL-NPs (Fig. 5A). NBD-cholesterol treated cells, when incubated with T-HDL-NPs, showed excellent cholesterol efflux property (Fig. 5A). The ratio of cholesterol present in the media to that retained by the cells gives a measure of cholesterol efflux property of the NPs. The results indicated that the T-CO40-HDL-NPs were the best in removing cholesterol from RAW264.7 macrophages when compared to T-CO70-HDL-NPs or the non-targeted analogues (Fig. 5A). Similar trends in the cholesterol efflux property of the T-HDL-NPs were also observed in smooth muscle cells (SMCs) isolated from normal mouse aorta (Fig. 5A). The contractile nature of the SMCs was ensured by staining the α-smooth muscle actin and imaging the stained cells under a confocal microscope (Fig. 5B). These results further established the potential of the mitochondria targeted self-therapeutic NPs in the context of anti-atherosclerotic treatment.
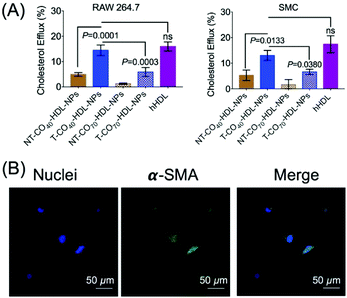 |
| Fig. 5 (A) Cholesterol efflux from RAW 264.7 cells and SMCs in the presence of T/NT-CO40/70-HDL-NPs. (B) Contractile nature of aortic SMCs as evident from the staining of α-smooth muscle actin (α-SMA). | |
Biodistribution, immunogenicity, and lipid modulation properties of T/NT-CO40/70-HDL-NPs in normal mice
We previously reported the PK and bioD properties of T-CO75-HDL-NPs in Sprague Dawley rats, which demonstrated favorable profiles.30 The T-CO75-HDL-NPs were mostly distributed in the heart. The ability of therapeutic agents to distribute in the target organs in vivo is of importance in order to assess the therapeutic potential. We set out to determine the bioD properties of T/NT-CO40/70-HDL-NPs in BALB/c albino mice. T-CO40-HDL-NP or T-CO70-HDL-NPs or their nontargeted controls containing quantum dots (QDs) for detection were administered in Balb/c albino mice at a dose of 10 mg kg−1 with respect to total NP (0.24 mg kg−1 for T-CO40, 0.28 mg kg−1 for T-CO70, 0.31 mg kg−1 for NT-CO40, and 0.33 mg kg−1 for NT-CO70 with respect to Cd) by intravenous injection. We used a polymer conjugated QD, PLGA-PEG-QD in these NP formulations.30,32,45 Detailed characterizations of these NPs are listed in Table S3.† Schematic representations of T/NT-CO40/70-HDL-QD-NPs are shown in Fig. 6A, top row. QD incorporated HDL-NPs can be seen from un-stained TEM images (Fig. 6A, middle row). Monodispersed populations of T/NT-CO40/70-HDL-QD-NPs can be predicted from the size distribution pattern obtained from dynamic light scattering (DLS) measurements and the surface charge of these NPs corresponded to positively charged T-NPs and negatively charged NT-NPs (Fig. 6A, bottom row). Normal mice were intravenously injected with 10 mg kg−1 of T/NT-CO40/70-HDL-QD-NPs and after 24 h treatment; serum samples were analyzed for triglyceride, total cholesterol, and LDL; bioD was followed by inductively coupled plasma mass spectrometry (ICP-MS). BioD profiles for the NPs in BALB/c mice indicated that the targeted NPs (T-CO40/70-QD-HDL-NPs) have an inherent tendency to localize in the heart, aorta, and liver tissues (Fig. 6B). The T-CO40-QD-HDL-NPs localize predominantly in the aorta and liver whereas the T-CO70-QD-HDL-NPs distribute mostly in the heart and liver (Fig. 6B). The non-targeted NPs (NT-CO40/70-QD-HDL-NPs) distributed mostly in the lungs, liver, and spleen (Fig. 6B). These observations make T-CO40-QD-HDL-NPs ideal for anti-atherosclerotic applications as these NPs are capable of localizing predominantly in the aorta where plaques are localized. Thus, these NPs will be able to collect lipids from the plaques which are mainly located in the aortic wall and transport the lipids to the liver for lipid metabolism since the clearance of these NPs possibly happens through the liver. The reason why the T-CO40-QD-HDL-NPs mostly accumulate in the aorta and the exact fate of the NPs in circulation, in the aorta, and liver needs further investigations.
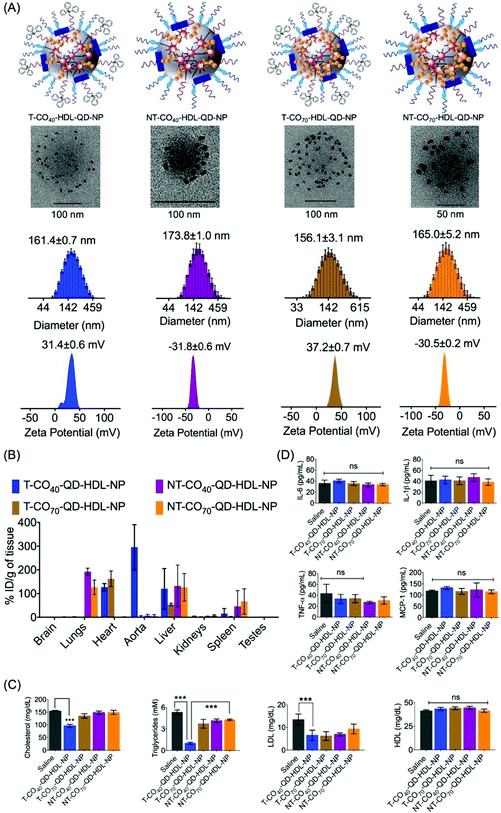 |
| Fig. 6 (A) Schematic representation of the NP formulations used for the bioD study in BALB/c mice, TEM images of the respective NPs, diameter, and zeta potentials of the NPs as determined by DLS. (B) Accumulation of T-CO40-HDL-NPs in the aorta and T-CO70-HDL-NPs in the heart of normal BALB/C mice. T/NT-CO40-QD-HDL-NPs or T/NT-CO70-QD-HDL-NPs (10 mg kg−1 with respect to total NP) were administered by intravenous injection. NPs were quantified by ICP-MS in organ samples. (C) Levels of serum total cholesterol, triglycerides, LDL, and HDL from the animals administered with T/NT-CO40-HDL-NPs and T/NT-CO70-HDL-NPs. (D) Levels of serum cytokines IL-6, TNF-α, IL-1β, and chemokine MCP-1 from the treated animals. *, **, ***P value 0.01–0.05, 0.001–0.01, and <0.001, respectively. ns: non-significant. | |
The lipid level analyses of the blood serum of T-CO40-QD-HDL-NP treated mice showed significant reduction in triglycerides (saline vs. T-CO40-QD-HDL-NPs, P < 0.0001) and cholesterol (saline vs. T-CO40-QD-HDL-NPs, P < 0.0001) (Fig. 6C). Since these are normal mice and are not challenged with high amounts of lipid, the T-CO40-HDL-NPs were able to get rid of the body lipids as quickly as 24 h. The LDL levels in the blood serum of T-CO40-QD-HDL-NP treated group mice were lower than that in the saline treated animals whereas the HDL, the good cholesterol levels, were similar in all the groups (Fig. 6C).
The induction of an immunogenic response in vivo by the NPs was studied by analyzing pro-inflammatory cytokines tumor necrosis factor-α (TNF-α), interleukin-6 (IL-6), and IL-1β in serum using the enzyme-linked immunosorbent assay (ELISA). There was no significant difference in the levels of TNF-α, IL-6, and IL-1β among serum samples when treated with T/NT-CO40-HDL-NPs or T/NT-CO70-HDL-NPs compared to the saline control group (Fig. 6D). Analyses of monocyte chemoattractant protein-1 (MCP-1), the key chemokine which is related to the migration and infiltration of monocytes or macrophages, in the NP treated serum samples indicated no enhancement or decrease of this chemokine (Fig. 6D). These studies show the non-immunogenic nature of these T/NT-HDL-NPs at the doses used in this mice study.
Therapeutic potential of T-CO40-HDL-NPs in apoE−/− mice
To evaluate the therapeutic potential of T-CO40-HDL-NP towards atherosclerosis treatment, we chose the apoE−/− mouse model as it would be a clinically relevant model for the study. A cohort of apoE−/− mice were fed with normal chow diet and treated with saline (n = 3), T-CO40-HDL-NPs (n = 3), or NT-CO40-HDL-NPs (n = 3) to understand the lipid reduction profiles of mitochondria-targeted self-therapeutic NPs in contrast to the non-targeted NPs (Table S4† for NP characterization). Saline, T-CO40-HDL-NPs, or NT-CO40-HDL-NPs (10 mg kg−1 with respect to total NP) were injected intravenously via the tail vein twice weekly for a total of 7 weeks. Blood samples were collected before starting the treatment to determine the serum lipid levels in all the animals. Serum samples were isolated on predetermined days; total cholesterol, triglyceride, and LDL levels were quantified. NT-CO40-HDL-NPs and saline treated animals served as control groups. Predose total cholesterol, triglyceride, and LDL levels were comparable in the animals from different groups (Fig. 7A). Quantification of serum lipid levels from different groups at the end of the study indicated that T-CO40-HDL-NP treated animals have significantly reduced levels of triglyceride, total cholesterol, and LDL compared to the control group injected with saline (Fig. 7A). The total cholesterol level in the T-CO40-HDL-NP treated group was significantly lower compared to the NT-CO40-HDL-NP group; the group which was treated with non-targeted NPs also demonstrated reduced total cholesterol compared to the saline group (Fig. 7A). A comparison of predose total cholesterol and LDL with those of saline, T-CO40-HDL-NP, and NT-CO40-HDL-NP treated groups on day 18 clearly demonstrated the efficacy of the targeted NPs to reduce lipid levels (Fig. 7A, bottom).
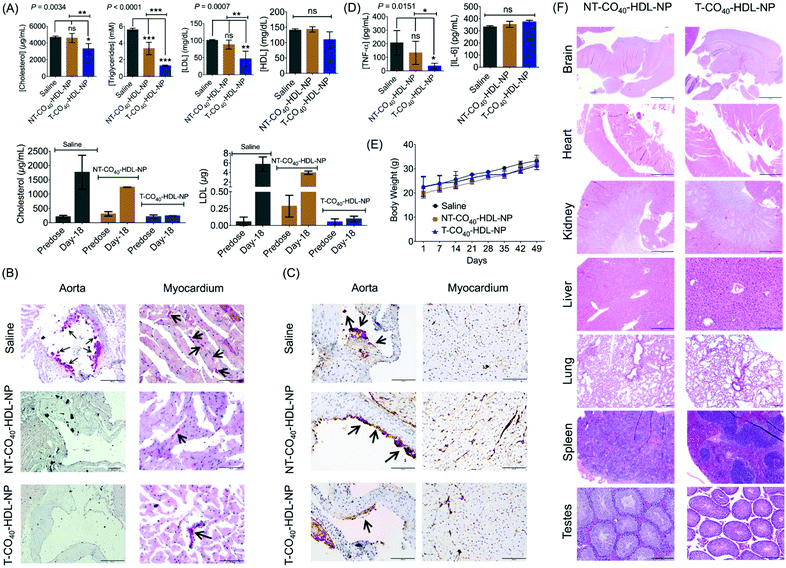 |
| Fig. 7 (A) Therapeutic efficacy of T-CO40-HDL-NP and NT-CO40-HDL-NP in the apoE−/− mouse model fed with normal diet. Animals (n = 3 for each group) were treated with T-CO40-HDL-NPs or NT-CO40-HDL-NPs at a dose of 10 mg kg−1 (with respect to total NP) twice weekly via intravenous injection. Serum lipid levels were compared between the treatments by quantifying triglycerides, total cholesterol, LDL, and HDL. (B) Representative image of Oil Red O staining for the aorta and myocardium showing no foam cells with T-CO40-HDL-NP or NT-CO40-HDL-NP treatment in the aorta and linear staining of the small vessel lumens in the myocardium. (C) Representative images of caspase 3 apoptotic staining in the aorta and myocardium from apoE−/− mice treated with saline, T-CO40-HDL-NP or NT-CO40-HDL-NP (D) Pro-inflammatory IL-6 and TNF-α cytokine level in the serum at the end of the study. (E) Average body weight of apoE−/− animals during the course of the study demonstrating the non-toxic nature of the NPs. (F) Toxicity of targeted HDL NPs in major organs from the apoE−/− mouse model fed with normal diet by histopathological analyses with hematoxylin and eosin staining. Scale bar = 100 μm. | |
Multifocal accumulations of macrophages lining the aortic valve and aorta have enlarged vacuolated cytoplasm that stained positive for lipid (e.g., triglycerides) by Oil Red O staining was observed with the saline treated group (Fig. 7B). Photographs of the aortic valve with Oil Red O positive macrophages on the surface can be seen with the saline treated group. However, there were little to no foam cells or Oil Red O staining along the endothelium of either the T-CO40-HDL-NP or NT-CO40-HDL-NP treated groups (Fig. 7B). Within the myocardium, there was linear Oil Red O staining of the small vessel lumens and myocytes contained minute positive lipid droplets in all the groups.
Caspase 3 plays a major role in the execution of the cascade of events during cellular apoptosis and has been used for apoptosis detection in tissues.46,47 The apoptosis of macrophages in plaques is actively involved in the status and progression of atherosclerosis in all stages.48 Apoptosis staining of caspase 3 was carried out to interpret the apoptotic status of early lipid laden macrophages. Cytoplasmic staining for caspase 3 (brown) was moderate in lipid laden macrophages adhered to the aortic valve (long arrow; left photo) but staining was not evident in adjacent endothelial cells (short arrow; left photo). Within the myocardium (right photo) there is severe staining, mostly cytoplasmic but also nuclear, of endothelial cells (dark brown) lining small vessels within the myocardium (Fig. 7C). At the arrow, the dark staining may be partially due to plaque deposition. Saline treated animals showed more lipid laden macrophages in comparison with the NP treated group. The difference between T and NT-HDL-CO40-NP treated groups was not very significant from this staining. A larger group of animals might reveal more statistically relevant results. Cytokine analysis in serum samples showed significant reduction in TNF-α with the T-HDL-CO40-NP treated group in comparison with saline and NT-NP treated mice (Fig. 7D). No change in IL-6 level was observed with respect to saline. Further, body weight data indicated that there is no toxicity associated with multiple dosing of T and NT-HDL-CO40-NPs (Fig. 7E). Histopathological examinations are shown in Fig. 7F. Hematoxylin and eosin staining of major tissues further supported no toxic effects from the NP treatments.
Conclusions
The NP platform presented here could decrease plaque formation directly by locally accumulating a HDL mimic, working at the intracellular cholesterol metabolism pathways by targeting mitochondria. This study indicated the therapeutic potential of T-CO40-HDL-NPs and also highlighted the advantages of mitochondria targeted HDL mimicking NPs over the non-targeted formulation. The rate at which cholesterol is transferred to the mitochondria determines the rate of cholesterol degradation in the liver. Therefore, mitochondria targeted T-HDL-NPs will be able to carry excess cholesterol from the cytosol to the mitochondria for cholesterol metabolism, thus maintaining proper equilibrium for intracellular lipids. This technology uses biocompatible polymers and lipids to create a synthetic yet biodegradable HDL mimic. The platform presented here is synthetic, biodegradable, and non-toxic and the mitochondria targeted HDL mimicking NP has the potential to participate in intracellular lipid homeostasis.
Experimental
Materials and methods
Description of materials and all methods are described in the ESI.†
Animals
Balb/c albino mice (4 weeks old) were purchased from Jackson Laboratory. ApoE−/− mice aged five weeks (Jackson Laboratory, Bar Harbor, ME) were fed a normal chow diet. All animals were handled in accordance with “The Guide for the Care and Use of Laboratory Animals” of the American Association for Accreditation of Laboratory Animal Care (AAALAC), Animal Welfare Act (AWA), and other applicable federal and state guidelines. All animal work presented here was approved by the Institutional Animal Care and Use Committee (IACUC) of the University of Miami Miller School of Medicine and the University of Georgia. All housing, surgical procedures, and experimental protocols were approved by the IACUC Committee of UM and UGA. Animals had free access to chow diet and water during all experiments.
Statistics
All data were expressed as mean ± S.D. (standard deviation). Statistical analyses were performed using GraphPad Prism® software v. 5.00. Comparisons between two values were performed using an unpaired Student's t test. A one-way ANOVA with a post-hoc Tukey test was used to identify significant differences among the groups.
Cell lines and cell culture
Mouse macrophage RAW 264.7 cells were procured from American Type Culture Collection (ATCC). H9C2 cardiomyocytes was given as a generous gift from Prof. Mark Anderson, University of Iowa. Both RAW 264.7 cells and cardiomyocytes were grown in Dulbecco's Modified Eagle's Medium (DMEM) supplemented with 4 mM L-glutamine, 1.5 g L−1 sodium pyruvate, 4.5 g L−1 glucose, 1% penicillin/streptomycin, and 10% fetal bovine serum. Cells were passed every 2–3 days and restarted from frozen stocks upon reaching pass 20. The mouse aortic smooth muscle cells were cultured in DMEM: nutrient mixture F-12 (DMEM/F-12) media supplemented with 4 mM L-glutamine, 1.5 g L−1 sodium pyruvate, 4.5 g L−1 glucose, 1% penicillin/streptomycin, and 5% fetal bovine serum.
Author contribution
S. D. designed the research and supervised experiments; B. B., R. W., S. M., A. K., and N. K. performed research; B. B., R. W., and S. M. contributed reagents; N. K. helped in designing the experiments and supervised R. W. in initial experiments; A. K. assisted in experiments with Balb/c and apoE−/− mice; E. W. H. performed and analyzed histopathology studies; B. B., R. W., S. M., N. K., E. W. H., and S. D. analyzed the data; and B. B., N. K., R. W., and S. D. wrote the paper. All authors discussed the results and commented on the manuscript.
Conflicts of interest
S. D. and N. K. had financial interests in Partikula LLC.
Acknowledgements
This work was supported, in whole or in part, by the National Institutes of Health Grant R56HL121392 from the National Heart, Lung, and Blood Institute and the American Heart Association National Scientist Award 14SDG18690009 to S. D., and Sylvester Comprehensive Cancer Center. We thank Lei Song for providing us with SMCs and Dr Roberto Vazquez-Pardon for useful discussions.
Notes and references
- J. A. Finegold, P. Asaria and D. P. Francis, Mortality from ischaemic heart disease by country, region, and age: statistics from World Health Organisation and United Nations, Int. J. Cardiol., 2013, 168(2), 934–945 CrossRef PubMed.
- R. Ross, The pathogenesis of atherosclerosis - a perspective for the 1990s, Nature, 1993, 362(6423), 801–809 CrossRef CAS PubMed.
- S. Ghosh, Macrophage cholesterol homeostasis and metabolic diseases: critical role of cholesteryl ester mobilization, Expert Rev. Cardiovasc. Ther., 2011, 9(3), 329–340 CrossRef CAS PubMed.
- J. F. Oram, HDL apolipoproteins and ABCA1: partners in the removal of excess cellular cholesterol, Arterioscler., Thromb., Vasc. Biol., 2003, 23(5), 720–727 CrossRef CAS PubMed.
- J. F. Oram, ATP-binding cassette transporter A1 and cholesterol trafficking, Curr. Opin. Lipidol., 2002, 13(4), 373–381 CrossRef CAS PubMed.
- G. H. Rothblat, M. de la Llera-Moya, V. Atger, G. Kellner-Weibel, D. L. Williams and M. C. Phillips, Cell cholesterol efflux: integration of old and new observations provides new insights, J. Lipid Res., 1999, 40(5), 781–796 CAS.
- E. Ikonen, Mechanisms for cellular cholesterol transport: defects and human disease, Physiol. Rev., 2006, 86(4), 1237–1261 CrossRef CAS PubMed.
- W. L. Miller and H. S. Bose, Early steps in steroidogenesis: intracellular cholesterol trafficking, J. Lipid Res., 2011, 52(12), 2111–2135 CrossRef CAS PubMed.
- J. Hu, Z. Zhang, W. J. Shen and S. Azhar, Cellular cholesterol delivery, intracellular processing and utilization for biosynthesis of steroid hormones, Nutr. Metab., 2010, 7, 47 Search PubMed.
- Y. Ning, Q. Bai, H. Lu, X. Li, W. M. Pandak, F. Zhao, S. Chen, S. Ren and L. Yin, Overexpression of mitochondrial cholesterol delivery protein, StAR, decreases intracellular lipids and inflammatory factors secretion in macrophages, Atherosclerosis, 2009, 204(1), 114–120 CrossRef CAS PubMed.
- E. Ikonen, Cellular cholesterol trafficking and compartmentalization, Nat. Rev. Mol. Cell Biol., 2008, 9(2), 125–138 CrossRef CAS PubMed.
- H. S. Bose, V. R. Lingappa and W. L. Miller, Rapid regulation of steroidogenesis by mitochondrial protein import, Nature, 2002, 417(6884), 87–91 CrossRef CAS PubMed.
- P. G. Yancey, M. de la Llera-Moya, S. Swarnakar, P. Monzo, S. M. Klein, M. A. Connelly, W. J. Johnson, D. L. Williams and G. H. Rothblat, High Density Lipoprotein Phospholipid Composition Is a Major Determinant of the Bi-directional Flux and Net Movement of Cellular Free Cholesterol Mediated by Scavenger Receptor BI, J. Biol. Chem., 2000, 275(47), 36596–36604 CrossRef CAS PubMed.
