DOI:
10.1039/C8QM00060C
(Research Article)
Mater. Chem. Front., 2018,
2, 1284-1290
Highly efficient yellow electroluminescence of iridium complexes with good electron mobility†
Received
6th February 2018
, Accepted 12th March 2018
First published on 13th March 2018
Abstract
Herein, two iridium(III) complexes with yellow emission using 1-(3,5-bis(trifluoromethyl)phenyl)isoquinoline (tfmphiq) and 4-(3,5-bis(trifluoromethyl)phenyl)quinazoline (tfmphqz) as the main ligands and tetraphenylimidodiphosphinate (tpip) as the ancillary ligand were applied in organic light-emitting diodes (OLEDs). The quinazoline moiety greatly influenced the nature of the complexes, and both the quantum yield and the electron mobility of Ir(tfmphqz)2(tpip) were much higher than that of Ir(tfmphiq)2(tpip) (Ir(tfmphiq)2(tpip) (λem: 581 nm and Φ: 0.61) and Ir(tfmphqz)2(tpip) (λem: 566 nm and Φ: 0.97)). Moreover, the device based on Ir(tfmphqz)2(tpip) displayed far better performance than that based on the Ir(tfmphiq)2(tpip) emitter. The device with a structure of ITO/MoO3 (3 nm)/TAPC (50 nm)/Ir(tfmphqz)2(tpip) (6 wt%): 26DCzPPy (10 nm)/TmPyPB (50 nm)/LiF (1 nm)/Al (100 nm) displayed a maximum luminance of 96
377 cd m−2 and a maximum current efficiency and a maximum power efficiency of 106.66 cd A−1 and 64.72 lm W−1, respectively, with low efficiency roll-off. The current efficiency still remains as high as 89.91 cd A−1 at the brightness of 1000 cd m−2 and 79.73 cd A−1 at the brightness of 5000 cd m−2. These results suggest that the Ir(III) complexes with quinazoline units are potential yellow phosphorescent materials for OLEDs.
Introduction
The phosphorescent iridium(III) complexes have been extensively studied in organic light-emitting diodes (OLEDs) because of their high quantum efficiency and their wide range of emission colors.1 The strong spin–orbit coupling (SOC) can promote triplet to ground state radiative transition by introducing the central heavy metal atom, which leads to a quite high phosphorescence quantum efficiency. Furthermore, the phosphorescence of Ir(III) complexes generated by the metal-to-ligand charge transfer (MLCT) and ligand-centered (LC) transition2 makes it possible to control the energy level of the excited state by adjusting ligands via a substituent effect.
Over the past decade, many efficient red, green and blue OLEDs have been developed.3 However only few yellow OLEDs have been reported.4 Yellow OLEDs can be used in lithography labs, traffic and signal lights, and high quality RGBY-TV. Moreover, they are one of the most crucial emitting devices for the fabrication of a physiologically friendly low-color temperature lighting source due to their lesser suppression effect on melatonin secretion.5 Therefore, the development of high-efficiency yellow OLEDs is also important.
As is well-known, the balance between the electron–hole injection and transport is necessary for high-efficiency OLEDs obtained using the Ir(III) complexes because both the charge carrier balance deterioration and increase in the non-radioactive quenching process will cause a serious efficiency roll-off. Since the hole mobility of majority of the hole-transporting materials is much higher than the electron mobility of electron-transporting materials, the performance of OLEDs depends on the electron-transport capability. Therefore, the use of bipolar host materials to gain phosphorescent OLEDs with low efficiency roll-off and the synthesis of dopants with excellent electron mobility are essential.6
In our previous study, we have found that tetraphenylimidodiphosphinate (tpip) is a good ancillary ligand for Ir(III) complexes due to its four bulky aromatic groups, leading to an increase in spatial separation from neighboring molecules to suppress TTA (triplet–triplet annihilation) and TPA (triplet-polaron annihilation) effectively,7 as well as the ability of Ph2P
O to improve the electron injection and transport capabilities.8 Moreover, the bulky trifluoromethyl (CF3) substituents can affect the molecular packing, the steric protection surrounding the metal would restrain the self-quenching impact, and the C–F bond with low vibrational frequency can reduce the radiationless deactivation rate.9 Both of them can enhance the electron affinity and electron mobility of the Ir(III) complexes.
Therefore, with an aim to obtain yellow emitters and devices with high efficiency by improving the electron mobility, two complexes Ir(tfmphiq)2(tpip) (tfmphiq = 1-(3,5-bis(trifluoromethyl) phenyl)isoquinoline) and Ir(tfmphqz)2(tpip) (tfmphqz = 4-(3,5-bis(trifluoromethyl)phenyl)quinazoline) with nitrogen heterocyclic main ligands and the tpip ancillary ligand were synthesized. The device based on Ir(tfmphqz)2(tpip) displayed far better performances than that based on Ir(tfmphiq)2(tpip), showing a maximum current efficiency (ηc,max) of 106.66 cd A−1 with low efficiency roll-off.
