DOI:
10.1039/C7QM00337D
(Research Article)
Mater. Chem. Front., 2018,
2, 64-75
Photothermal effect: an important aspect for the enhancement of photocatalytic activity under illumination by NIR radiation†
Received
26th July 2017
, Accepted 4th October 2017
First published on 10th October 2017
Abstract
A key auxiliary role of the photothermal effect (PTE) in the enhancement of photocatalytic activity under illumination by near-infrared (NIR) radiation has been experimentally revealed. It was found that the photoexcitation of electrons, required for the process of photocatalysis, does not occur in the presence of NIR radiation alone without the support of both UV and visible radiation. Herein, a binary heterogeneous nanophotocatalyst, GR–ZnO, was fabricated by a novel approach using a hydrothermal process, in which ZnO nanotrapezoids were deposited over the surfaces of graphene nanosheets. In the unique approach reported, the reduction of graphene oxide to graphene has been accomplished using water by an entirely alternative mechanism compared to traditional reduction processes which require hazardous reducing agents. The produced GR–ZnO photocatalyst exhibited excellent performance in the rapid degradation of a perilous dye, methyl orange voluntarily used in industry. It has been found that the PTE is mainly responsible for the rapid degradation of methyl orange, which occurs under NIR laser irradiation. Furthermore, it has been established that the PTE could not emerge with NIR radiation alone, without the support of both UV and visible radiation. Upon considering its importance, the PTE of the GR–ZnO nanocatalyst has been revealed and compared with that of its individual components, viz., graphene and ZnO. Moreover, the photothermal conversion efficiency of the GR–ZnO nanocatalyst was evaluated. Overall, the excellent catalytic activity found in the GR–ZnO nanocatalyst was accounted for by its decreased band gap, which arose because the hybridization of ZnO with graphene could efficiently prevent the recombination of photo-generated charge carriers, resulting in enhanced catalytic activity of GR-ZnO nanocatalyst was attributed to improved optical absorption and enhancement in the adsorption affinity to methyl orange molecules.
Introduction
Recently nanostructured photocatalysts have received a great deal of attention owing to their excellent performance in the degradation of hazardous organic pollutants.1–5 Nanostructured photocatalysts have been proposed as better alternatives to conventional methods, viz. adsorption, biological degradation, chlorination, and ozonation used in the removal of toxic chemicals or dyes from industrial waste water.6 Among the nanostructured photocatalysts, agents developed on the basis of graphene (GR) are especially interesting due to their incredible properties and outstanding efficiency in the conversion of photo-energy into thermal energy.4,5 GR possesses a two-dimensional structure and consists of sp2-bonded carbon atoms linked in a hexagonal fashion. It has a specific arrangement that each carbon atom in the system is covalently bonded with three other carbon atoms. Moreover, GR has a high charge carrier mobility and specific surface area apart from its zero band gap, which effectively separates the photo-generated charge carriers and transfers them rapidly. In this manner, GR is an ideal platform to improve the quantum efficiency of semiconductor based photocatalysts. Hence, the coupling of GR with a semiconducting material could not only trap and shuttle the photo-generated electrons, but also increase the absorbing ability of pollutants and light radiation over the surface of the photocatalysts. In this manner, a series of GR based semiconductor photocatalysts have been designed and tested for their performace in photocatalytic activity.4,7–10 Several semiconductors were employed in conjugation with GR for the advancement of photocatalysts.1–5,7–10 Compared to those semiconductors, ZnO is principally interesting owing to its excellent optical activity, good stability and high mobility of electrons. In addition, ZnO has a large exciton binding energy of 60 meV at room temperature, and is low cost and nontoxic.11 The electron mobility of ZnO is nearly two orders of magnitude higher than that of the popularly known photocatalyst TiO2.4 In general, ZnO is a promising candidate for the promotion of robust photocatalysts. However the use of ZnO is obstructed by the major drawback of its large band gap of about 3.37 eV, and it cannot be excited in the presence of radiation having a wavelength higher than 400 nm. In addition, the recombination rate of charge carriers in ZnO is predominant and rapid as it transpires within nanoseconds. Therefore to extend the absorption threshold of ZnO, the coupling of a secondary material like GR is a favorable approach. The interesting possibility at this juncture is that ZnO conjugated with GR could lead to narrowing of the band gap in ZnO and also extend the absorption ability of near-infrared (NIR) radiation.
Meanwhile, the NIR region is considered as a biological window as living cells and tissues have low light scattering and adsorption of NIR radiation. The advantage of NIR radiation is the production of localized heat named the photothermal effect (PTE), which is readily employed in hyperthermia treatment to kill cancer cells due to its minimal invasiveness and higher effectiveness.12–16 Instead, the PTE could play a crucial role and significantly influence the enhanced activity of a photocatalyst. To date, the importance of PTE in photocatalytic activity has been completely ignored. So addressing the role of PTE in photocatalytic activity is a current urgent need. It has been identified that GR based nanocomposites are strong PTE agents under exposure to NIR radiation.17–19 During photocatalysis, illumination of GR nanocomposites to NIR radiation could generate a PTE even under the control of a catalytic mixture at room temperature by either flowing water or some other methods, which may improve the mobility of photo-generated charge carriers. This process causes prevention of the recombination of charge carriers and leads to enhanced activity of a photocatalyst. Therefore, on account of the significance of the PTE, herein we revealed the detailed mechanism for rapid photodegradation found for methyl orange (MO) over the surface of a ZnO-coupled GR (GR–ZnO) nanocatalyst. MO is an industrially versatile N-containing hazardous dye, which yields potentially carcinogenic aromatic amines under its natural reductive anaerobic degradation process. So the complete removal of such a toxic dye persisting in waste water is critically needed, and it has been successfully accomplished using a GR–ZnO nanocatalyst. Besides, the degradation of MO in the presence of NIR radiation was compared with the rate found for UV and visible irradiation. Overall, this study is dedicated to investigate the role of the PTE in photocatalysis.
Experimental methods
Materials
All the reagents were purchased from Aldrich and used without any purification unless otherwise noted, and aqueous solutions were prepared using ultrapure water obtained from a Milli-Q Plus system (Millipore).
Preparation of GO
Graphene oxide (GO) was prepared from graphite powder according to the Hummers and Offeman method with slight modifications.20 In a typical procedure, 1 g of graphite powder (<20 μm, Aldrich) was added to 40 mL of concentrated H2SO4 and stirred for 1 h under ice-cooling conditions. Then 15 mL of fuming HNO3 was slowly added and the mixture was stirred for 30 min. To this, 5 g of KMnO4 was gradually added with constant stirring and cooling. The resulting mixture was then stirred at room temperature for 12 h followed by the addition of 150 mL of DI water. After 30 min of stirring, 30 mL of H2O2 (30%) was slowly added, and immediately the color of the reaction mixture turned to bright yellow. This reaction mixture was centrifuged and washed with 1
:
10 HCl solution in order to remove metal ions. Then the mixture was washed with DI water until the acid was removed. The obtained dark-yellow colored GO was dried under vacuum at low temperature (40 °C) in order to avoid deoxygenation.
Preparation of GR–ZnO nanocatalysts
20 mg of GO was dispersed in 25 mL of ethanol by sonication for 5 min, followed by the addition of 20 mL of aqueous solution of zinc acetate (1 mmol). The resulting suspension was stirred under ambient conditions for 15 min, and subsequently an aqueous solution containing 8 mmol of NaOH was added. Furthermore, the contents of the mixture were stirred at room temperature for 15 min and transferred to a Teflon-lined stainless steel autoclave. The autoclave was then subjected to heating at 140 °C for 8 h, and the formed GR–ZnO nanocatalyst was separated by centrifugation. The GR–ZnO nanocatalyst was further purified by successive washing with ethanol and DI water and dried under vacuum.
Photocatalytic activity
The photocatalytic activity of the GR–ZnO nanocatalyst was evaluated by the degradation efficiency of MO under exposure to UV light (UVIR, 90 W), visible light (Philips, 40 and 90 W) and NIR laser irradiation (Armlaser Inc. USA, 2 W cm−2, 980 nm). In each experiment, 10 mg of the catalyst was suspended in 100 mL of aqueous solution of MO (10 mg L−1), and the suspension was magnetically stirred in the dark for 30 min in order to establish adsorption/desorption equilibrium of MO molecules on the surface of the catalyst. Then the pH of the catalytic mixture containing MO and GR–ZnO nanocatalysts measured prior to exposure was found to be 8.1. Subsequently, the mixture was transferred to a double-walled photocatalytic reactor with a water circulation system to maintain the reaction mixture at room temperature. Then the suspension was exposed to UV, visible and NIR irradiation individually. At a given interval of time, 5 mL of the suspension was taken out and centrifuged and the concentration of MO was analyzed by measuring the absorbance at 462 nm using a UV-vis spectrophotometer. Furthermore, the normalized concentration of MO after photocatalysis was calculated to be C/C0, where C0 is the initial concentration of MO and C is the concentration of MO measured after being exposed for a particular interval of time.
To explore the PTE of the GR–ZnO nanocatalyst under exposure to a NIR laser and to identify the possibility of PTE in the presence of UV and visible light, the photocatalytic experiments were performed without external circulation of water. Then the elevation in temperature of the catalytic mixture was measured using a Hanna precision digital thermometer (Model: HI93510).
Control experiments
For control experiments, ZnO was prepared in accordance with the procedure depicted above for the GR–ZnO nanocatalyst without the use of GO and GR was obtained by the reduction of GO using the procedure explained in ref. 21. Then the photodegradation of MO in the presence of ZnO and GR was evaluated and compared with that for the GR–ZnO nanocatalyst.
Photothermal effect
The PTE of the samples was evaluated using a 980 nm NIR diode laser system (Armlaser Inc. USA) with an output power of 2 W cm−2. In each experiment, 1 mL of an aqueous dispersion of the sample was transferred into a 1 × 1 × 4 cm3 cuvette and illuminated with a NIR laser. Then the increase in temperature of the suspension mediated by exposure to laser radiation was measured using a digital thermometer by immersing its thermocouple in the reaction mixture during the experiment.
Characterization
ATR-IR spectra were recorded using a Smiths ChemID diamond attenuated total reflection (DATR) spectrometer and TGA was conducted on a PerkinElmer Diamond TG/DTA instrument at a heating rate of 10 °C min−1. Powder XRD patterns were recorded on a Scintag X-ray diffractometer (PAD X), equipped with a Cu Kα photon source (45 kV, 40 mA) at a scanning rate of 3° min−1. SEM measurements were carried out using a JEOL JXA-8900 microscope and Raman spectra were recorded on a Renishaw R-3000QE system in the backscattering configuration using an argon ion laser with a wavelength of 785 nm. The UV-vis-NIR absorption spectra were recorded using a Jasco V-770 spectrophotometer and the pH of the catalytic mixture was measured using a Sargent-Welch (pH 8001) pH meter. The BET surface area was measured by N2 adsorption–desorption isotherms using an ASAP 2020 (Micromeretics, USA) surface area analyzer at 77 K.
Results and discussion
The FTIR spectra of GO, the GR–ZnO nanocatalyst and ZnO are shown in Fig. 1. The spectrum of GO (Fig. 1a) displayed the characteristic absorption bands corresponding to various oxygen containing functional groups, viz. O–H stretching of water at 3395 cm−1, C–O stretching of COOH groups at 1719 cm−1, epoxy symmetrical ring C–O deformation vibrations or phenolic C–O–H stretching at 1161 cm−1, and alkoxy C–O stretching at 1039 cm−1.21–23 The representative absorption band at 1619 cm−1 is attributed to aromatic C–C vibrations mixed with –OH of water and the band at 1619 cm−1 is due to carboxy C–O. However, the absorption bands related to oxygen containing functional groups, viz. C–O stretching, phenolic C–O–H stretching, alkoxy C–O stretching and carboxy C–O recorded for GO, disappeared in the spectrum of the GR–ZnO nanocatalyst (Fig. 1b). In addition, a new band was displayed at 1505 cm−1 due to skeletal vibrations of the GR nanosheets.24 The band generated by the vibrations of Zn–O was observed at 551 cm−1. The spectrum of ZnO (Fig. 1c) displayed bands at 3360 and 1405 cm−1 corresponding to –OH stretching and C–OH vibration, respectively. The band that appeared at 530 cm−1 is attributed to the stretching vibration of Zn–O.
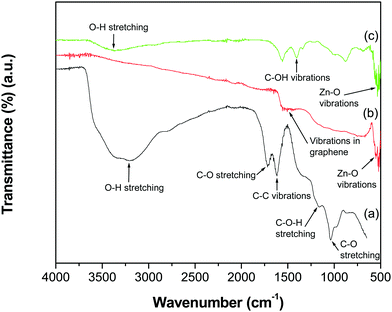 |
| Fig. 1 ATR-IR spectra of (a) GO, (b) the GR–ZnO nanocatalyst and (c) ZnO. | |
Fig. 2 shows the UV-vis-NIR absorption spectra of GO, ZnO and the GR–ZnO nanocatalyst. In the spectrum of GO (Fig. 2a), the characteristic absorption band exhibited at 229 nm and a shoulder around 300 nm could be assigned to the π → π* transition of aromatic C–C bonds and the n → π* transitions of C
O bonds, respectively.23–25 The absorption band at 363 nm in Fig. 2b could be due to the intrinsic band gap absorption of ZnO by the electron transition from the valence band to the conduction band (O2p → Zn3d).26 The GR–ZnO nanocatalyst (Fig. 2c) revealed a shoulder around 215 nm and a weak absorption at 280 nm (these bands are distinctly identifiable in Fig. S1, ESI†). These correspond to the π → π* transition of GR and are consistent with the absorption observed for aqueous stable graphene.27 This observation signifies that after reduction of GO, the electronic conjugation within GR nanosheets has successfully been restored. Moreover, the absorption band related to ZnO was also displayed at 369 nm. The red shift observed in the characteristic band of ZnO in the GR–ZnO nanocatalyst is ascribed to the strong interaction that exists between the GR nanosheets and ZnO. It was also observed that the absorbance of the band related to ZnO in the spectrum of the GR–ZnO nanocatalyst (Fig. 2c) increased compared to the absorbance of pristine ZnO (Fig. 2b), which could be due to the presence of GR and its high absorption ability. It is interesting to note that GO, ZnO and the GR–ZnO nanocatalyst possess strong absorbance in the NIR region also. Hence the conjugation of GR with ZnO has increased the photo-absorption capability of the GR–ZnO nanocatalyst, and this is a primary assessment of whether the GR–ZnO nanocomposite can be employed as an NIR-active photocatalyst.
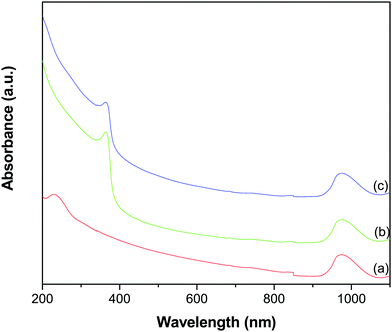 |
| Fig. 2 UV-vis-NIR absorption spectra of (a) GO, (b) ZnO and (c) the GR–ZnO nanocatalyst. | |
The XRD patterns shown in Fig. 3 depict the crystal phase and purity of GO, ZnO and the GR–ZnO nanocatalyst. The GO (Fig. 3a) displayed a reflection at a 2θ value of 9.9°, corresponding to its (002) crystalline plane with an interlayer d-spacing of 0.89 nm. In addition, this characteristic peak indicates complete oxidation of graphite and formation of GO. Furthermore, a low intensity peak appearing at 42.5° in GO corresponds to the (100) reflection of GR.23 The peaks that appeared for ZnO (Fig. 3b) at 31.8, 34.5, 36.2, 47.5, 56.6, 62.8, 66.4, 67.9, 69.1, 72.6 and 76.9° are indexed to the (100), (002), (101), (102), (110), (103), (200), (112), (201), (004) and (202) crystal planes of the hexagonal wurtzite structure of ZnO, respectively (JCPDS No. 36-1451). Meanwhile, the diffraction pattern of the GR–ZnO nanocatalyst (Fig. 3c) is identical to the pattern of ZnO, while an additional two low intensity peaks at 23.7 and 43.5° corresponding to the (002) and (100) planes of GR, respectively, appeared.22,28 The disappearance of the characteristic peak of GO in Fig. 3c suggests the successful reduction of GO to GR. However, the peaks corresponding to GR are weakly resolved in the GR–ZnO nanocatalyst due to effective exfoliation of the GR nanosheets. However, the magnified display shown in Fig. S2 (ESI†) provides clarification of the presence of the characteristic peaks corresponding to GR in the GR–ZnO nanocatalyst. Furthermore, the existence of the reflections corresponding to the hexagonal wurtzite structure of ZnO illustrates that conjugation of GR with ZnO could not alter the orientation and structure of ZnO in the GR–ZnO nanocatalyst. Moreover, the occurrence of sharp and intense diffraction peaks in Fig. 3b and c reveals the high crystalline nature of ZnO.
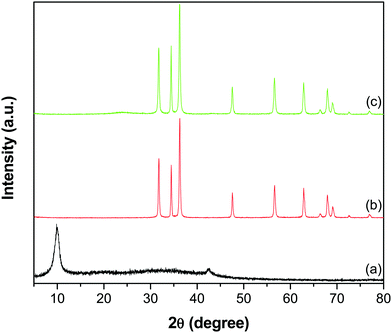 |
| Fig. 3 XRD patterns of (a) GO, (b) ZnO and (c) the GR–ZnO nanocatalyst. | |
The FESEM images of GO shown in Fig. 4a and b depict the effective exfoliation of GO in the form of thin nanosheets and their porous structures generated by the opening of planar carbon networks wedged at the edge surface of the crystallites due to oxidation. It is also evident that GO possesses a two-dimensional structure having a wrinkled surface with single to few thin nanosheets. Fig. 4c–f show the formation of homogeneous tetragonal trapezoid shaped ZnO nanocrystals and their surface is smooth and uniform. The well-faceted side surfaces and perfect tetragonal end planes of the as-grown ZnO nanotrapezoids (NTs) indicate that the synthesized ZnO NTs are single crystalline. Furthermore, the images of the GR–ZnO nanocatalyst (Fig. 4g–i) reveal anchoring of systematically arranged uniform ZnO NTs over the surface of the GR nanosheets. In the process of encapsulation of ZnO NTs over the GR nanosheets, the GR nanosheets behave as templates and prevent the aggregation of the ZnO NTs. The calculated value of the mean diameter of the ZnO NTs existing in the GR–ZnO nanocatalyst was found to be around 30 nm, and this matches with the value calculated using the Debye–Scherrer equation.29 In Fig. 4g and i, the GR nanosheets are distinctly definable and these nanosheets have a typical crumpled sheet-like morphology carrying single to few thin sheets. Adequate anchoring of ZnO NTs over the surface of the GR nanosheets is ascribed to the wrinkled structure of the GR nanosheets. The comparison of Fig. 4c–i demonstrates that the layered structure of GO is destroyed in the GR–ZnO nanocatalyst due to strong adherence of ZnO NTs over the surface of the GR nanosheets. Hence, assessment of Fig. 4c–i illustrates that the morphology of the pristine ZnO NTs is retained in the GR–ZnO nanocatalyst also. The interfacial structure formed by the hybridization of ZnO NTs and GR nanosheets provides the strong interaction between them and results in close contact between the ZnO NTs and GR nanosheets. Owing to the close contact between GR and ZnO, electrons can easily be transported from the ZnO NTs to the GR nanosheets, leading to the separation of photo-generated charge carriers. Hence it enhances the photocatalytic activity of the GR–ZnO nanocatalyst in addition to absorption of NIR light. Moreover, elemental analysis data analyzed from EDS indicate the presence of C, Zn and O elements in the GR–ZnO nanocatalyst (Fig. S3, ESI†); among these, the peaks for Zn and O originated from the ZnO NTs and C was generated from the GR nanosheets. In addition, the content of oxygen is comparatively lower in the GR–ZnO nanocatalyst, which reveals reduction of GO to GR by the successful removal of oxygen-containing functional groups in the presence of water. Then the BET surface area measured for the GR–ZnO nanocatalyst from the N2 adsorption–desorption isotherm was found to be 34.1 m2 g−1.
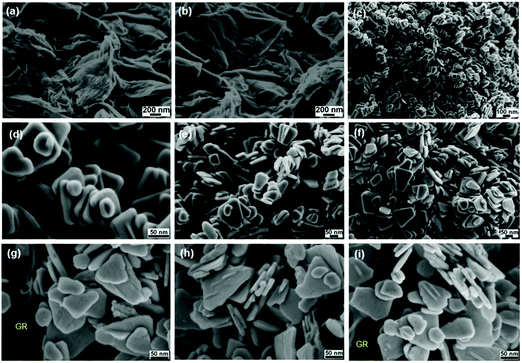 |
| Fig. 4 FESEM images of (a and b) graphene oxide, (c–f) ZnO nanotrapezoids and (g–i) the GR–ZnO nanocatalyst. | |
Furthermore, the reduction of GO to GR and the subsequent deposition of ZnO NTs over the GR framework were examined by Raman analysis. The Raman spectra of GO and the GR–ZnO nanocatalyst (Fig. 5) displayed the characteristic D and G bands. The spectrum of GO (Fig. 5a) exhibited the D band at 1328 cm−1 and the G band at 1598 cm−1, while the GR–ZnO nanocatalyst (Fig. 5b) showed the D and G bands at 1304 and 1588 cm−1, respectively. The D band displayed in the spectrum of the GR–ZnO nanocatalyst is ascribed to the breathing mode of the k-point phonons of A1g symmetry and the G band is attributed to the first-order scattering of the E1g phonon mode of sp2 carbon atoms.30 Compared to GO, blue shifting of the D and G bands occurred in the GR–ZnO nanocatalyst, which indicates the successful reduction of GO to GR. Furthermore, the value of ID/IG calculated for the GR–ZnO nanocatalyst (1.31) was found to be higher than that of GO (1.14), which signifies the increase in the number of smaller sp2 domains in the GR–ZnO nanocatalyst.31 This suggests that in the GR–ZnO nanocatalyst most of the oxygenated groups are removed, and the vacant lattice sites produced via carbon atom removal in the form of CO or CO2 during graphite oxidation remain unchanged by the reduction process and predominantly define the intact graphene regions.31
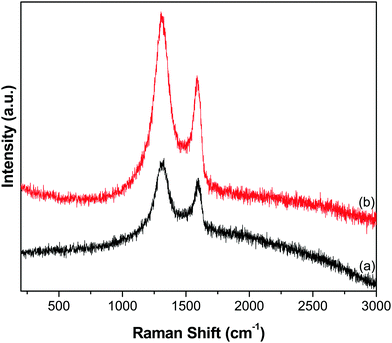 |
| Fig. 5 Raman spectra of (a) GO and (b) the GR–ZnO nanocatalyst. | |
To quantify the contents of the GR–ZnO nanocatalyst, TGA was performed and the thermograms obtained for GO, ZnO and the GR–ZnO nanocatalyst are illustrated in Fig. 6. The profile of GO (Fig. 6a) displayed two weight loss steps. The initial weight loss observed below 150 °C was identified by the removal of water molecules adsorbed on the surface of GO. Whereas the successive significant weight loss that occurred around 180 °C was ascribed to the decomposition of labile oxygen functional groups and the pyrolysis of the carbon skeleton. The ZnO (Fig. 6b) possessed better thermal stability, while the minor weight loss in ZnO around 200 °C is attributed to the decomposition and dehydration of surface hydroxyls.32 The GR–ZnO nanocatalyst (Fig. 6c) showed considerably less weight loss compared to GO due to the presence of a minute number of decomposable functional groups, which suggests effective conversion of GO to GR by the action of deoxygenation during the hydrothermal process. The weight loss that appeared below 100 °C for the GR–ZnO nanocatalyst (Fig. 6b) was attributed to the loss of absorbed water, and the subsequent weight loss below 400 °C was attributed to the decomposition of residual organic functional groups persisting in GR.33,34 The subsequent weight loss that occurred in the GR–ZnO nanocatalyst between 400 and 500 °C was assigned to the oxidation of GR.
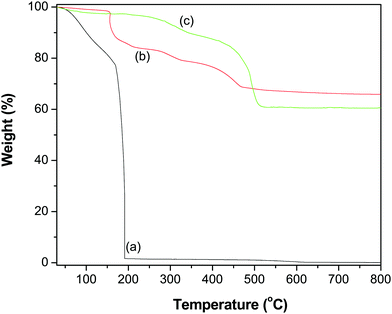 |
| Fig. 6 TGA profiles of (a) GO, (b) ZnO and (c) the GR–ZnO nanocatalyst. | |
Here in the process of synthesis it was found that the initial light yellow colored suspension of GO and zinc acetate turned to a dark color after the hydrothermal process, which indicated the reduction of GO to GR without any supplemental reducing agent. Compared to the traditional reduction process which employs hazardous hydrazine or borohydride, herein the reduction of GO to GR occurred with a completely different reduction mechanism. The physiochemical properties of water used as a solvent in this hydrothermal process can change with an increase in its temperature and pressure; due to this, the supercritical water becomes a fluid having special features such as strong electrolytic solvent power, high diffusion coefficient, and ion molecules.35,36 Moreover, water at high temperatures can promote a favorable environment for the cleavage of heterolytic bonds of the functional groups such as –OH, C
O and C–O–C persisting over the surface of GO. Therefore, the reduction of GO to GR that occurred in this process without any external reducing agent could be related to the deoxygenation or dehydration of bonds of functional groups existing over GO.
In consideration of its enhanced absorption in the NIR region, the catalytic activity of the GR–ZnO nanocatalyst was assessed in the presence of a NIR laser by the photodegradation rate of MO. Fig. 7 shows the absorption spectra of the aqueous solution of MO in the presence of the GR–ZnO nanocatalyst at different intervals of illumination by NIR radiation. It is obvious that the characteristic band of MO located around 462 nm has completely disappeared, which suggests the structural rupture of MO molecules and their subsequent degradation by the action of the GR–ZnO nanocatalyst. The photodegradation of MO with the elapse of time was quantitatively estimated by sequential variation in its concentration after exposure to NIR laser irradiation. Fig. 8 reveals the photodegradation profile of MO in the presence of GR, ZnO and the GR–ZnO nanocatalyst. In the absence of any catalyst or NIR radiation, the degradation rate of MO was insignificant, which indicates that the self-photosensitization of MO was very low. With the GR–ZnO nanocatalyst, MO was completely degraded after 40 min, while complete degradation was not attained with either GR or ZnO within 40 min of exposure. Hence the synergetic combination of GR and ZnO has significantly enhanced the photocatalytic activity of the GR–ZnO nanocatalyst.
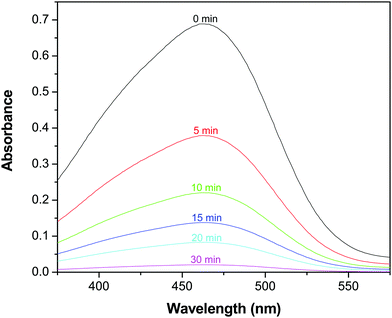 |
| Fig. 7 Absorption spectra of an aqueous solution of methyl orange in the presence of the GR–ZnO nanocatalyst at different intervals of illumination by a NIR laser. | |
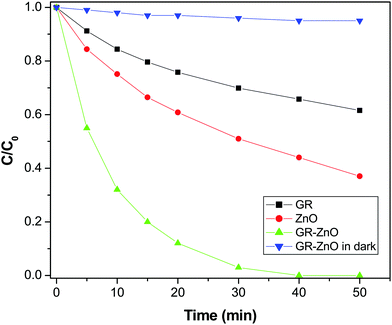 |
| Fig. 8 Photocatalytic degradation profile of methyl orange over the surface of GR, ZnO and the GR–ZnO nanocatalyst under exposure to a NIR laser and the GR–ZnO nanocatalyst in the dark. | |
Furthermore, the degradation of MO in the presence of NIR laser irradiation was compared with the degradation rate measured in visible radiation (Fig. 9). It was found that MO was completely degraded at 70 min in the presence of visible light, which is significantly lower than the degradation rate found with the NIR laser. To find the influence of power or the intensity of the light source, the photodegradation of MO was executed using a different intensity visible lamp of 40 W instead of a 90 W lamp used in the prior experiment. It was found that the degradation rate of MO was not majorly affected by varying the power of the source, which elucidate that the degradation of MO is not solely dependent on the power of the light source (Fig. S4, ESI†). For comparison, the photodegradation of MO was assessed under illumination by UV radiation also and it was observed that MO was entirely degraded in 60 min of exposure. Interestingly, photodegradation of MO was very high in NIR radiation compared to both UV and visible radiation. The excellence of the GR–ZnO nanocatalyst under exposure to a NIR laser could be related to PTE, which could be generated during the process of photocatalysis. It is known that NIR radiation is mainly responsible for the PTE.37 Therefore, to explore the influence of the PTE which could play a decisive role in the endowed degradation of MO, this study has been further extended to emphasize the PTE. The GR–ZnO nanocatalyst is composed of GR nanosheets, and it is identified that GR is a promising candidate for PTE in the presence of NIR radiation.18 Under this influence, we measured the PTE or rise in temperature of the catalytic mixture comprising the GR–ZnO nanocatalyst and MO solution identical to prior photocatalytic experiments without external circulation of water employed to prevent heating-up of the catalytic suspension (Fig. S5, ESI†). To elucidate the PTE in detail, the rise in temperature of pure water, MO solution alone, and a suspension containing a mixture of GR and MO solution was measured under illumination by a NIR laser for 40 min. Overall, an increase of 4.6 °C was perceived for the suspension containing a mixture of the GR–ZnO nanocatalyst and MO solution. This was higher than the values measured for pure water, MO solution and the suspension containing a mixture of GR and MO solution (Fig. S5, ESI†). Moreover to reveal any possibility of a PTE under exposure to either UV or visible radiation, we measured the change in temperature of the samples by exposing to UV and visible light distinctly, and found a minute elevation in both cases (Fig. S6, ESI†).
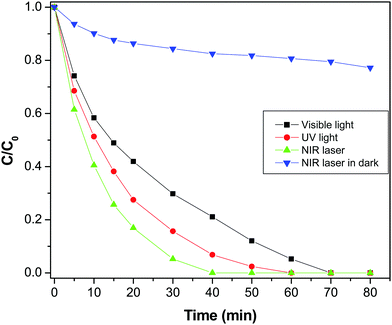 |
| Fig. 9 Photodegradation profile of methyl orange in the presence of the GR–ZnO nanocatalyst under exposure to UV light, visible light and NIR laser irradiation. | |
Fascinatingly, the degradation of MO under exposure to NIR radiation in the dark was significantly lower than the rate measured in NIR radiation in addition to the lamps present in the laboratory (Fig. 9). So, it is obvious that NIR radiation plays a decisive role in the enhanced photocatalytic activity of the GR–ZnO nanocatalyst. It seems that the degradation of MO could not occur with NIR radiation alone in the dark, instead it needs supplement of both UV and visible radiation. In general, NIR radiation requires the support of both UV and visible radiation to enhance the activity of a photocatalyst. Based on this important evidence, it could be assessed that the excitation of electrons could not ensue with NIR radiation alone without coupling of UV and visible radiation.2
Since NIR radiation is unable to accelerate either ZnO or MO, almost no electrons could be generated, and MO could not degrade in the dark. However, in combination with UV and visible radiation, the heat generated by NIR light could remarkably speed up the process of degradation. So NIR radiation plays an important auxiliary role in enhancing the photocatalytic activity of the GR–ZnO nanocatalyst, since the NIR light alone cannot result in the photodegradation of MO. The key role played by the PTE in the enhanced photodegradation of MO could be explained as the cause of photocatalysis is the separation and transfer of photo-generated charge carriers. Herein, the PTE leads to an increase in temperature and facilitates the mobility of charge carriers in the GR–ZnO nanocatalyst, which results to the rapid photodegradation of MO. In detail, illumination of the suspension containing the GR–ZnO nanocatalyst with a NIR laser heats-up the GR sheets due to the PTE and accelerates the photo-generated electrons. This causes rapid degradation of MO molecules over the surface of the GR–ZnO nanocatalyst.
The degradation of MO was performed with constant circulation of water, and even under this condition the PTE could influence photodegradation, so we attempted to quantify the effect of the PTE. Hot carrier relaxation that occured in the GR nanosheets could be the reason for the rise in temperature found in the presence of NIR laser illumination.38 The relaxation of photo-generated carriers usually consists of several processes, viz. carrier–carrier interaction, carrier–phonon scattering (mainly optical phonon emission), Auger recombination, and carrier trapping.38,39 It was reported by Ruzicka et al. that the relaxation of a hot carrier in GR nanosheets is independent of temperature,38 and the carriers have a hot distribution immediately after photo-excitation. Subsequently, energy relaxation is dominated by carrier–phonon scattering, and a large number of optical phonons are emitted and give rise to a significant deviation of the phonon distribution in the excitation spot away from the equilibrium distribution dictated by the temperature of the sample. As the photothermal process is a collective electronic effect, the lattice temperature rapidly reaches equilibrium. Therefore, the hot carriers experience a much higher lattice temperature than the temperature of other parts of the sample, and the finite temperature variation has no influence on the carrier dynamics.2 This might lead to the PTE and increase the local temperature of the photocatalytic mixture upon illumination by a NIR laser even under the condition of constant circulation of water. Hence the PTE caused a significant degradation of MO in the presence of the NIR laser compared to the degradation rate in UV and visible light.
Owing to the importance of the PTE in photocatalysis, the PTE of the GR–ZnO nanocatalyst was quantified under exposure to a 980 nm laser and compared with that of its individual components, viz. GR and ZnO. Fig. 10 illustrates the profile of time-dependent temperature increase in the samples in response to illumination of the 980 nm laser at their concentration level of 2 mg mL−1. After 7 min of irradiation, an elevation of 47.3 °C was found for an aqueous dispersion of the GR–ZnO nanocatalyst. Under identical conditions, the temperature of GR was increased by 43.7 °C and it was 39.2 °C for ZnO. Meanwhile, the increase in temperature found for water was 8.3 °C. Since the ability of water to absorb NIR radiation is very low, it caused a minute PTE. Hence, the contribution of water in the enhanced PTE of the GR–ZnO nanocatalyst is extremely low. Comparatively it can be perceptible that the PTE of the GR–ZnO nanocatalyst is higher than that of GR and ZnO, and this demonstrates that the unique structure generated by coupling GR with ZnO NTs has radically improved the absorption ability of NIR radiation and made the GR–ZnO nanocomposites a robust photocatalyst. In addition, the PTE of the GR–ZnO nanocatalyst measured in five consecutive cycles was virtually constant, so no photobleaching was found in the GR–ZnO nanocatalyst (Fig. 11). It was also observed that the PTE of the GR–ZnO nanocatalyst was linearly dependent on its concentration level (Fig. S7, ESI†).
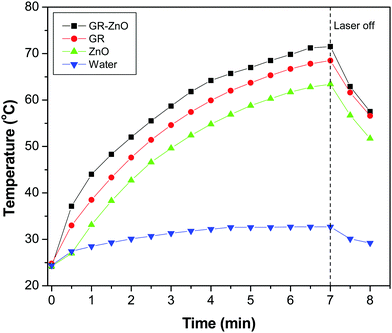 |
| Fig. 10 Temperature increase in aqueous dispersion measured as a function of time under exposure to a 980 nm laser. | |
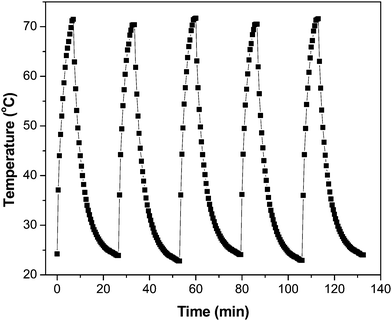 |
| Fig. 11 Temperature variation measured for the aqueous dispersion of the GR–ZnO nanocatalyst (2 mg mL−1) for five cycles under illumination by a NIR laser. | |
Subsequently, the photothermal conversion efficiency of the GR–ZnO nanocatalyst was evaluated using the equation proposed by Roper with some modifications. For this purpose, the variation in temperature of the aqueous dispersion of the GR–ZnO nanocatalyst (2 mg mL−1) was measured as a function of time under exposure to the laser for 7 min, and at this juncture, the temperature increase reached a steady condition. Then shut-off of the laser leads to a drop in temperature, and this was monitored to find the rate of heat transfer from the aqueous dispersion of the GR–ZnO nanocatalyst to the surrounding environment using eqn (1):
| 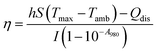 | (1) |
where
η is the photothermal efficiency,
h is the heat transfer coefficient and
S is the surface area of the sample container.
Tmax is the maximum temperature attained by the system of aqueous dispersions and
Tamb is the ambient surrounding temperature in °C. However,
I is the power of the laser source,
A980 is the absorbance of aqueous dispersions of the GR–ZnO nanocatalyst measured at 980 nm and
Qdis is the rate of heat dissipated due to absorption of light by the solvent and the container. The values of all these parameters are provided in
Table 1.
Table 1 Values used in the calculation of photothermal efficiency (η) of the GR–ZnO nanocatalyst
T
max (°C) |
T
amb (°C) |
I (mW) |
A
980
|
m
D (g) |
C
D (J g−1 °C) |
τ
s (s) |
hS (mW °C−1) |
Q
Dis (mW) |
η (%) |
71.5 |
24.2 |
2000 |
1.195 |
1.01 |
4.179 |
219.5 |
19.8 |
25.9 |
48.6 |
To find the value of hS, a dimensionless driving force of temperature, θ, is introduced and scaled using the maximum system temperature, Tmax, and the ambient surrounding temperature, Tamb:
|  | (2) |
and the time constant of the sample system,
τs, was evaluated using
eqn (3) | t = −τs ln(θ) | (3) |
Then the value of
τs was calculated as shown in Fig. S8 and S9 (ESI
†), and using its value the unknown parameter,
hS, was evaluated with the help of eqn (4).
|  | (4) |
where
mD and
CD are the mass and heat capacity of water, respectively. The value of
Qdis was measured separately using a quartz cuvette containing only DI water without any sample. Hence, the photothermal conversion efficiency (
η) estimated for the GR–ZnO nanocatalyst was found to be 48.6%.
The overall degradation of MO employing the GR–ZnO nanocatalyst under exposure to UV, visible light and NIR laser irradiation could be ascribed to its high surface area, which enhances the adsorption of MO molecules due to the π–π interaction between the aromatic ring of the MO molecules and GR nanosheets, which leads to the noncovalent adsorption of dye molecules.40 An additional crucial factor that could contribute to the significant photocatalytic activity of the GR–ZnO nanocatalyst was narrowing of its band gap, which extends the optical or light absorption of the GR–ZnO nanocatalyst and results in an efficient transfer of photo-generated electrons from ZnO to the GR nanosheets.41 Therefore, the bandgap measured for ZnO and the GR–ZnO nanocatalyst was found to be 3.37 and 3.35 eV, respectively. The band gap in ZnO is reduced by its conjugation with GR nanosheets. Moreover, the GR in the GR–ZnO nanocatalyst behaves as a source for trapping and shuttling of photo-generated electrons. The mechanism underlying the photocatalytic activity of the GR–ZnO nanocatalyst in the degradation of MO under exposure to a NIR laser is schematically demonstrated in Fig. 12 and also narrated with the possible mechanism in UV and visible radiation (Fig. S10, ESI†). The valence band (VB) and the conduction band (CB) in ZnO are located at −7.39 and −4.19 eV (vs. vacuum), respectively42 and the work function of GR is −4.42 eV (vs. vacuum).43 Exposure of an aqueous solution of the GR–ZnO nanocatalyst and MO to light produces pairs of electrons (e−) and holes (h+), and ZnO promotes the electrons from the VB to the CB by leaving the holes in the VB. This is followed by efficient interfacial transfer of electrons from the CB of ZnO to the GR nanosheets as the Fermi level of GR is lower than the CB minimum of ZnO. This process remarkably reduces the recombination probability of photo-excited electrons and holes in ZnO. Therefore, a number of photo-excited holes were retained to a large extent, which participate in the oxidation of MO and enhance the photocatalytic activity. Simultaneously, more photo-generated holes can react with adsorbed water to form a hydroxyl radical (OH˙), which can promote the decomposition of MO. In addition, the oxygen adsorbed on the surface of ZnO can accept electrons and form superoxide radical anions (O2˙−), which also causes the formation of OH˙ upon protonation. These hydroxyl radicals and superoxide radical anions are responsible for the decomposition of MO into non-toxic products under simulated light irradiation.44 From these predictions, possible degradation reactions can be summarized as follows:
H2O2 + O2˙− → −OH + ˙OH + O2 |
MO + ˙OH → H2O + CO2 + Non toxic products |
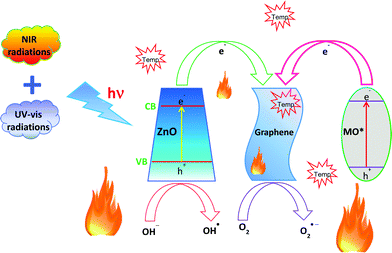 |
| Fig. 12 The proposed mechanism for the photocatalytic degradation of methyl orange in the presence of the GR–ZnO nanocatalyst upon illumination by a NIR laser. | |
For a better quantification of photocatalytic activity of the GR–ZnO nanocatalyst, a kinetic analysis was used. It was assumed that the photocatalytic degradation of MO in the presence of catalysts follows pseudo-first-order kinetics, which could be described in terms of the Langmuir–Hinshelwood (L–H) model:1,45,46
| 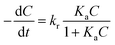 | (5) |
where (−d
C/d
t) and
C are the degradation rate and the concentration of MO, respectively,
t is the reaction or exposure time,
kr is the rate constant of the reaction, and
Ka is the adsorption coefficient of the reactant. The initial concentration of MO used in the photocatalytic experiments was very low (
C0 = 10 mg L
−1), so that the
KaC term becomes negligible and
eqn (5) can be described with first-order kinetics. Then modifying
eqn (5) under the initial conditions of the photocatalytic procedure,
viz.,
t = 0,
C =
C0 gives:
where
C0 is the initial concentration of MO,
kapp is the apparent rate constant of the reaction and
t is the time of exposure to light.
The L–H plots obtained on the basis of eqn (6) for the photodegradation of MO under exposure to a NIR laser have provided a linear relationship with concentration and exposure time (Fig. S11, ESI†). The linearity of the L–H plots reveals that the photodegradation of MO follows pseudo-first-order reaction kinetics. Furthermore, the apparent rate constants (kapp) calculated for GO, ZnO and the GR–ZnO nanocatalyst using the L–H plots are summarized in Table 2. It was found that the value of kapp for the GR–ZnO nanocatalyst (0.1128 min−1) was about twelve times higher than that for GR (0.0092 min−1) and six times higher than that for ZnO (0.0188 min−1). Hence the perfect blending of GR with ZnO has brought tremendous improvement in the activity of the GR–ZnO nanocatalyst. Moreover, the L–H plots acquired for UV, visible, NIR and NIR in the dark were also found to be linear (Fig. S12, ESI†). The value of kapp calculated for UV light was 0.0693 min−1 (Table 3), which was higher than the value of visible light (0.0446 min−1). Whereas the value of kapp assessed for the NIR laser in the presence of laboratory lights was significantly higher (0.1128 min−1) than the value obtained under exposure to the NIR laser in the dark (0.0038 min−1).
Table 2 Rate constants (kapp) calculated by the Langmuir–Hinshelwood (L–H) plots for the photodegradation of methyl orange under exposure to the NIR laser
|
k
app (min−1) |
GR |
0.0092 |
ZnO |
0.0188 |
GR–ZnO nanocomposite |
0.1128 |
Table 3 Rate constants calculated for the photodegradation of methyl orange over the surface of the GR–ZnO nanocatalyst under exposure to different light sources
k
app (min−1) |
UV |
Visible (90 W) |
NIR |
NIR in the dark |
0.0693 |
0.0446 |
0.1128 |
0.0038 |
For practical implementation of a catalyst, its recycling and stability are crucial. So after its application in the degradation of MO, the GR–ZnO nanocatalyst was recycled by centrifugation and employed in the following four successive cycles. It was found that after its usage in five consecutive cycles, the efficiency of the GR–ZnO nanocatalyst was not reduced significantly. In addition, the XRD patterns of the GR–ZnO nanocatalyst (Fig. S13, ESI†) acquired after its employment in five consecutive cycles could not show any prominent alteration. This elucidates the stability of the GR–ZnO nanocatalyst, and no structural rupture or detachment between the ZnO NTs and GR nanosheets occurs.
Conclusions
In summary, a promising heterogeneous GR–ZnO nanocatalyst was prepared by the in situ reduction of GO to GR and the successive nucleation of ZnO NTs through a facile hydrothermal process. In this approach, water has reduced GO to GR by promoting a favorable environment for the cleavage of heterolytic bonds of the functional groups such as –OH, C
O and C–O–C persisting over the surface of GO. The GR–ZnO nanocatalyst has been revealed to be an excellent photocatalyst for the complete degradation of hazardous MO. The enhanced photocatalytic activity of the GR–ZnO nanocatalyst can be attributed to an increase in optical absorption, a decrease in the band gap, and an effective separation of photo-generated charge carriers. The heterostructured GR–ZnO nanocatalyst could be easily recycled without any significant loss in its activity, and hence the GR–ZnO nanocatalyst is an excellent anti-photocorrosive catalyst. Overall, PTE has played an important crucial role in the significantly improved photocatalytic activity of the GR–ZnO nanocatalyst under illumination by a NIR laser. Furthermore, the photo-excitation process of electrons could not occur alone under exposure to NIR radiation without the support of both UV and visible radiation.
Conflicts of interest
There are no conflicts to declare.
Acknowledgements
The authors acknowledge the support from the NIH-NIGMS grant #1SC3GM086245 and the department grant L0002 from the Welch Foundation, Texas, USA.
References
- P. Dou, F. Tan, W. Wang, A. Sarreshteh, X. Qiao, X. Qiu and J. Chen, One-step Microwave-Assisted Synthesis of Ag/ZnO/Graphene Nanocomposites with Enhanced Photocatalytic Activity, J. Photochem. Photobiol., A, 2015, 302, 17–22 CrossRef CAS.
- Z. Gan, X. Wu, M. Meng, X. Zhu, L. Yang and P. K. Chu, Photothermal Contribution to Enhanced Photocatalytic Performance of Graphene-Based Nanocomposites, ACS Nano, 2014, 8, 9304–9310 CrossRef CAS PubMed.
- H. Yang, P. Wang, D. Wang, Y. Zhu, K. Xie, X. Zhao, J. Yang and X. Wang, New Understanding on Photocatalytic Mechanism of Nitrogen-Doped Graphene Quantum Dots-Decorated BiVO4 Nanojunction Photocatalysts, ACS Omega, 2017, 2, 3766–3773 CrossRef CAS.
- Y. Bu, Z. Chen, W. Li and B. Hou, Highly Efficient Photocatalytic Performance of Graphene–ZnO Quasi-Shell–Core Composite Material, ACS Appl. Mater. Interfaces, 2013, 5, 12361–12368 CAS.
- J. Wang, T. Tsuzuki, B. Tang, X. Hou, L. Sun and X. Wang, Reduced Graphene Oxide/ZnO Composite: Reusable Adsorbent for Pollutant Management, ACS Appl. Mater. Interfaces, 2012, 4, 3084–3090 CAS.
- M. R. Hoffmann, S. T. Martin, W. Choi and D. W. Bahnemann, Environmental Applications of Semiconductor Photocatalysis, Chem. Rev., 1995, 95, 69–96 CrossRef CAS.
- C. Han, Z. Chen, N. Zhang, J. C. Colmenares and Y. Xu, Hierarchically CdS Decorated 1D ZnO Nanorods-2D Graphene Hybrids: Low Temperature Synthesis and Enhanced Photocatalytic Performance, Adv. Funct. Mater., 2015, 25, 221–229 CrossRef CAS.
- H. Zhang, L. Guo, D. Wang, L. Zhao and B. Wan, Light-Induced Efficient Molecular Oxygen Activation on a Cu(II)-Grafted TiO2/Graphene Photocatalyst for Phenol Degradation, ACS Appl. Mater. Interfaces, 2015, 7, 1816–1823 CAS.
- M. Latorre-Sanchez, I. Esteve-Adell, A. Primo and H. Garcia, Innovative Preparation of MoS2–Graphene Heterostructures Based on Alginate Containing (NH4)2MoS4 and their Photocatalytic Activity for H2 Generation, Carbon, 2015, 81, 587–596 CrossRef CAS.
- W. Wang, Y. Li, Z. Kang, F. Wang and J. C. Yu, NIR-driven photocatalyst based on α-NaYF4:Yb,Tm@TiO2 core–shell structure supported on reduced graphene oxide, Appl. Catal., B, 2016, 182, 184–192 CrossRef CAS.
- M. H. Huang, S. Mao, H. Feick, H. Yan, Y. Wu, H. Kind, E. Webber, R. Russo and P. Yang, Room-Temperature Ultraviolet Nanowire Nanolasers, Science, 2001, 292, 1897–1899 CrossRef CAS PubMed.
- J. Liu, X. Zheng, L. Yan, L. Zhou, G. Tian, W. Yin, L. Wang, Y. Liu, Z. Hu, Z. Gu, C. Chen and Y. Zhao, Bismuth Sulfide Nanorods as a Precision Nanomedicine for in Vivo Multimodal Imaging-Guided Photothermal Therapy of Tumor, ACS Nano, 2015, 9, 696–707 CrossRef CAS PubMed.
- H. J. Lee, W. Hong, S. Jeon, Y. Choi and Y. Cho, Electroactive Polypyrrole Nanowire Arrays: Synergistic Effect of Cancer Treatment by On-Demand Drug Release and Photothermal Therapy, Langmuir, 2015, 31, 4264–4269 CrossRef CAS PubMed.
- P. Zhang, H. Huang, J. Huang, H. Chen, J. Wang, K. Qiu, D. Zhao, L. Ji and H. Chao, Noncovalent Ruthenium(II) Complexes-Single-Walled Carbon Nanotube Composites for Bimodal Photothermal and Photodynamic Therapy with Near-Infrared Irradiation, ACS Appl. Mater. Interfaces, 2015, 7, 23278–23290 CAS.
- Y. Li, Y. Deng, X. Tian, H. Ke, M. Guo, A. Zhu, T. Yang, Z. Guo, Z. Ge, X. Yang and H. Chen, Multipronged Design of Light-Triggered Nanoparticles To Overcome Cisplatin Resistance for Efficient Ablation of Resistant Tumor, ACS Nano, 2015, 9, 9626–9637 CrossRef CAS PubMed.
- X. Yao, Z. Tian, J. Liu, Y. Zhu and N. Hanagata, Mesoporous Silica Nanoparticles Capped with Graphene Quantum Dots for Potential Chemo–Photothermal Synergistic Cancer Therapy, Langmuir, 2017, 33, 591–599 CrossRef CAS PubMed.
- G. Gollavelli and Y. Ling, Magnetic and Fluorescent Graphene for Dual Modal Imaging and Single Light Induced Photothermal and Photodynamic Therapy of Cancer Cells, Biomaterials, 2014, 35, 4499–4507 CrossRef CAS PubMed.
- K. Yang, S. Zhang, G. Zhang, X. Sun, S. Lee and Z. Liu, Graphene in Mice: Ultrahigh In Vivo Tumor Uptake and Efficient Photothermal Therapy, Nano Lett., 2010, 10, 3318–3323 CrossRef CAS PubMed.
- T. Yeh, C. Teng, L. Chen, S. Chen and H. Teng, Graphene Oxide-based Nanomaterials for Efficient Photoenergy Conversion, J. Mater. Chem. A, 2016, 4, 2014–2048 CAS.
- W. S. Hummers and R. E. Offeman, Preparation of Graphitic Oxide, J. Am. Chem. Soc., 1958, 8, 1339 CrossRef.
- G. M. Neelgund, A. Oki and Z. Luo, In situ Deposition of Hydroxyapatite on Graphene Nanosheets, Mater. Res. Bull., 2013, 48, 175–179 CrossRef CAS PubMed.
- G. M. Neelgund, A. Oki and Z. Luo, ZnO and Cobalt Phthalocyanine Hybridized Graphene: Efficient Photocatalysts for Degradation of Rhodamine B, J. Colloid Interface Sci., 2014, 43, 257–264 CrossRef PubMed.
- Q. Li, B. D. Guo, J. G. Yu, J. R. Ran, B. H. Zhang, H. J. Yan and J. R. Gong, Highly Efficient Visible-Light-Driven Photocatalytic Hydrogen Production of CdS-Cluster-Decorated Graphene Nanosheets, J. Am. Chem. Soc., 2011, 133, 10878–10884 CrossRef CAS PubMed.
- J. I. Paredes, S. Villar-Rodil, A. Martinez-Alonso and J. M. D. Tascon, Graphene Oxide Dispersions in Organic Solvents, Langmuir, 2008, 24, 10560–10564 CrossRef CAS PubMed.
- L. Li, K. Liu, G. Yang, C. Wang, J. Zhang and J. Zhu, Fabrication of Graphene-Quantum Dots Composites for Sensitive Electrogenerated Chemiluminescence Immunosensing, Adv. Funct. Mater., 2011, 21, 869–878 CrossRef CAS.
- M. Azarang, A. Shuhaimi, R. A. M. Golsheikh and M. Sookhakian, Synthesis and Characterization of ZnO NTs/Reduced Graphene Oxide Nanocomposite Prepared in Gelatin Medium as Highly Efficient Photo-degradation of MB, Ceram. Int., 2014, 40, 10217–10221 CrossRef CAS.
- D. Li, M. B. Mueller, S. Gilje, R. B. Kaner and G. G. Wallace, Processable Aqueous Dispersions of Graphene Nanosheets, Nat. Nanotechnol., 2008, 3, 101–105 CrossRef CAS PubMed.
- W. Ong, S. Voon, L. Tan, B. T. Goh, S. Yong and S. Chai, Enhanced Daylight-Induced Photocatalytic Activity of Solvent Exfoliated Graphene (SEG/ZnO) Hybrid Nanocomposites toward Degradation of Reactive Black 5, Ind. Eng. Chem. Res., 2014, 53, 17333–17344 CrossRef CAS.
-
B. D. Cullity, Elements of X-Ray Diffraction, Edison-Wesley Publishing Company Inc., 1978 Search PubMed.
- G. Malekshoar, K. Pal, Q. He, A. Yu and A. K. Ray, Enhanced Solar Photocatalytic Degradation of Phenol with Coupled Graphene-Based Titanium Dioxide and Zinc Oxide, Ind. Eng. Chem. Res., 2014, 53, 18824–18832 CrossRef CAS.
- C. Gomez-Navarro, R. T. Weitz, A. M. Bittner, S. Matteo, A. Mews, M. Burghard and K. Kern, Electronic Transport Properties of Individual Chemically Reduced Graphene Oxide Sheets, Nano Lett., 2007, 7, 3499–3503 CrossRef CAS PubMed.
- K. Morishige, S. Kittaka, T. Moriyasu and T. Morimoto, Thermal Desorption Study of Surface Hydroxyls on ZnO, J. Chem. Soc., Faraday Trans. 1, 1980, 76, 738–745 RSC.
- C. Xu, X. Wang, J. W. Zhu, X. J. Yang and L. Lu, Deposition of Co3O4 Nanoparticles onto Exfoliated Graphite Oxide Sheets, J. Mater. Chem., 2008, 18, 5625–5629 RSC.
- S. Stankovich, D. Dikin, R. D. Piner, K. A. Kohlhaas, A. Kleinhammes, Y. Jia, Y. Wu, T. SNguyen and R. S. Ruoff, Synthesis of Gaphene-Based Nanosheets via Chemical Reduction of Exfoliated Graphite Oxide, Carbon, 2007, 45, 1558–1565 CrossRef CAS.
- P. Wang, J. Wang, X. F. Wang, H. G. Yu, J. G. Yu, M. Lei and Y. G. Wang, One-step Synthesis of Easy-recycling TiO2–rGO Nanocomposite Photocatalysts with Enhanced Photocatalytic Activity, Appl. Catal., B, 2013, 132–133, 452–459 CrossRef CAS.
- Q. G. Luo, X. J. Jiang, M. J. Li, Q. Shen, L. M. Zhang and H. G. Yu, Facile Fabrication and Enhanced Photocatalytic Performance of Ag/AgCl/rGO Heterostructure Photocatalyst, ACS Appl. Mater. Interfaces, 2013, 5, 2161–2168 Search PubMed.
- L. Yang, L. Y. Tseng, G. Suo, L. Chen, J. Yu, W. Chiu, C. Huang and C. Lin, Photothermal Therapeutic Response of Cancer Cells to Aptamer-Gold Nanoparticle-Hybridized Graphene Oxide under NIR Illumination, ACS Appl. Mater. Interfaces, 2015, 7, 5097–5106 CAS.
- B. A. Ruzicka, L. K. Werake, H. Zhao, S. Wang and K. P. Loh, Femtosecond Pump-Probe Studies of Reduced Graphene Oxide Thin Films, Appl. Phys. Lett., 2010, 96, 173106/1 CrossRef CAS.
- S. Kaniyankandy, S. N. Achary, S. Rawalekar and H. N. Ghosh, Ultrafast Relaxation Dynamics in Graphene Oxide: Evidence of Electron Trapping, J. Phys. Chem. C, 2011, 115, 19110–19116 CAS.
- H. Zhang, X. J. Lv, Y. M. Li, Y. Wang and J. H. Li, P25-Graphene Composite as a High Performance Photocatalyst, ACS Nano, 2010, 4, 380–386 CrossRef CAS PubMed.
- T. Lv, L. Pan, X. Liu and Z. Sun, Enhanced Photocatalytic Degradation of Methylene blue by ZnO-Reduced Graphene Oxide–Carbon Nanotube Composites Synthesized via Microwave-Assisted Reaction, Catal. Sci. Technol., 2012, 2, 2297–2301 CAS.
- L. J. Tzeng, C. L. Cheng and Y. F. Chen, Enhancement of Band-edge Emission Induced by Defect Transition in the Composite of ZnO Nanorods and CdSe/ZnS Quantum Dots, Opt. Lett., 2008, 33, 69–571 CrossRef.
- N. Yang, J. Zhai, D. Wang, Y. Chen and L. Jiang, Two-Dimensional Graphene Bridges Enhanced Photoinduced Charge Transport in Dye-Sensitized Solar Cells, ACS Nano, 2010, 4, 887–894 CrossRef CAS PubMed.
- T. J. Liu, Q. Wang and P. Jiang, Morphology-Dependent Photo-catalysis of Bare Zinc Oxide Nanocrystals, RSC Adv., 2013, 3, 12662–12670 RSC.
- G. M. Neelgund and A. Oki, Photocatalytic Activity of CdS and Ag2S Quantum dots Deposited on Poly(amidoamine) Functionalized Carbon Nanotubes, Appl. Catal., B, 2011, 110, 99–107 CrossRef CAS PubMed.
- G. M. Neelgund, V. N. Bliznyuk and A. Oki, Photocatalytic Activity and NIR Laser Response of Polyaniline Conjugated Graphene Nanocomposite Prepared by a Novel Acid-less Method, Appl. Catal., B, 2016, 187, 357–366 CrossRef CAS PubMed.
Footnote |
† Electronic supplementary information (ESI) available. See DOI: 10.1039/c7qm00337d |
|
This journal is © the Partner Organisations 2018 |
Click here to see how this site uses Cookies. View our privacy policy here.