- T. F. Daniels, K. M. Killinger, J. J. Michal, R. W. Wright Jr. and Z. Jiang, Lipoproteins, cholesterol homeostasis and cardiac health, Int. J. Biol. Sci., 2009, 5(5), 474–488 CrossRef CAS PubMed.
- B. L. Sanchez-Gaytan, F. Fay, M. E. Lobatto, J. Tang, M. Ouimet, Y. Kim, S. E. van der Staay, S. M. van Rijs, B. Priem, L. Zhang, E. A. Fisher, K. J. Moore, R. Langer, Z. A. Fayad and W. J. Mulder, HDL-Mimetic PLGA Nanoparticle To Target Atherosclerosis Plaque Macrophages, Bioconjugate Chem., 2015, 26(3), 443–451 CrossRef CAS PubMed.
- M. E. Lobatto, C. Calcagno, A. Millon, M. L. Senders, F. Fay, P. M. Robson, S. Ramachandran, T. Binderup, M. P. Paridaans, S. Sensarn, S. Rogalla, R. E. Gordon, L. Cardoso, G. Storm, J. M. Metselaar, C. H. Contag, E. S. Stroes, Z. A. Fayad and W. J. Mulder, Atherosclerotic plaque targeting mechanism of long-circulating nanoparticles established by multimodal imaging, ACS Nano, 2015, 9(2), 1837–1847 CrossRef CAS PubMed.
- R. Duivenvoorden, J. Tang, D. P. Cormode, A. J. Mieszawska, D. Izquierdo-Garcia, C. Ozcan, M. J. Otten, N. Zaidi, M. E. Lobatto, S. M. van Rijs, B. Priem, E. L. Kuan, C. Martel, B. Hewing, H. Sager, M. Nahrendorf, G. J. Randolph, E. S. Stroes, V. Fuster, E. A. Fisher, Z. A. Fayad and W. J. Mulder, A statin-loaded reconstituted high-density lipoprotein nanoparticle inhibits atherosclerotic plaque inflammation, Nat. Commun., 2014, 5, 3065 Search PubMed.
- A. Barazza, C. Blachford, O. Even-Or, V. A. Joaquin, K. C. Briley-Saebo, W. Chen, X. C. Jiang, W. J. Mulder, D. P. Cormode, Z. A. Fayad and E. A. Fisher, The complex fate in plasma of gadolinium incorporated into high-density lipoproteins used for magnetic imaging of atherosclerotic plaques, Bioconjugate Chem., 2013, 24(6), 1039–1048 CrossRef CAS PubMed.
- T. Skajaa, Y. Zhao, D. J. van den Heuvel, H. C. Gerritsen, D. P. Cormode, R. Koole, M. M. van Schooneveld, J. A. Post, E. A. Fisher, Z. A. Fayad, C. de Mello Donega, A. Meijerink and W. J. Mulder, Quantum dot and Cy5.5 labeled nanoparticles to investigate lipoprotein biointeractions via Forster resonance energy transfer, Nano Lett., 2010, 10(12), 5131–5138 CrossRef CAS PubMed.
- T. Skajaa, D. P. Cormode, P. A. Jarzyna, A. Delshad, C. Blachford, A. Barazza, E. A. Fisher, R. E. Gordon, Z. A. Fayad and W. J. Mulder, The biological properties of iron oxide core high-density lipoprotein in experimental atherosclerosis, Biomaterials, 2011, 32(1), 206–213 CrossRef CAS PubMed.
- D. P. Cormode, J. C. Frias, Y. Ma, W. Chen, T. Skajaa, K. Briley-Saebo, A. Barazza, K. J. Williams, W. J. Mulder, Z. A. Fayad and E. A. Fisher, HDL as a contrast agent for medical imaging, Clin. Lipidol., 2009, 4(4), 493–500 CrossRef CAS PubMed.
- W. Chen, P. A. Jarzyna, G. A. van Tilborg, V. A. Nguyen, D. P. Cormode, A. Klink, A. W. Griffioen, G. J. Randolph, E. A. Fisher, W. J. Mulder and Z. A. Fayad, RGD peptide functionalized and reconstituted high-density lipoprotein nanoparticles as a versatile and multimodal tumor targeting molecular imaging probe, FASEB J., 2010, 24(6), 1689–1699 CrossRef CAS PubMed.
- M. Schiener, M. Hossann, J. R. Viola, A. Ortega-Gomez, C. Weber, K. Lauber, L. H. Lindner and O. Soehnlein, Nanomedicine-based strategies for treatment of atherosclerosis, Trends Mol. Med., 2014, 20(5), 271–281 CrossRef CAS PubMed.
- C. S. Thaxton, J. S. Rink, P. C. Naha and D. P. Cormode, Lipoproteins and lipoprotein mimetics for imaging and drug delivery, Adv. Drug Delivery Rev., 2016, 106(Pt A), 116–131 CrossRef CAS PubMed.
- L. Foit and C. S. Thaxton, Synthetic high-density lipoprotein-like nanoparticles potently inhibit cell signaling and production of inflammatory mediators induced by lipopolysaccharide binding Toll-like receptor 4, Biomaterials, 2016, 100, 67–75 CrossRef CAS PubMed.
- S. Yang, M. G. Damiano, H. Zhang, S. Tripathy, A. J. Luthi, J. S. Rink, A. V. Ugolkov, A. T. Singh, S. S. Dave, L. I. Gordon and C. S. Thaxton, Biomimetic, synthetic HDL nanostructures for lymphoma, Proc. Natl. Acad. Sci. U. S. A., 2013, 110(7), 2511–2516 CrossRef CAS PubMed.
- M. P. Plebanek, R. K. Mutharasan, O. Volpert, A. Matov, J. C. Gatlin and C. S. Thaxton, Nanoparticle Targeting and Cholesterol Flux Through Scavenger Receptor Type B-1 Inhibits Cellular Exosome Uptake, Sci. Rep., 2015, 5, 15724 CrossRef CAS PubMed.
- A. J. Luthi, N. N. Lyssenko, D. Quach, K. M. McMahon, J. S. Millar, K. C. Vickers, D. J. Rader, M. C. Phillips, C. A. Mirkin and C. S. Thaxton, Robust passive and active efflux of cellular cholesterol to a designer functional mimic of high density lipoprotein, J. Lipid Res., 2015, 56(5), 972–985 CrossRef CAS PubMed.
- K. M. McMahon, L. Foit, N. L. Angeloni, F. J. Giles, L. I. Gordon and C. S. Thaxton, Synthetic high-density lipoprotein-like nanoparticles as cancer therapy, Cancer Treat. Res., 2015, 166, 129–150 CAS.
- S. Marrache and S. Dhar, Biodegradable synthetic high-density lipoprotein nanoparticles for atherosclerosis, Proc. Natl. Acad. Sci. U. S. A., 2013, 110(23), 9445–9450 CrossRef CAS PubMed.
- Z. Zhang, J. Chen, L. Ding, H. Jin, J. F. Lovell, I. R. Corbin, W. Cao, P. C. Lo, M. Yang, M. S. Tsao, Q. Luo and G. Zheng, HDL-mimicking peptide-lipid nanoparticles with improved tumor targeting, Small, 2010, 6(3), 430–437 CrossRef CAS PubMed.
- S. Marrache and S. Dhar, Engineering of blended nanoparticle platform for delivery of mitochondria-acting therapeutics, Proc. Natl. Acad. Sci. U. S. A., 2012, 109(40), 16288–16293 CrossRef CAS PubMed.
- S. Marrache, S. Tundup, D. A. Harn and S. Dhar, Ex vivo programming of dendritic cells by mitochondria-targeted nanoparticles to produce interferon-gamma for cancer immunotherapy, ACS Nano, 2013, 7392–7402 CrossRef CAS PubMed.
- B. J. Arsenault, I. Lemieux, J. P. Despres, P. Gagnon, N. J. Wareham, E. S. Stroes, J. J. Kastelein, K. T. Khaw and S. M. Boekholdt, HDL particle size and the risk of coronary heart disease in apparently healthy men and women: the EPIC-Norfolk prospective population study, Atherosclerosis, 2009, 206(1), 276–281 CrossRef CAS PubMed.
- N. L. Jones, J. W. Reagan and M. C. Willingham, The pathogenesis of foam cell formation: modified LDL stimulates uptake of co-incubated LDL via macropinocytosis, Arterioscler., Thromb., Vasc. Biol., 2000, 20(3), 773–781 CrossRef CAS.
- S. Xie, Y. F. Lee, E. Kim, L. M. Chen, J. Ni, L. Y. Fang, S. Liu, S. J. Lin, J. Abe, B. Berk, F. M. Ho and C. Chang, TR4 nuclear receptor functions as a fatty acid sensor to modulate CD36 expression and foam cell formation, Proc. Natl. Acad. Sci. U. S. A., 2009, 106(32), 13353–13358 CrossRef CAS PubMed.
- S. Xu, Y. Huang, Y. Xie, T. Lan, K. Le, J. Chen, S. Chen, S. Gao, X. Xu, X. Shen, H. Huang and P. Liu, Evaluation of foam cell formation in cultured macrophages: an improved method with Oil Red O staining and DiI-oxLDL uptake, Cytotechnology, 2010, 62(5), 473–481 CrossRef CAS PubMed.
- J. D. Toledo, L. V. Cabaleiro, H. A. Garda and M. C. Gonzalez, Effect of reconstituted discoidal high-density lipoproteins on lipid mobilization in RAW 264.7 and CHOK1 cells, J. Cell. Biochem., 2012, 113(4), 1208–1216 CrossRef CAS PubMed.
- C. J. Fielding and P. E. Fielding, Molecular physiology of reverse cholesterol transport, J. Lipid Res., 1995, 36(2), 211–228 CAS.
- A. V. Khera, M. Cuchel, M. de la Llera-Moya, A. Rodrigues, M. F. Burke, K. Jafri, B. C. French, J. A. Phillips, M. L. Mucksavage, R. L. Wilensky, E. R. Mohler, G. H. Rothblat and D. J. Rader, Cholesterol Efflux Capacity, High-Density Lipoprotein Function, and Atherosclerosis, N. Engl. J. Med., 2011, 364(2), 127–135 CrossRef CAS PubMed.
- A. R. Tall, Cholesterol efflux pathways and other potential mechanisms involved in the athero-protective effect of high density lipoproteins, J. Int. Med., 2008, 263(3), 256–273 CrossRef CAS PubMed.
- B. P. Atshaves, O. Starodub, A. McIntosh, A. Petrescu, J. B. Roths, A. B. Kier and F. Schroeder, Sterol Carrier Protein-2 Alters High Density Lipoprotein-mediated Cholesterol Efflux, J. Biol. Chem., 2000, 275(47), 36852–36861 CrossRef CAS PubMed.
- A. Frolov, A. Petrescu, B. P. Atshaves, P. T. C. So, E. Gratton, G. Serrero and F. Schroeder, High Density Lipoprotein-mediated Cholesterol Uptake and Targeting to Lipid Droplets in Intact L-cell Fibroblasts: A Single and Multiphoton Fluorescence Approach, J. Biol. Chem., 2000, 275(17), 12769–12780 CrossRef CAS PubMed.
- T.-S. Lee, C.-C. Pan, C.-C. Peng, Y. R. Kou, C.-Y. Chen, L.-C. Ching, T.-H. Tsai, S.-F. Chen, P.-C. Lyu and S.-K. Shyue, Anti-atherogenic effect of berberine on LXRα-ABCA1-dependent cholesterol efflux in macrophages, J. Cell. Biochem., 2010, 111(1), 104–110 CrossRef CAS PubMed.
- M. E. Weaver, G. A. Pantely, J. D. Bristow and H. D. Ladley, A quantitative study of the anatomy and distribution of coronary arteries in swine in comparison with other animals and man, Cardiovasc. Res., 1986, 20(12), 907–917 CrossRef CAS PubMed.
- A. M. Gown and M. C. Willingham, Improved detection of apoptotic cells in archival paraffin sections: immunohistochemistry using antibodies to cleaved caspase 3, J. Histochem. Cytochem., 2002, 50(4), 449–454 CrossRef CAS PubMed.
- A. Resendes, N. Majo, J. Segales, J. Espadamala, E. Mateu, F. Chianini, M. Nofrarıas and M. Domingo, Apoptosis in normal lymphoid organs from healthy normal, conventional pigs at different ages detected by TUNEL and cleaved caspase-3 immunohistochemistry in paraffin-embedded tissues, Vet. Immunol. Immunopathol., 2004, 99(3), 203–213 CrossRef CAS PubMed.
- I. Tabas, Consequences and therapeutic implications of macrophage apoptosis in atherosclerosis the importance of lesion stage and phagocytic efficiency, Arterioscler., Thromb., Vasc. Biol., 2005, 25(11), 2255–2264 CrossRef CAS PubMed.
Footnotes |
† Electronic supplementary information (ESI) available: Description of materials and methods, and additional data. See DOI: 10.1039/c7nr06295h |
‡ These authors contributed equally to this work. |
|
This journal is © The Royal Society of Chemistry 2018 |
Click here to see how this site uses Cookies. View our privacy policy here.