Results and discussion
Preparation and X-ray crystallography
Scheme 1 shows the chemical structures and synthetic routes of Ir(III) complexes. The two ligands tfmphiq and tfmphqz were synthesized using a Suzuki coupling reaction. Tetraphenylimidodiphosphinate acid (Htpip) and potassium salt (Ktpip) were prepared according to our previous studies. The iridium complexes were obtained in two steps via classical methods using an Ir(III) chloro-bridged dimer. Purification of the mixture by silica gel chromatography provided crude products, which were further purified by vacuum sublimation. All the new compounds were fully characterized by 1H NMR and electrospray ionization mass spectra (ESI-MS).
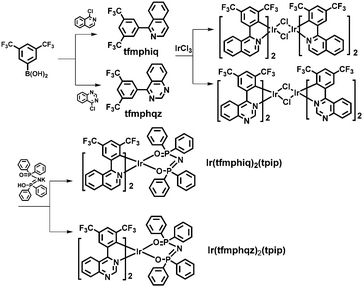 |
| Scheme 1 Synthetic routes of ligands and complexes. | |
Furthermore, the structure of Ir(tfmphqz)2(tpip) complex was confirmed via single-crystal measurement, and the crystal diagram is displayed in Fig. 1. The molecular parameters and atomic coordinates were obtained, as presented in the Tables S1 and S2 (ESI†), respectively. From the crystal diagrams, it can be found that the iridium atom is embraced by C, N, and O atoms from tfmphqz and tpip, with a twisted octahedral coordination geometry. The angle of [O–Ir–O] is 92.32(12)°, and the angles of [C–Ir–N] are 80.08(17)°–80.67(17)°. The bond lengths of Ir–C, Ir–N, and Ir–O are similar to the parameters of the cyclometalated Ir(III) complexes reported in literature.
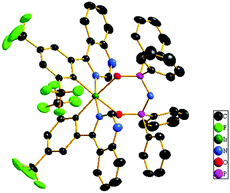 |
| Fig. 1 The crystal diagram of Ir(tfmphqz)2(tpip) (CCDC 1582727) shown at a 30% probability level.† The hydrogen atoms are omitted for clarity. | |
Thermal stability
The thermal stability of materials is crucial for efficient OLEDs. To apply a complex in practical OLEDs, the decomposition temperature (Td) needs to be high enough to guarantee that the complex can be deposited by sublimation in vacuum without any decomposition. The thermogravimetric analysis (TGA) curves of Ir(tfmphiq)2(tpip) and Ir(tfmphqz)2(tpip) are shown in Fig. S1 (ESI†). It can be figured that both complexes show good thermal stability, and the decomposition temperature (5% loss of weight) is 300 °C for Ir(tfmphiq)2(tpip) and 360 °C for Ir(tfmphqz)2(tpip).
Electrochemical property and theoretical calculation
The frontier molecular orbitals (FMOs), especially the highest occupied molecular orbital (HOMO) and lowest unoccupied molecular orbital (LUMO) levels, are quite crucial to the design of a device structure. Therefore, to measure the energy levels of the HOMO/LUMO, electrochemistry measurements by cyclic voltammetry (CV) were adopted with ferrocene as the internal standard in CH3CN (Fig. S2, ESI†). The HOMO level was obtained via the oxidation potential, and then, the LUMO level was calculated by the HOMO and the band gap observed from the UV-vis absorption spectra. During the progress of anodic oxidation, an obvious oxide peak can be observed for all complexes with oxidation potential ranging 1.15–1.27 V, which can be ascribed to the metal-centered Ir(III)/Ir(IV) oxide couple.10Ir(tfmphiq)2(tpip) exhibits a lower oxidation potential (1.15 V), and the HOMO/LUMO energy levels are −5.81/−3.69 eV. The oxidation potential of Ir(tfmphqz)2(tpip) increases to 1.27 V, and the HOMO/LUMO energy levels are −5.91/−4.00 eV.
Furthermore, it can be observed from the theoretical calculation that nearly all LUMOs are on tfmphiq/tfmphqz ligands (94.40–95.27%) and rarely situated on the iridium atom (3.06–3.23%) and tpip ancillary ligand (1.50–2.55%). On the other hand, HOMOs are situated on tfmphiq ligands (50.44%) and d orbitals of the iridium atom (44.99%) and tpip ancillary ligand (4.57%) for Ir(tfmphiq)2(tpip). For Ir(tfmphqz)2(tpip), HOMOs are situated on the tfmphqz ligands (41.21%) and d orbitals of the iridium atom (51.49%) and tpip ancillary ligand (7.31%).11 The introduction of quinazoline influences the electron cloud distribution of the complexes, resulting in reduction of the HOMO and LUMO levels, which leads to the differences in the nature of Ir(tfmphiq)2(tpip) and Ir(tfmphqz)2(tpip). Moreover, the reduction of the LUMO level is beneficial to trap electrons and broaden the recombination zone, leading to a partial improvement in the performance of the EL. As a result, there is a great chance that the difference in the photophysical property and the EL performances is very apparent between Ir(tfmphiq)2(tpip) and Ir(tfmphqz)2(tpip).
Photophysical property
The UV-vis absorption and photoluminescence spectra of the two complexes Ir(tfmphiq)2(tpip) and Ir(tfmphqz)2(tpip) in CH2Cl2 (5 × 10−5 M) are shown in Fig. 2. The broad and intensive absorption bands below 300 nm are due to the spin-allowed intraligand 1LC (π–π*) transition of tfmphiq/tfmphqz and tpip ligands. The weak absorption lasting to 550 nm should be attributed to the metal–ligand charge transfer band (MLCT) and/or broad LC absorptions. The strongest emissions peaks at 581 and 566 nm in CH2Cl2 are due to the electronic transition between the lowest triplet excited state and the ground state that makes Ir(tfmphiq)2(tpip) and Ir(tfmphqz)2(tpip) emit yellow phosphorescence. The emissions in the region of lower energy around 623 nm may be generated by the overlapping of vibrational satellites.12,13 Furthermore, the complexes show a high quantum efficiency of 0.61 and 0.97 for Ir(tfmphiq)2(tpip) and Ir(tfmphqz)2(tpip), respectively. The differences in the absorption and emission profiles of Ir(tfmphiq)2(tpip) and Ir(tfmphqz)2(tpip) suggest that the introduction of quinazoline unit has an obvious effect, and it can increase the quantum efficiency greatly (Table 1).
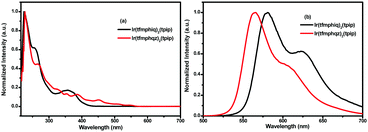 |
| Fig. 2 (a) UV-vis absorption and (b) emission spectra of Ir(tfmphiq)2(tpip) and Ir(tfmphqz)2(tpip) complexes in degassed CH2Cl2 solutions (5.0 × 10−5 mol L−1) at room temperature. | |
Table 1 Physical properties of Ir(tfmphiq)2(tpip) and Ir(tfmphqz)2(tpip)
Complex |
T
d
(°C) |
λ
abs
(nm) |
λ
em
(nm) |
Φ
|
HOMO/LUMOe (eV) |
T
d: decomposition temperature.
Measured in a degassed CH2Cl2 solution at a concentration of 5 × 10−5 mol L−1 at room temperature.
Measured in a degassed CH2Cl2 solution at a concentration of 5 × 10−5 mol L−1 at room temperature.
Measured in a degassed CH2Cl2 solution at a concentration of 5 × 10−5 mol L−1 at room temperature emission relative to Ir(ppy)3 (Φ = 0.4).
From the onset of oxidation potentials of the cyclic voltammetry (CV) diagram using ferrocene as the internal standard and the optical band gap from the absorption spectra in the degassed solution (CH3CN).
|
Ir(tfmphiq)2(tpip)
|
300 |
224/254/357 |
581 |
0.61 |
−5.81/−3.69 |
Ir(tfmphqz)2(tpip)
|
361 |
227/266/387/452 |
566 |
0.97 |
−5.91/−4.00 |
Electron mobility
According to our previous studies, an excellent electron mobility of emitters could benefit electron transport and improve the device efficiency.14 To determine the electron mobility of the two complexes, the transient electroluminescence (TEL) measurements were carried out using the devices with a structure of ITO (indium-tin-oxide)/TAPC (1,1-bis[4-[N,N-di(p-tolyl)amino]phenyl]cyclohexane, 50 nm)/Ir complexes (60 nm).15 The Ir(III) complexes act not only as an emissive layer (EML) but also as an electron-transport layer (ETL). The experimental results (Fig. 3) indicate that the electron mobility values of Ir(tfmphiq)2(tpip) and Ir(tfmphqz)2(tpip) are 8.14–8.61 × 10−6 cm2 V−1 s−1 and 9.38–10.00 × 10−6 cm2 V−1 s−1, respectively, under the electric field from 1040 (V cm−1)1/2 to 1300 (V cm−1)1/2, higher than that of the popularly used electron transport material Alq3 (aluminum 8-hydroxyquinolinate, 4.74–4.86 × 10−6 cm2 V−1 s−1).16 Moreover, the electron mobility of Ir(tfmphqz)2(tpip) is better than as that of Ir(tfmphiq)2(tpip), which suggests that the quinazoline moiety has a strong impact on the enhancement of the electron flow due to an additional nitrogen atom. The result exactly fits the intent of our design to improve the electron transport ability of the emitter. The good electron transport ability of Ir(tfmphiq)2(tpip) and Ir(tfmphqz)2(tpip) will improve the chance of electron and hole recombination, so that their devices may have good performances, especially for those using Ir(tfmphqz)2(tpip).
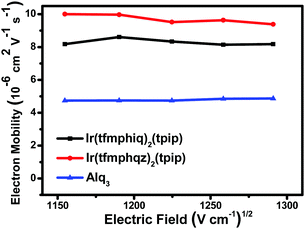 |
| Fig. 3 Electric field dependence of charge electron mobility in the thin films of Ir(tfmphiq)2(tpip) and Ir(tfmphqz)2(tpip) and Alq3. | |
OLED performance
To confirm our speculation, we researched the single-emitting layer (EML) devices named G1 and G2 using Ir(tfmphiq)2(tpip) and Ir(tfmphqz)2(tpip) emitters, respectively, with a structure of ITO/MoO3 (molybdenum oxide, 3 nm)/TAPC (50 nm)/Ir complex (x wt%): 26DCzPPy (2,6-bis(3-(carbazol-9-yl)phenyl) pyridine, 10 nm)/TmPyPB (1,3,5-tri(m-pyrid-3-yl-phenyl) benzene, 50 nm)/LiF (1 nm)/Al (100 nm) (Fig. 4). MoO3 and LiF served as the hole- and electron-injecting interface-modified materials, respectively. Owning high hole mobility (1 × 10−2 cm2 V−1 s−1) and high-lying LUMO level (−2.0 eV), TAPC was used as hole transport/electron block layer (HTL/EBL), while TmPyPB was used as electron transport/hole block layer (ETL/HBL) with high electron mobility (1 × 10−3 cm2 V−1 s−1) and low-lying HOMO level (−6.7 eV). The ambipolar material 26DCzPPy has been chosen as the host because of its nearly equal electron mobility (μe) and hole mobility (μh) values (1–8 × 10−5 cm2 V−1 s−1 at an electric field between 6.0 × 105 and 1.0 × 106 V cm−1), which benefits the electron–hole balance in the EML.17–19 Optimal device performances are achieved at a doping level of 6 wt% for Ir(tfmphiq)2(tpip) and Ir(tfmphqz)2(tpip).
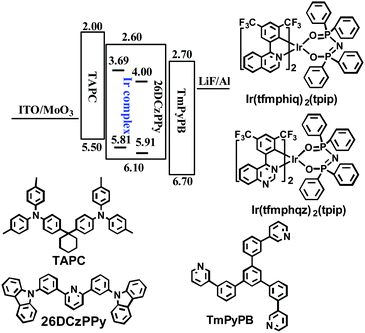 |
| Fig. 4 Energy level diagram of HOMO and LUMO levels of materials used in this study and molecular structures of these materials. | |
The EL spectra, luminance–voltage–current density (L–V–J) curves, current efficiency–luminance (ηc–L) curves, and power efficiency–luminance (ηp–L) curves of G1 and G2 are shown in Fig. 5, and the crucial EL data are shown in Table 2. The peaks of EL emission are at 578 and 560 nm for G1 and G2, respectively. The emission spectra are almost invariant of the current density, and there is no dependence on concentration. Moreover, it can be seen that the EL spectra are almost identical to the PL spectra of the complexes suggesting that the EL emissions of the devices originate from the triplet excited states of the phosphors. Apart from the characteristic emission of Ir(III) complexes, these devices displayed weak emissions ranging from 350 to 500 nm, which originated from the host 26DCzPPy due to the accumulation of holes and electrons within the EML, and few holes/electrons can recombine on 26DCzPPy molecules. The Commission Internationale de 1’Eclairage (CIE) color coordinates of G1 and G2 are (0.556, 0.427) and (0.469, 0.522), respectively.
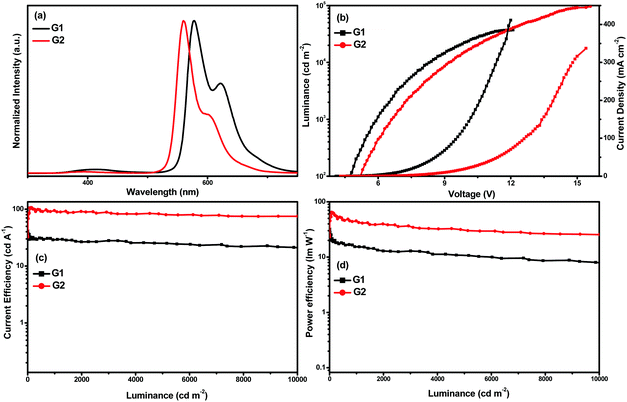 |
| Fig. 5 Characteristics devices G1 and G2: (a) electroluminescence spectra (8 V); (b) luminance–voltage–current density (L–V–J) curves; (c) current efficiency–luminance (ηc–L) curves; and (d) power efficiency–luminance (ηp–L) curves. | |
Table 2 EL performances of G1 and G2
Device |
V
turn-on
(V) |
L
max
(cd m−2) |
η
c,max
(cd A−1) |
η
c,L1000
(cd A−1) |
EQEmaxe (%) |
η
p,max
(lm W−1) |
CIE(x, y) |
V
turn-on: turn-on voltage obtained at a luminance of 1 cd m−2.
L
max: maximum luminance.
η
c,max: maximum current efficiency.
η
c,L1000: current efficiency at 1000 cd m−2.
EQEmax: maximum external quantum efficiency.
η
p,max: maximum power efficiency.
|
G1
|
3.9 |
37 208 |
41.57 |
29.33 |
16.7 |
37.46 |
0.556, 0.427 |
G2
|
4.3 |
96 377 |
106.66 |
89.91 |
29.7 |
64.72 |
0.469, 0.522 |
For the device G1, a maximum current efficiency (ηc,max) of 41.57 cd A−1 (4.1 V) with a maximum external quantum efficiency (EQEmax) of 16.7%, a maximum power efficiency (ηp,max) of 31.84 lm W−1 (4.1 V), and a maximum luminance (Lmax) of 37
208 cd m−2 were obtained. G2 exhibits much better performances with an Lmax of 96
377 cd m−2 at 15.6 V, a ηc,max of 106.66 cd A−1 (5.3 V), an EQEmax of 29.7%, and a ηp,max of 64.72 (5.1 V) lm W−1. Moreover, G2 can remain highly efficient even at relatively high luminance values, and the EL efficiency roll-off is rather low. For instance, the current efficiency for the device G2 still remains as high as 89.91 cd A−1 at the brightness of 1000 cd m−2 and 79.73 cd A−1 at the brightness of 5000 cd m−2, suggesting that the complex is a promising material for OLEDs.
From the device performances, it can be concluded that the introduction of quinazoline has definitely improved the electron mobility of the iridium complexes. As a result, the recombination chance of electrons and holes has been strengthened, which is beneficial to the improvement of OLED performances.
Conclusions
In conclusion, we have prepared two bis-cyclometalated iridium complexes, Ir(tfmphiq)2(tpip) and Ir(tfmphqz)2(tpip), with 1-(3,5-bis(trifluoromethyl)phenyl)isoquinoline (tfmphiq) and 4-(3,5-bis(trifluoromethyl)phenyl)quinazoline (tfmphqz) as the main ligands and tetraphenylimidodiphosphinate (tpip) as the ancillary ligand. Both complexes emit yellow phosphorescence with a high quantum efficiency and good electron mobility, especially Ir(tfmphqz)2(tpip). Compared to the device based on Ir(tfmphiq)2(tpip), the device based on Ir(tfmphqz)2(tpip) displays better EL performances and lower efficiency roll-off, with an Lmax of 96
378 cd m−2, a ηc,max of 106.66 cd A−1, and an EQEmax of 29.7%. The results suggest that the Ir(III) complexes with quinazoline units are potential yellow phosphorescent materials for OLEDs.
Experimental
The general information including the 1H NMR spectra, electrospray ionization mass spectra (ESI-MS), elemental analyses for C, H and N, TG-DSC, UV-vis absorption and photoluminescence spectra, cyclic voltammetry, X-ray crystallography, and OLEDs measurements are listed in the ESI† section.
Syntheses
All reagents used were of commercial grade. The ligands and complexes were synthesized under a nitrogen atmosphere, and the synthetic routes are listed in Scheme 1.
General syntheses of ligands.
1-Chloroisoquinoline or 4-chloroquinazoline (10 mmol), bis(diphenylphosphino) ferrocene palladium(II) dichloromethane (0.3 mmol), and (3,5-bis(trifluoromethyl)phenyl)boronic acid were added to 50 mL THF. After the addition of 20 mL of aqueous 2 N K2CO3, the reaction mixture was heated at 70 °C for 1 day under a nitrogen atmosphere. The mixture was poured into water and extracted with CH2Cl2 (10 mL × 3 times). Finally, silica column purification (PE
:
EA = 10
:
1 as eluant) provided the ligand 1-(3,5-bis(trifluoromethyl)phenyl)isoquinoline or 4-(3,5-bis(trifluoromethyl)phenyl)quinazoline.
1-(3,5-Bis(trifluoromethyl)phenyl)isoquinoline.
40% yield. 1H NMR (400 MHz, CDCl3) δ 8.66 (d, J = 5.4 Hz, 1H), 8.20 (s, 2H), 8.03 (s, 1H), 7.95 (dd, J = 7.8, 5.9 Hz, 2H), 7.77 (t, J = 7.0 Hz, 2H), 7.68–7.59 (m, 1H). MS (ESI) m/z calcd for C17H9F6N+: 341.26 [M]+, found: 342.31 [M + H]+.
4-(3,5-Bis(trifluoromethyl)phenyl)quinazoline.
30% yield. 1H NMR (400 MHz, CDCl3) δ 9.45 (s, 1H), 8.28 (s, 2H), 8.21 (d, J = 8.4 Hz, 1H), 8.11 (s, 1H), 8.04–7.95 (m, 2H), 7.73 (t, J = 7.7 Hz, 1H). MS (ESI) m/z calcd for C16H8F6N2+[M]+: 342.24, found 343.26 [M + H]+.
General syntheses of iridium complexes.
A mixture of IrCl3 (1 mmol) and tfmphiq or tfmphqz (2.5 mmol) in 2-ethoxyethanol and water (20 mL, 3
:
1, v/v) was refluxed for 24 h. After cooling, the solid precipitate was filtered to obtain the crude cyclometalated Ir(III) chloro-bridged dimer. Then, the slurry of crude chloro-bridged dimer (0.2 mmol) and Ktpip (0.5 mmol) in 2-ethoxyethanol (20 mL) was refluxed for 24 h. The solvent was evaporated at low pressure, and the mixture was poured into water, extracted with CH2Cl2 (10 mL × 3 times), and then chromatographed to obtain the complexes Ir(tfmphiq)2(tpip) and Ir(tfmphqz)2(tpip), which were further purified by sublimation in vacuum.
Ir(tfmphiq)2(tpip)
.
46% yield. 1H NMR (400 MHz, CDCl3) δ 8.78 (d, J = 6.5 Hz, 2H), 8.69 (d, J = 8.6 Hz, 2H), 8.53 (s, 2H), 7.75–7.66 (m, 6H), 7.63 (t, J = 7.1 Hz, 2H), 7.54 (d, J = 7.8 Hz, 2H), 7.50 (s, 2H), 7.41–7.29 (m, 6H), 7.01–6.93 (m, 4H), 6.74–6.65 (m, 4H), 6.49 (td, J = 7.7, 3.3 Hz, 4H). MS (ESI) m/z calcd for C58H36F12IrN3O2P2: 1289.09 [M]+, found 1290.17 [M + H]+. Anal. calcd for C58H36F12IrN3O2P2: C 54.04, H 2.82, N 3.26. Found: C 53.97, H 2.95, N 3.05.
Ir(tfmphqz)2(tpip)
.
27% yield. 1H NMR (400 MHz, CDCl3) δ 9.58 (d, J = 1.3 Hz, 2H), 8.64 (s, 2H), 8.56 (d, J = 8.6 Hz, 2H), 7.88–7.82 (m, 4H), 7.77 (ddd, J = 8.4, 5.4, 2.9 Hz, 2H), 7.71 (ddd, J = 12.4, 7.7, 1.7 Hz, 4H), 7.59 (s, 2H), 7.41–7.34 (m, 6H), 7.02–6.93 (m, 4H), 6.66–6.60 (m, 2H), 6.52 (td, J = 7.5, 3.2 Hz, 4H). MS (ESI) m/z calcd for C56H34F12IrN5O2P2: 1291.07 [M]+, found 1292.19 [M + H]+. Anal. calcd for C56H34F12IrN5O2P2: C 52.10, H 2.65, N 5.42. Found: C 51.94, H 2.76, N 5.39.
Conflicts of interest
There are no conflicts to declare.
Acknowledgements
This work was supported by the National Natural Science Foundation of China (51773088) and the Natural Science Foundation of Jiangsu Province (BY2016075-02).
Notes and references
-
(a) S. Lamansky, P. Djurovich, D. Murphy, F. Abdel-Razzaq, H. E. Lee, C. Adachi, P. E. Burrow, S. R. Forrest and M. E. Thompson, J. Am. Chem. Soc., 2001, 123, 4304 CrossRef CAS PubMed;
(b) J. J. Kim, Y. You, Y. S. Park, J. J. Kim and S. Y. Park, J. Mater. Chem., 2009, 19, 8347 RSC;
(c) Z. Q. Chen, Z. Q. Bian and C. H. Huang, Adv. Mater., 2010, 22, 1534 CrossRef CAS PubMed;
(d) S. M. Chen, G. P. Tan, W. Y. Wong and H. S. Kwok, Adv. Funct. Mater., 2011, 21, 3785 CrossRef CAS;
(e) J. M. Fernández-Hernández, C. H. Yang, J. I. Beltrán, V. Lemaur, F. Polo, R. Fröhlich, J. Cornil and L. D. Cola, J. Am. Chem. Soc., 2011, 133, 10543 CrossRef PubMed;
(f) K. Y. Lu, H. H. Chou, C. H. Hsieh, Y. H. O. Yang, H. R. Tsai, H. Y. Tsai, L. C. Hsu, C. Y. Chen, I. C. Chen and C. H. Cheng, Adv. Mater., 2011, 23, 4933 CrossRef CAS PubMed;
(g) C.-H. Fan, P. Sun, T.-H. Su and C.-H. Cheng, Adv. Mater., 2011, 23, 2981 CrossRef CAS PubMed;
(h) S. Lee, S. O. Kim, H. Shin, H. J. Yun, K. Yang, S. K. Kwon, J. J. Kim and Y. H. Kim, J. Am. Chem. Soc., 2013, 135, 14321 CrossRef CAS PubMed;
(i) X. L. Yang, N. Sun, J. S. Dang, Z. Huang, C. L. Yao, X. B. Xu, C. L. Ho, G. J. Zhou, D. G. Ma, X. Zhao and W. Y. Wong, J. Mater. Chem. C, 2013, 1, 3317 RSC;
(j) H. Sasabe, H. Nakanishi, Y. Watanabe, S. Yano, M. Hirasawa, Y. J. Pu and J. Kido, Adv. Funct. Mater., 2013, 23, 5550 CrossRef CAS;
(k) H.-H. Chou, Y.-K. Li, Y.-H. Chen, C.-C. Chang, C.-Y. Liao and C.-H. Cheng, ACS Appl. Mater. Interfaces, 2013, 5, 6168 CrossRef CAS PubMed;
(l) V. K. Rai, M. Nishiura, M. Takimoto and Z. Hou, J. Mater. Chem. C, 2014, 2, 5317 RSC;
(m) H. Cao, H. Sun, Y. Yin, X. Wen, G. Shan, Z. Su, R. N. Zhong, W. Xie, P. Li and D. Zhu, J. Mater. Chem. C, 2014, 2, 2150 RSC;
(n) A. Graf, P. Liehm, C. Murawski, S. Hofmann, K. Leo and M. C. Gather, J. Mater. Chem. C, 2014, 2, 10298 RSC;
(o) X. Xu, X. Yang, J. Dang, G. Zhou, Y. Wu, H. Li and W.-Y. Wong, Chem. Commun., 2014, 50, 2473 RSC;
(p) X. Yang, G. Zhou and W.-Y. Wong, J. Mater. Chem. C, 2014, 2, 1760 RSC.
- W. S. Jeon, T. J. Park, S. Y. Kim, R. Pode, J. Jang and J. H. Kwon, Appl. Phys. Lett., 2008, 93, 063303 CrossRef.
-
(a) M. C. Gather, A. Kohnen and K. Meerholz, Adv. Mater., 2011, 23, 233 CrossRef CAS PubMed;
(b) C. H. Chien, F. M. Hsu, C. F. Shu and Y. Chi, Org. Electron., 2009, 10, 871 CrossRef CAS;
(c) J. H. Jou, P. H. Wu, C. H. Lin, M. H. Wu, Y. C. Chou, H. C. Wang and S. M. Shen, J. Mater. Chem., 2010, 20, 8464 RSC;
(d) T. Chiba, Y. J. Pu, R. Miyazaki, K. Nakayama, H. Sasabe and J. Kido, Org. Electron., 2011, 12, 710 CrossRef CAS;
(e) H. Sasabe, J. Takamatsu, T. Motoyama, S. Watanabe, G. Wagenblast, N. Langer, O. Molt, E. Fuchs, C. Lennartz and J. Kido, Adv. Mater., 2010, 22, 5003 CrossRef CAS PubMed;
(f) D. Tanaka, H. Sasabe, Y. J. Li, S. J. Su, T. Takeda and J. Kido, Jpn. J. Appl. Phys., Part 2, 2007, 46, L10 CrossRef CAS;
(g) N. Chopra, J. S. Swensen, E. Polikarpov, L. Cosimbescu, F. So and A. B. Padmaperuma, Appl. Phys. Lett., 2010, 97, 033304 CrossRef;
(h) J. H. Jou, M. F. Hsu, W. B. Wang, C. L. Chin, Y. C. Chung, C. T. Chen, J. J. Shyue, S. M. Shen, M. H. Wu, W. C. Chang, C. P. Liu, S. Z. Chen and H. Y. Chen, Chem. Mater., 2009, 21, 2565 CrossRef CAS;
(i) C. L. Ho, L. C. Chi, W. Y. Hung, W. J. Chen, Y. C. Lin, H. Wu, E. Mondal, G. J. Zhou, K. T. Wong and W. Y. Wong, J. Mater. Chem., 2012, 22, 215 RSC;
(j) H. H. Chou and C. H. Cheng, Adv. Mater., 2010, 22, 2468 CrossRef CAS;
(k) T. H. Han, Y. Lee, M. R. Choi, S. H. Woo, S. H. Bae, B. H. Hong, J. H. Ahn and T. W. Lee, Nat. Photonics, 2012, 6, 105 CrossRef CAS.
-
(a) C. L. Chiang, S. M. Tseng, C. T. Chen, C. P. Hsu and C. F. Shu, Adv. Funct. Mater., 2008, 18, 248 CrossRef CAS;
(b) J. Zhao, J. S. Yu, Z. Ma, L. Liand and Y. D. Jiang, Synth. Met., 2011, 161, 2417 CrossRef CAS;
(c) M. Velusamy, C. H. Chen, Y. S. Wen, J. T. Lin, C. C. Lin, C. H. Lai and P. T. Chou, Organometallics, 2010, 29, 3912 CrossRef CAS;
(d) C. T. Chen, Y. Wei, J. S. Lin, M. Moturu, W. S. Chao, Y. T. Tao and C. H. Chien, J. Am. Chem. Soc., 2006, 128, 10992 CrossRef CAS PubMed;
(e) G. J. Zhou, C. L. Ho, W. Y. Wong, Q. Wang, D. G. Ma, L. X. Wang, Z. Y. Lin, T. B. Marder and A. Beeby, Adv. Funct. Mater., 2008, 18, 499 CrossRef CAS;
(f) G. P. Ge, J. He, H. Q. Guo, F. Z. Wang and D. C. Zou, J. Organomet. Chem., 2009, 694, 3050 CrossRef CAS;
(g) M. T. Lee, M. T. Chu, J. S. Lin and M. R. Tseng, J. Phys. D: Appl. Phys., 2010, 43, 442003 CrossRef;
(h) F. Lindla, M. Boesing, C. Zimmermann, F. Jessen, P. van Gemmern, D. Bertram, D. Keiper, N. Meyer, M. Heuken, H. Kalisch and R. H. Jansen, Appl. Phys. Lett., 2009, 95, 213305 CrossRef;
(i) J. H. Jou, Y. S. Wang, C. H. Lin, S. M. Shen, P. C. Chen, M. C. Tang, Y. Wei, F. Y. Tsai and C. T. Chen, J. Mater. Chem., 2011, 21, 12613 RSC;
(j) F. S. Steinbacher, R. Krause, A. Hunze and A. Winnacker, Org. Electron., 2011, 12, 911 CrossRef CAS;
(k) Q. S. Zhang, T. Komino, S. P. Huang, S. Matsunami, K. Goushi and C. Adachi, Adv. Funct. Mater., 2012, 22, 2327 CrossRef CAS;
(l) H. Sasabe, N. Toyota, H. Nakanishi, T. Ishizaka, Y. J. Pu and J. Kido, Adv. Mater., 2012, 24, 3212 CrossRef CAS PubMed;
(m) E. Orselli, J. Maunoury, D. Bascour and J. P. Catinat, Org. Electron., 2012, 13, 1506 CrossRef CAS.
-
(a) S. M. Pauley, Med. Hypotheses, 2004, 63, 588 CrossRef PubMed;
(b) G. C. Brainard, B. A. Richardson, T. S. King and R. J. Reiter, Brain Res., 1984, 294, 333 CrossRef CAS PubMed;
(c) S. W. Lockley, G. C. Brainard and C. A. Czeisler, J. Clin. Endocrinol. Metab., 2003, 88, 4502 CrossRef CAS PubMed.
-
(a) S. Heun and P. M. Borsenberger, Chem. Phys., 1995, 200, 245 CrossRef CAS;
(b) H. H. Fong, K. C. Lun and S. K. So, Chem. Phys. Lett., 2002, 353, 407 CrossRef CAS.
-
(a) Y. C. Zhu, L. Zhou, H. Y. Li, Q. L. Xu, M. Y. Teng, Y. X. Zheng, J. L. Zuo, H. J. Zhang and X. Z. You, Adv. Mater., 2011, 23, 4041 CrossRef CAS PubMed;
(b) M. Y. Teng, S. Zhang, S. W. Jiang, X. Yang, C. Lin, Y. X. Zheng, L. Y. Wang, D. Wu, J. L. Zuo and X. Z. You, Appl. Phys. Lett., 2012, 100, 073303 CrossRef;
(c) Q. L. Xu, C. C. Wang, T. Y. Li, M. Y. Teng, S. Zhang, Y. M. Jing, X. Yang, W. N. Li, C. Lin, Y. X. Zheng, J. L. Zuo and X. Z. You, Inorg. Chem., 2013, 52, 4916 CrossRef CAS PubMed;
(d) H. Y. Li, L. Zhou, M. Y. Teng, Q. L. Xu, C. Lin, Y. X. Zheng, J. L. Zuo, H. J. Zhang and X. Z. You, J. Mater. Chem. C, 2013, 1, 560 RSC;
(e) C. C. Wang, Y. M. Jing, T. Y. Li, Q. L. Xu, S. Zhang, W. N. Li, Y. X. Zheng, J. L. Zuo, X. Z. You and X. Q. Wang, Eur. J. Inorg. Chem., 2013, 5683 CrossRef CAS;
(f) M. Y. Teng, S. Zhang, Y. M. Jin, T. Y. Li, X. Liu, Q. L. Xu, C. Lin, Y. X. Zheng, L. Wang and J. L. Zuo, Dyes Pigm., 2014, 10, 105 CrossRef;
(g) Q. L. Xu, X. Liang, S. Zhang, Y. M. Jing, X. Liu, G. Z. Lu, Y. X. Zheng and J. L. Zuo, J. Mater. Chem. C, 2015, 3, 3694 RSC.
-
(a) H.-H. Chou and C.-H. Cheng, Adv. Mater., 2010, 22, 2468 CrossRef CAS PubMed;
(b) S. O. Jeon, S. E. Jang, H. S. Son and J. Y. Lee, Adv. Mater., 2011, 23, 1436 CrossRef CAS PubMed.
- Y. T. Tao, C. L. Yang and J. G. Qin, Chem. Soc. Rev., 2011, 40, 2943 RSC.
- S. Bettington, M. Tavasli, M. R. Bryce, A. Beeby, H. Al-Attar and A. P. Monkman, Chem. – Eur. J., 2007, 13, 1423 CrossRef CAS PubMed.
-
(a)
M. J. Frisch, G. W. Trucks, H. B. Schlegel, G. E. Scuseria, M. A. Robb, J. R. Cheeseman, G. Scalmani, V. Barone, B. Mennucci, G. A. Petersson, H. Nakatsuji, M. Caricato, X. Li, H. P. Hratchian, A. F. Izmaylov, J. Bloino, G. Zheng, J. L. Sonnenberg, M. Hada, M. Ehara, K. Toyota, R. Fukuda, J. Hasegawa, M. Ishida, T. Nakajima, Y. Honda, O. Kitao, H. Nakai, T. Vreven, J. A. Montgomery Jr., J. E. Peralta, F. Ogliaro, M. