DOI:
10.1039/C7QM00571G
(Research Article)
Mater. Chem. Front., 2018,
2, 768-774
Facile preparation of Prussian blue/polypyrrole hybrid nanofibers as robust peroxidase mimics for colorimetric detection of L-cysteine
Received
9th December 2017
, Accepted 30th January 2018
First published on 30th January 2018
Abstract
A facile and sensitive detection of L-cysteine is essential for biosensors, environmental monitoring and medical diagnosis. In this study, a simple approach has been demonstrated to prepare Prussian blue (PB)/polypyrrole (PPy) hybrid nanofibers as efficient peroxidase-like mimics for the determination of L-cysteine. α-Fe2O3 nanofibers act as both templates and a source of Fe3+ to polymerize pyrrole in an acidic environment, resulting in the formation of PB/PPy hybrid nanofibers. The as-prepared PB/PPy hybrid nanofibers show a superior catalytic activity as peroxidase mimics over α-Fe2O3 nanofibers. Due to the high catalytic efficiency of the resultant PB/PPy hybrid nanofibers, we have developed a simple colorimetric approach for the rapid and sensitive determination of L-cysteine with a detection limit of about 16 nM over a wide linear range from 1 to 20 μM. In addition, the detection system displays a high selectivity toward L-cysteine over other amino acids, glucose, and common ions. This work provides a new strategy for the preparation of peroxidase-like nanomaterials with improved catalytic activity, which shows great promise for application in highly sensitive detection.
Introduction
The determination of L-cysteine is very important because it plays a crucial role in the metabolism of the human body.1–3 Generally, a large variety of diseases including coronary heart disease, cerebrovascular disease and peripheral vascular disease are strongly related to the abnormal concentration of L-cysteine in vivo.1–3 In the past few decades, diverse detection routes such as high performance liquid chromatography (HPLC), fluorescence spectroscopy, electrochemical methods, etc., have been developed for the determination of L-cysteine.4–11 However, these approaches face insurmountable drawbacks of the requirement of expensive instruments and time consuming and complicated sample preparation processes. Recently, a simple colorimetric method for the detection of L-cysteine has attracted more and more attention due to the advantages of fast response, high sensitivity, and excellent selectivity.12–15 These colorimetric routes are based on diverse kinds of enzyme-like catalytic reactions. For example, FeCo nanoparticles have been incorporated into carbon nanofibers as efficient peroxidase mimics, which have been used for the sensitive detection of L-cysteine with a detection limit of 0.15 μM.12
For the determination of L-cysteine using an enzyme-like reaction system, one of the most important issues is to fabricate high performance nanocatalysts. Natural enzymes have been proven to be the most efficient biological catalysts with an extraordinarily high catalytic activity and specificity, but there are some unavoidable major problems including the sharp decrease of catalytic efficiency under harsh environmental conditions, instability for operation, and high costs for synthesis and purification.16–18 Recently, nanomaterial based enzyme mimics have attracted much attention due to their tunable catalytic efficiency, easy preparation procedure, and low cost.19–22 Up to now, a large variety of nanomaterials including noble metal nanoparticles,23 metal oxide nanobelts,24 chalcogenide nanomaterials,25 carbon nanomaterials,26 and metal–organic frameworks (MOFs)27 have been reported to exhibit enzyme-like activities. In order to enhance the catalytic activities of enzyme mimics, hybrid nanomaterials with a synergistic effect have been widely studied. For example, we have demonstrated a facile one-pot hydrothermal approach to prepare CuS/graphene composites, which showed an enhanced peroxidase-like activity over individual CuS nanomaterials and graphene alone.28 A large number of other hybrid nanomaterials such as PtPd/Fe3O4 nanoparticles,29 reduced graphene oxide (rGO)/Cu2O/Ag nanosheets,30 Au/PANI nanorices,31 Ag@carbon dot hybrids,32 and Co3O4/CeO2/PEDOT nanofibers33 have also been prepared to show improved catalytic activities compared with individual components. These hybrid nanomaterial based enzyme-like catalytic reaction systems have promising applications in biosensing and environmental monitoring. However, it is still a great challenge to synthesize new hybrid nanomaterials with a controllable composition and unique chemical structure to show a superior enzyme-like catalytic activity for the detection of specific substances with a high sensitivity.
In this study, we have demonstrated a facile approach for the preparation of Prussian blue (PB)/polypyrrole (PPy) hybrid nanofibers using α-Fe2O3 nanofibers as both templates and a source of Fe3+ to polymerize pyrrole in an acidic environment. In the past few years, PB/PPy hybrid nanomaterials have shown excellent electrocatalytic activity toward the reduction of H2O2.34,35 Herein, the resultant PB/PPy hybrid nanofibers have been proved to be efficient peroxidase mimics, showing an improved catalytic activity compared to α-Fe2O3 nanofibers. The apparent kinetic experiment demonstrated their superior affinity to peroxidase substrates over HRP. Owing to the high catalytic efficiency of the prepared PB/PPy hybrid nanofibers, a colorimetric route for assaying L-cysteine with a high sensitivity and excellent selectivity has been developed. The terrific catalytic efficiency of the prepared PB/PPy hybrid nanofibers as peroxidase mimics shows bright prospects in biotechnology, medicine, environmental science and other fields.
Experimental
Chemicals and materials
L-Cysteine and polyvinylpyrrolidone (PVP, Mw = 1
300
000 g mol−1) were purchased from Sigma-Aldrich. Fe(NO3)3·9H2O was commercially obtained from Tianjin East China Reagent Factory. 3,3′,5,5′-Tetramethylbenzidine (TMB) was bought from Sinopharm Chemical Reagent Beijing Co., Ltd. K3Fe(CN)6 was purchased from Tianjin Gwangfu Fine Chemical Industry Research Institute. Pyrrole was obtained from Aladdin. Dimethyl sulfoxide (DMSO) was bought from Aladdin. Isopropanol and N,N-dimethylformamide (DMF) were acquired from Tianjin Tiantai Fine Chemical Co., Ltd. H2O2 (30%), hydrochloric acid (36–38%) and ethanol were purchased from Beijing Chemical Works. All the chemicals in our experiments were used as received without further purification.
Preparation of α-Fe2O3 nanofibers via an electrospinning technique
α-Fe2O3 nanofibers were prepared via electrospinning followed by a heat treatment process.36 In a typical procedure, 0.9 g of PVP and 0.9 g of Fe(NO3)3·9H2O were dissolved in a mixed solvent consisting of 5 mL of DMF and 5 mL of isopropanol. After vigorous stirring at room temperature for 12 h, a homogeneous solution was obtained. Then, the solution was loaded into a single glass capillary with the tip fixed about 30 cm away from an aluminum foil collector. A high-voltage power supply maintained at 18 kV was applied for the electrospinning process, and the fibrous PVP/Fe(NO3)3 membrane was obtained. Finally, the fibrous membrane was peeled off from the aluminum foil and calcined in air at 450 °C for 3 h; then α-Fe2O3 nanofibers were obtained.
Fabrication of PB/PPy hybrid nanofibers via a hydrothermal reaction
5 mg of the as-prepared α-Fe2O3 nanofibers was dispersed in 10 mL of distilled water under ultrasonication for a few minutes. Then 25 mg of K3Fe(CN)6 and 20 μL of the pyrrole monomer were added into the solution mentioned above, and the solution was under magnetic stirring at 0 °C for about 1 h. After that, 100 μL of hydrochloric acid was injected into the above mixture and stirred for another 0.5 h. The mixture solution was transferred into a 50 mL Teflon-lined stainless steel autoclave. The reaction was carried out in an electric oven at 150 °C for 4 h. After cooling down to room temperature, the final product was centrifuged and washed thoroughly with water and ethanol several times, and then dried in a vacuum oven.
Peroxidase-like catalytic activity of PB/PPy hybrid nanofibers
The peroxidase-like activity of the synthesized PB/PPy hybrid nanofibers was evaluated through a model catalytic reaction toward the oxidation of a peroxidase substrate of TMB. Typically, 20 μL of TMB solution (15 mM in DMSO) and 20 μL of H2O2 (30%) were injected into 3 mL of acetate buffer solution (pH = 4, unless otherwise stated). Subsequently, 20 μL of PB/PPy hybrid nanofiber suspension (3 mg mL−1) was added into the above solution. The catalytic process was monitored via a UV-vis spectrophotometer. For the detection of H2O2, different concentrations of H2O2 from 0 to 10 mM were substituted in the above-mentioned reaction.
Detection of L-cysteine
A simple colorimetric route was developed for the detection of L-cysteine. In a model assay, different concentrations of L-cysteine from 0 to 300 μM were added into the mixture containing 20 μL of TMB solution (15 mM in DMSO), 20 μL of H2O2 (30%) and 3 mL of acetate buffer solution (pH = 4.0). Next, 20 μL of PB/PPy hybrid nanofiber suspension (3 mg mL−1) was added to the above mixture. Then, these solutions were incubated at room temperature for 10 min. Catalytic measurements were conducted by monitoring the absorbance changes in spectrum mode after 10 min of the catalytic reaction in natural daylight.
Characterization
The morphologies of the resultant α-Fe2O3 and PB/PPy hybrid nanofibers were characterized using a scanning electron microscope (SEM, Nova NanoSEM 450) and a transmission electron microscope (TEM, JEOL JEM-1200 EX) operated at 15 and 100 kV, respectively. Typical high-resolution TEM (HRTEM) experiments and energy dispersive X-ray (EDX) spectroscopy and mapping were performed on a JEOL JEM-2100F and an FEI Tecnai G2 F20 electron microscope. The crystal phases of the products were determined using an X-ray diffractometer (Empyrean, PANalytical B.V.) based on Cu-Kα radiation. The chemical structures of the samples were recorded on a Raman spectrometer (LabRAM HR Evolution). To study the chemical states of the as-prepared PB/PPy hybrid nanofibers, X-ray photoelectron spectra (XPS) were measured on a Thermo Scientific ESCALAB250 instrument. UV-vis spectral measurements were performed on a Shimadzu 2501 PC spectrometer to study the peroxidase-like catalytic properties of the as-prepared PB/PPy hybrid nanofibers.
Results and discussion
Morphology and chemical structure of PB/PPy hybrid nanofibers
In the present work, α-Fe2O3 nanofibers were first prepared by electrospinning a mixture consisting of PVP, iron nitrate, isopropanol and DMF, followed by a calcination process at 450 °C in air. Fig. 1a shows a typical SEM image of the as-prepared α-Fe2O3 nanofibers, displaying a uniform and smooth morphology and most of the nanofibers have diameters in the f 90 to 260 nm range. The morphology of the α-Fe2O3 nanofibers was also characterized using a TEM image shown in Fig. 1b. It is found that the nanofibers exhibit a hollow structure and a wall thickness of about 10 to 40 nm. Then, we fabricated PB/PPy hybrid nanofibers using α-Fe2O3 nanofibers as both templates and a source of Fe3+ in acidic solution in the presence of pyrrole and K3Fe(CN)6via a facile hydrothermal process. During the reaction, Fe3+ released from α-Fe2O3 initiates the polymerization of pyrrole and produces Fe2+. Meanwhile, the generated Fe2+ will react with K3Fe(CN)6 to form PB, which are encapsulated in PPy matrices. Fig. 1c show a typical SEM image of the as-prepared PB/PPy hybrid nanofibers. It is found that the surfaces of the prepared PB/PPy hybrid nanofibers are much rougher than those of α-Fe2O3 nanofibers. The resultant PB/PPy hybrid nanofibers have diameters ranging from 120 to 330 nm, which are much larger than those of individual α-Fe2O3 nanofibers. In addition, a typical TEM image shows that the PB/PPy hybrid nanofibers exhibit a porous structure, which is attributed to the formation of PB crystal blocks and PPy via a sacrificial-template approach.
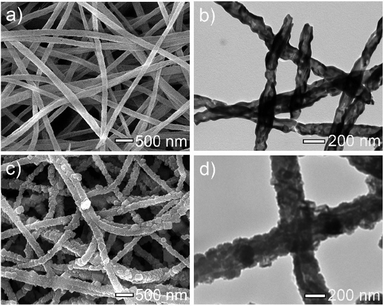 |
| Fig. 1 (a) SEM image and (b) TEM image of the prepared α-Fe2O3 nanofibers. (c) SEM image and (d) TEM image of the prepared PB/PPy hybrid nanofibers. | |
The formation of the as-prepared PB/PPy hybrid nanofibers is further characterized using a HRTEM image, which shows the PB crystal blocks coated with PPy (Fig. 2a and b). The typical HRTEM image of the PB/PPy hybrid nanofibers displays a lattice fringe spacing of about 0.31 nm, which is consistent with the (220) crystallographic plane of PB, suggesting the formation of PB.37Fig. 2c shows an EDX spectral image of the PB/PPy hybrid nanofibers, showing the presence of Fe, C and N elements. This result indicates the formation of PB/PPy. It should be pointed out that the signals of O, Cu and Si are attributed to the carbon-coated copper grid and the TEM instrument. Fig. 2e–g show the elemental mapping images of the Fe, C and N elements, showing that these elements are distributed on the nanofibers, demonstrating that the hybrid nanofibers consist of PB crystal blocks and PPy layers.
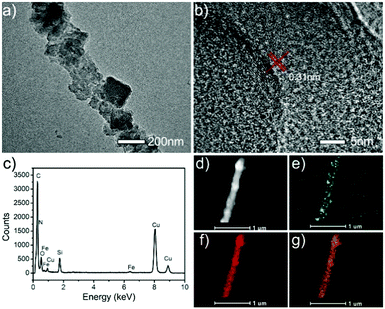 |
| Fig. 2 (a) TEM image, (b) HRTEM image, and (c) EDX spectrum of the prepared PB/PPy hybrid nanofibers. (d) HAADF-STEM pattern and (e–g) EDX elemental mapping images of Fe–L, C–K and N–K in PB/PPy hybrid nanofibers. | |
The crystallographic features of the as-prepared α-Fe2O3 and PB/PPy nanofibers were characterized by XRD measurements. In Fig. 3a, the XRD pattern of α-Fe2O3 nanofibers shows characteristic peaks at 2θ ≈ 24.2, 33.2, 35.7, 40.9, 49.5, 54.1, 57.5, 62.5, 64.2, and 72.0°, which can be ascribed to the (012), (104), (110), (113), (024), (116), (018), (214), (300), and (1010) planes of the crystallographic α-Fe2O3.38 In addition to these typical bands, there are no other obvious diffraction peaks, indicating the successful fabrication of α-Fe2O3 nanofibers. After the hydrothermal reaction, all of the peaks corresponding to the α-Fe2O3 nanofibers disappear and some typical peaks at 2θ ≈ 17.4, 24.8, 35.3, 39.6, 50.7, 54.0, and 57.2° emerge. All of these peaks can be ascribed to the (200), (220), (400), (420), (440), (600), and (620) planes of Fe4[Fe(CN)6]3, demonstrating that the α-Fe2O3 has been completely converted into PB.37 The formation of PB/PPy hybrid nanofibers was also characterized by Raman spectroscopy. As shown in Fig. 3b, in the spectrum of the α-Fe2O3 nanofibers, the peaks at 226.8 and 495.5 cm−1 are ascribed to the A1g mode, the peaks at 292.9, 408.1, and 609.6 cm−1 are attributed to the Eg modes, the peak at 660.8 cm−1 is related to the Eu mode, and the peak at 1320.7 cm−1 is ascribed to the second harmonic vibration of α-Fe2O3.38 However, all of these typical peaks disappear in the spectrum of PB/PPy hybrid nanofibers, while two new strong characteristic peaks at around 1326.1 and 1572.4 cm−1 are observed, which are attributed to the antisymmetric in-ring C–N stretching and C
C vibrations of PPy. In addition, the weak peak at 925.2 cm−1 is caused by ring deformation and the peak at 1039.3 cm−1 is ascribed to the symmetrical C–H in-plane bending and N–H in-plane deformation of PPy.39 On the other hand, a typical peak at 2157.0 cm−1 related to the stretching vibration of the C
N group of PB was also observed in the spectrum of PB/PPy hybrid nanofibers.40 These results indicate the successful preparation of PB/PPy hybrid nanofibers.
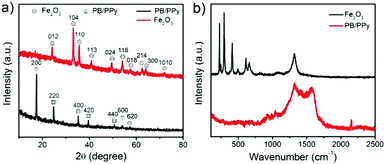 |
| Fig. 3 (a) XRD patterns of the prepared α-Fe2O3 nanofibers and PB/PPy hybrid nanofibers. (b) Raman spectra of the as-prepared α-Fe2O3 nanofibers and PB/PPy hybrid nanofibers. | |
Further information on the chemical composition and oxidation state of the prepared PB/PPy hybrid nanofibers was verified by XPS measurements. As shown in Fig. 4a, the full XPS spectrum displays the existence of C, N, O and Fe elements in the product. In detail, the high resolution XPS spectrum of Fe2p exhibits peaks at 712.0 and 724.0 eV, which can be attributed to the Fe2p3/2 and Fe2p1/2 of the Fe3+ in PB, respectively (Fig. 4b). Furthermore, the peak at 708.8 eV can be related to the Fe2p3/2 in [Fe(CN)6]4−.41 The high resolution XPS spectrum of C1s features four peaks. As shown in Fig. 4c, the peaks positioned at 284.2 and 284.9 eV are attributed to C
C and C–C groups, respectively. In addition, the peaks centered at 284.6 and 285.6 eV originate from the C–N of PPy and [Fe(CN)6]4−, respectively. In Fig. 4d, the high resolution XPS spectrum of N1s is fitted with three components. The peak at 397.9 eV is ascribed to the C–N of [Fe(CN)6]4−,41 while the peaks at 399.9 and 400.1 eV are related to the –NH– and positively charged nitrogen (N+) of PPy.38 In a word, the XPS results further verified the formation of PB/PPy hybrid nanofibers.
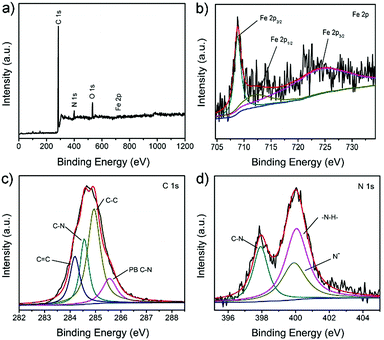 |
| Fig. 4 XPS spectra of the as-prepared PB/PPy hybrid nanofibers: (a) full survey spectrum, (b) Fe 2p, (c) C 1s, and (d) N 1s. | |
Peroxidase-like catalytic activity of PB/PPy hybrid nanofibers
It has been reported that PB nanoparticles show an excellent peroxidase-like activity;42,43 thus the as-prepared PB/PPy hybrid nanofibers are expected to be good peroxidase-like catalysts. To investigate the peroxidase-like activity of the product, we performed a model reaction toward the oxidation of TMB in the presence of H2O2. UV-vis absorption spectra were used to evaluate the catalytic process. As shown in Fig. 5a, the prepared PB/PPy hybrid nanofibers can quickly catalyze the oxidation of the colorimetric substrate TMB in the presence of H2O2, which generates a typical blue color in the reaction solution with a maximum absorption peak at 651 nm. In comparison, there are no obvious absorbance peaks in the absence of TMB, H2O2, or PB/PPy hybrid nanofibers. This result indicates that the prepared PB/PPy hybrid nanofibers are an efficient peroxidase-like nanocatalyst. In the following, we have compared the catalytic activity of the prepared PB/PPy hybrid nanofibers with that of individual α-Fe2O3 nanofibers. As shown in Fig. 5b, the prepared PB/PPy hybrid nanofibers show much better peroxidase-like catalytic activity than individual α-Fe2O3 nanofibers. The superior catalytic activity of the prepared PB/PPy hybrid nanofibers might be due to the unique chemical structure of PB and good conductivity of PPy. It is reported that the peroxidase-like catalytic activity is strongly related to pH values; thus we studied the catalytic activity of PB/PPy hybrid nanofibers under different pH values, and found that the catalytic activity at pH = 4.0 is much higher than those at other pH values (Fig. 5c and d). Therefore, an optimal pH value for this model reaction is chosen as 4.0.
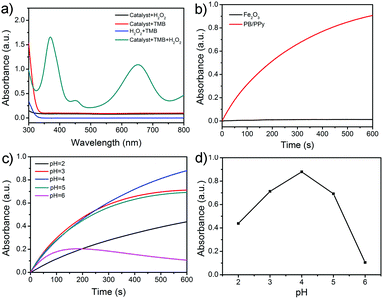 |
| Fig. 5 (a) UV-vis absorbance curves of the oxidation of TMB in different systems (H2O2 + PB/PPy hybrid nanofibers, TMB + PB/PPy hybrid nanofibers, TMB + H2O2, TMB + H2O2 + PB/PPy hybrid nanofibers) in acetate buffer solution (pH = 4.0) recorded at 600 s of the reaction. (b) Time-dependent absorption changes at 651 nm with addition of α-Fe2O3 nanofibers and PB/PPy hybrid nanofibers. (c) Relationship toward the peroxidase-like catalytic activity of the PB/PPy hybrid nanofibers against various pH values and (d) the relevant line chart. | |
In order to investigate the peroxidase-like catalytic mechanism of the prepared PB/PPy hybrid nanofibers, we performed the kinetic analysis by changing the concentrations of TMB or H2O2, while the other one is invariant. As shown in Fig. 6a–d, a series of steady-state reaction rates were calculated and applied to the Lineweaver–Burk double reciprocal plots. The data are fitted to the Michaelis–Menten equation:
where
ν is the initial velocity,
Km is the Michaelis–Menten constant,
Vmax is the maximum reaction velocity and [S] represents the concentration of the substrate. Generally, the
Km value represents the affinity of the catalyst towards the substrate. The lower the
Km value, the stronger the affinity between the catalyst and the substrate. The Michaelis constant (
Km) and the maximal reaction velocity (
Vmax) of the PB/PPy hybrid nanofibers compared to those of HRP and previously reported nanomaterial based artificial peroxidases are listed in
Table 1. It is obviously observed that the
Km values of the PB/PPy hybrid nanofibers with both H
2O
2 and TMB as the substrates are much lower than those of HRP and other nanomaterials, suggesting a higher affinity of PB/PPy hybrid nanofibers for H
2O
2 and TMB than HRP and other nanomaterials. This result demonstrates the efficient peroxidase-like activity of the prepared PB/PPy hybrid nanofibers.
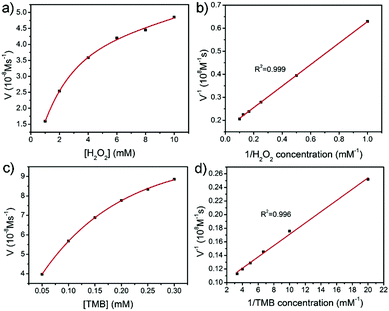 |
| Fig. 6 Steady-state kinetic experiments of PB/PPy hybrid nanofibers. The PB/PPy hybrid nanofibers suspension concentration was fixed at 20 μg mL−1 in 3 mL of acetate buffer solution (pH = 4). (a) H2O2 concentration was kept constant at 65 mM with varied concentrations of TMB; (b) the concentration of TMB was maintained at 0.1 mM and the H2O2 content was diverse; and (c and d) double-reciprocal plots of the catalyst under the condition of a fixed content of one substrate (H2O2 or TMB) with various concentrations of the other substrate. | |
Table 1 Comparison of the kinetic parameters of PB/PPy with HRP and different reported nanomaterials toward the substrates H2O2 (A) and TMB (B)
A |
Catalyst |
K
m [mM] |
V
max [10−8 M s−1] |
Substrate H2O2 |
PB/PPy |
2.88 |
6.18 |
HRP44 |
3.7 |
8.71 |
PB/γ-Fe2O3 MNPs45 |
323.6 |
117 |
APTES-Fe3O446 |
23.466 |
86.2 |
Co3O4 GNs47 |
245 |
28.5 |
B |
Catalyst |
K
m [mM] |
V
max [10−8 M s−1] |
Substrate TMB |
PB/PPy |
0.094 |
11.37 |
HRP44 |
0.434 |
10.0 |
PB/γ-Fe2O3 MNPs45 |
0.307 |
106 |
APTES-Fe3O446 |
0.836 |
4.72 |
Co3O4 GNs47 |
0.12 |
33.2 |
On the basis of the peroxidase-like activity, the accurate determination of H2O2 has been developed via a colorimetric approach. As the absorbance of the oxidized TMB is proportional to the concentration of H2O2, H2O2 can be detected by monitoring the absorbance changes at 651 nm in UV-vis spectra. Fig. 7a exhibits time-dependent absorbance changes at 651 nm with different concentrations of H2O2 in acetate buffer solution (pH = 4.0). It is obviously shown that the increase of the absorbance at 651 nm is related to the increase of the H2O2 concentration. From a typical H2O2 concentration response curve which is shown in Fig. 7b, it can be calculated that the limit detection of H2O2 is about 0.68 μM (S/N = 3) over a wide linear range from 2 to 40 μM (R2 = 0.9988). This result demonstrates that the as-prepared PB/PPy hybrid nanofibers are excellent peroxidase-like catalysts and have potential application in H2O2 sensing.
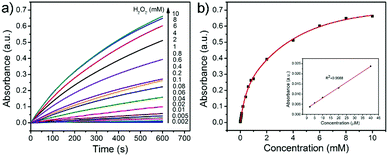 |
| Fig. 7 (a) Time-dependent absorbance changes at 651 nm for the oxidation of TMB (0.1 mM) in the presence of varied concentrations of H2O2 by PB/PPy catalyst suspension (20 μg mL−1). (b) The dependence of the corresponding absorbance changes at 651 nm at 600 s on the concentration of H2O2 and the inset shows the linear calibration curve for the detection of H2O2. | |
L-Cysteine, a vital amino acid for people′s life, has found widespread application in the biomedicine, cosmetic, and food industries. In addition, it can protect vitamins from being oxidized, which can be used in the treatment of bronchitis. Another application of L-cysteine is in detoxification of acrylonitrile poisoning. Hence, the sensitive detection of L-cysteine is in fact indispensable. It has been reported that L-cysteine can decrease the oxidation of TMB and shallow the blue color of the mixing solution. Therefore, we have developed a simple colorimetric approach for the detection of L-cysteine in this work. As depicted in Fig. 8a, the absorbance of the reaction solution decreases as the concentration of L-cysteine increases. In Fig. 8b, a linear calibration curve of ΔA values versus the concentration of L-cysteine over a linear range from 1 to 20 μM (R2 = 0.994) is plotted. And the detection limit for L-cysteine is calculated to be about 16 nM (S/N = 3) on the basis of the linear calibration curve. This detection limit is much better than most of the previously reported nanomaterial-based peroxidase and oxidase-like colorimetric detection methods of L-cysteine (Table 2).12–14,48–52
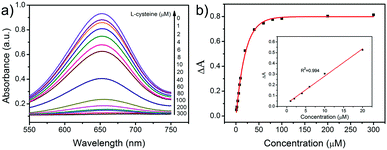 |
| Fig. 8 (a) The absorbance changes of the system consisting of TMB (0.1 mM), H2O2 (65 mM), PB/PPy catalyst suspension (20 μg mL−1) in the absence or presence of various concentrations of L-cysteine. (b) The dose–response curve for detection of L-cysteine and the inset displays the linear calibration plot for the detection of L-cysteine. | |
Table 2 Comparison of the sensing performance of this work with the similar previously reported colorimetric detection methods
Material |
Substrate |
Linear range (μM) |
Limit of detection (nM) |
Ref. |
FeCo NPs/CNTs |
TMB |
1–20 |
150 |
12
|
UiO-66(NH2) |
TMB |
5–120 |
306 |
13
|
NiO NFs |
TMB |
20–100 |
1100 |
14
|
Fe3+ |
TMB |
0–100 |
970 |
48
|
Ce-DMTDC |
TMB |
0–1.0 |
150 |
49
|
Ce-MOF (MVCM) |
TMB |
0–40 |
135 |
50
|
Fe-MIL-88NH2 |
TMB |
1–80 |
390 |
51
|
MnCo2O4 NFs |
TMB |
0.5–10 |
34.4 |
52
|
PB/PPy NFs |
TMB |
1–20 |
16 |
This work |
In consideration of the interferences of other amino acids and metal ions in the human body toward the detection of L-cysteine, selectivity measurements were carried out. In this study, we studied the selectivity for L-cysteine detection via monitoring the absorbance of the reaction systems at 651 nm by replacing L-cysteine with various types of metal ions (including Ca2+, Cl−, HCO3−, K+, Na+, and NO3−), amino acids (including phenylalanine, methionine, lysine, tryptophan, threonine, valine, and histidine), and glucose. The ΔA values of the system containing these species or L-cysteine are shown in Fig. 9a. It is obviously observed that the ΔA values of these control experiments are much lower than that of L-cysteine. And the color of the reaction solution including L-cysteine is completely faded; however, the other solutions still remain blue (Fig. 9b). This result demonstrates that the prepared PB/PPy hybrid nanofibers–H2O2–TMB system exhibits a high selectivity toward the detection of L-cysteine.
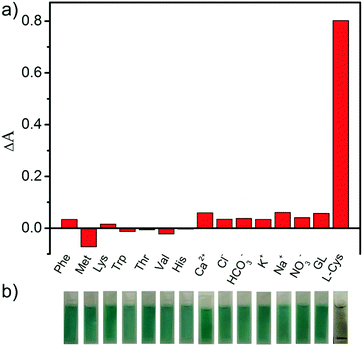 |
| Fig. 9 (a) The difference values of absorbance at 651 nm between 0 min and 10 min in diverse systems containing fixed concentrations of TMB (0.1 mM), H2O2 (65 mM), PB/PPy catalyst suspension (20 μg mL−1) with L-cysteine (0.3 mM) and other interferential substances (0.3 mM); and (b) the corresponding photographs of the above reaction systems on 10 min. | |
Conclusion
In summary, we have demonstrated a facile route to synthesize PB/PPy hybrid nanofibers using α-Fe2O3 nanofibers as both templates and a source of Fe3+ in an acidic environment. The as-prepared PB/PPy hybrid nanofibers show a superior peroxidase catalytic activity compared to individual α-Fe2O3 nanofibers. Owing to the high catalytic efficiency of the as-prepared PB/PPy hybrid nanofibers, a convenient and rapid route for the sensitive determination of H2O2 and L-cysteine with a low detection limit has been developed. This detection system also shows a high selectivity toward L-cysteine with other biological interferential substances such as glucose, other amino acids, and metal ions. This work provides a meaningful approach for the preparation of functional hybrid nanomaterials as peroxidase mimics, which find promising potential applications in the fields of biotechnology, medicine, environmental science, and the food industry.
Conflicts of interest
There are no conflicts to declare.
Acknowledgements
This work was financially supported by the National Natural Science Foundation of China (51473065, 21474043) and the Natural Science Foundation of Heilongjiang Province (B201317).
References
- W. Wang, O. Rusin, X. Xu, K. K. Kim, J. O. Escobedo, S. O. Fakayode, K. A. Fletcher, M. Lowry, C. M. Schowalter, C. M. Lawrence, F. R. Fronczek, I. M. Warner and R. M. Strongin, J. Am. Chem. Soc., 2005, 127, 15949 CrossRef CAS PubMed.
- S. Y. Lim and H. J. Kim, Tetrahedron Lett., 2011, 52, 3189 CrossRef CAS.
- Y. Luo, Z. Shen, P. Liu, L. Zhao and X. Wang, Carbohydr. Polym., 2016, 140, 122 CrossRef CAS PubMed.
- A. Kumar, L. John, M. M. Alam, A. Gupta, G. Sharma, B. Pillai and S. Sengupta, Biochem. J., 2006, 396, 61 CrossRef CAS PubMed.
- H. Mi, M. Guan, J. Liu, H. Shan, Q. Fei, Y. Huan and G. Feng, Spectrochim. Acta, Part A, 2017, 176, 168 CrossRef CAS PubMed.
- C. Han, H. Yang, M. Chen, Q. Su, W. Feng and F. Li, ACS Appl. Mater. Interfaces, 2015, 7, 27968 CAS.
- W. Jin and Y. Wang, J. Chromatogr. A, 1997, 769, 307 CrossRef CAS PubMed.
- N. Cebi, C. E. Dogan, A. Develioglu, M. E. A. Yayla and O. Sagdic, Food Chem., 2017, 228, 116 CrossRef CAS PubMed.
- L. Zhao, J. Peng, M. Chen, Y. Liu, L. Yao, W. Feng and F. Li, ACS Appl. Mater. Interfaces, 2014, 6, 11190 CAS.
- J. Athilakshmi, M. Mohan and D. K. Chand, Tetrahedron Lett., 2013, 54, 427 CrossRef CAS.
- H. Wu, C. Huang, T. Cheng and W. Tseng, Talanta, 2008, 76, 347 CrossRef CAS PubMed.
- Z. Yang, Y. Zhu, G. Nie, M. Li and C. Wang, Dalton Trans., 2017, 46, 8942 RSC.
- Z. Hu, X. Jiang, F. Xu, J. Jia, Z. Long and X. Hou, Talanta, 2016, 158, 276 CrossRef CAS PubMed.
- C. Ray, S. Dutta, S. Sarkar, R. Sahoo, A. Roy and T. Pal, J. Mater. Chem. B, 2014, 2, 6097 RSC.
- Y. Wang, S. Tang, H. Yang and H. Song, Talanta, 2016, 146, 71 CrossRef CAS PubMed.
- J. B. Sumner, J. Biol. Chem., 1926, 69, 435 CAS.
- F. Cramer and W. Kampe, J. Am. Chem. Soc., 1965, 87, 1115 CrossRef CAS PubMed.
- Y. Murakami, J. Kikuchi, Y. Hisaeda and O. Hayashida, Chem. Rev., 1996, 96, 721 CrossRef CAS PubMed.
- H. Wei and E. Wang, Chem. Soc. Rev., 2013, 42, 6060 RSC.
- W. He, W. Wamer, Q. Xia, J. Yin and P. Fu, J. Environ. Sci. Health, Part C: Environ. Carcinog. Ecotoxicol. Rev., 2014, 32, 186 CrossRef CAS PubMed.
- Y. Lin, J. Ren and X. Qu, Acc. Chem. Res., 2014, 47, 1097 CrossRef CAS PubMed.
- Y. Lin, J. Ren and X. Qu, Adv. Mater., 2014, 26, 4200 CrossRef CAS PubMed.
- Q. Zhao, S. Chen, H. Huang, L. Zhang, L. Wang, F. Liu, J. Chen, Y. Zeng and P. Chu, Analyst, 2014, 139, 1498 RSC.
- G. Nie, L. Zhang, J. Lei, L. Yang, Z. Zhang, X. Lu and C. Wang, J. Mater. Chem. A, 2014, 2, 2910 CAS.
- W. He, H. Jia, X. Li, Y. Lei, J. Li, H. Zhao, L. Mi, L. Zhang and Z. Zheng, Nanoscale, 2012, 4, 3501 RSC.
- Y. Song, K. Qu, C. Zhao, J. Ren and X. G. Qu, Adv. Mater., 2010, 22, 2206 CrossRef CAS PubMed.
- D. Feng, Z. Gu, J. Li, H. Jiang, Z. Wei and H. Zhou, Angew. Chem., Int. Ed., 2012, 51, 10307 CrossRef CAS PubMed.
- G. Nie, L. Zhang, X. Lu, X. Bian, W. Sun and C. Wang, Dalton Trans., 2013, 42, 14006 RSC.
- X. Sun, S. Guo, C. Chung, W. Zhu and S. Sun, Adv. Mater., 2013, 25, 132 CrossRef CAS PubMed.
- Y. Guo, H. Wang, X. Ma, J. Jin, W. Ji, X. Wang, W. Song, B. Zhao and C. He, ACS Appl. Mater. Interfaces, 2017, 9, 19074 CAS.
- W. Song, M. Chi, M. Gao, B. Zhao, C. Wang and X. Lu, J. Mater. Chem. A, 2017, 5, 7465 CAS.
- J. Jin, S. Zhu, Y. Song, H. Zhao, Z. Zhang, Y. Gou, J. Li, W. Song, B. Yang and B. Zhao, ACS Appl. Mater. Interfaces, 2016, 8, 27956 CAS.
- M. Chi, Y. Zhu, Z. Yang, M. Gao, S. Chen, N. Song, C. Wang and X. Lu, Nanotechnology, 2017, 28, 295704 CrossRef PubMed.
- Y. Zhou, J. Cheng, C. Xiang, H. Chu, S. Qiu, F. Xu and L. Zheng, Int. J. Electrochem. Sci., 2015, 10, 4626 Search PubMed.
- Z. Yang, X. Zheng and J. Zheng, Ind. Eng. Chem. Res., 2016, 55, 12161 CrossRef CAS.
- G. Nie, X. Lu, J. Lei, Z. Jiang and C. Wang, J. Mater. Chem. A, 2014, 2, 15495 CAS.
- H. Maaoui, R. Jijie, G. H. Pan, D. Drider, D. Caly, J. Bouckaert, N. Dumitrascu, R. Chtourou, S. Szunerits and R. Boukherroub, J. Colloid Interface Sci., 2016, 480, 63 CrossRef CAS PubMed.
- Y. Jiang, N. Song, C. Wang, N. Pinna and X. Lu, J. Mater. Chem. B, 2017, 5, 5499 RSC.
- J. Wang, Y. Xu, Y. Feng, J. Zhu and J. Wang, J. Power Sources, 2011, 196, 2373 CrossRef CAS.
- R. Chen, Q. Zhang, Y. Gu, L. Tang, C. Li and Z. Zhang, Anal. Chim. Acta, 2015, 853, 579 CrossRef CAS PubMed.
- M. Sookhakian, W. J. Basirun, M. M. Teridi, M. R. Mahmoudian, M. Azarang, E. Zalnezhad, G. H. Yoon and Y. Alias, Electrochim. Acta, 2017, 230, 316 CrossRef CAS.
- P. Ni, Y. Sun, H. Dai, W. Lu, S. Jiang, Y. Wang, Z. Li and Z. Li, Sens. Actuators, B, 2017, 240, 1314 CrossRef CAS.
- W. Zhang, D. Ma and J. Du, Talanta, 2014, 120, 362 CrossRef CAS PubMed.
- L. Gao, J. Zhuang, L. Nie, J. Zhang, Y. Zhang, N. Gu, T. Wang, J. Feng, D. Yang, S. Perrett and X. Yan, Nat. Nanotechnol., 2007, 2, 577 CrossRef CAS PubMed.
- X. Zhang, S. Gong, Y. Zhang, T. Yang, C. Wang and N. Gu, J. Mater. Chem., 2010, 20, 5110 RSC.
- H. Zhu, Y. Hu, G. Jiang and G. Shen, Eur. Food Res. Technol., 2011, 233, 881 CrossRef CAS.
- J. Yin, H. Cao and Y. Lu, J. Mater. Chem., 2012, 22, 527 RSC.
- X. Q. Wu, Y. Xu, Y. L. Chen, H. Zhao, H. J. Cui, J. S. Shen and H. W. Zhang, RSC Adv., 2014, 4, 64438 RSC.
- R. Dalapati, S. Balasubramanian, M. K. Ghosalya, A. Dhakshinamoorthy and S. Biswas, CrystEngComm, 2017, 19, 5915 RSC.
- Y. Xiong, S. Chen, F. Ye, L. Su, C. Zhang, S. Shen and S. Zhao, Chem. Commun., 2015, 51, 4635 RSC.
- Z. Jiang, Y. Liu, X. Hua and Y. Li, Anal. Methods, 2014, 6, 5647 RSC.
- M. Gao, X. F. Lu, M. Q. Chi, S. H. Chen and C. Wang, Inorg. Chem. Front., 2017, 4, 1862 RSC.
|
This journal is © the Partner Organisations 2018 |
Click here to see how this site uses Cookies. View our privacy policy here.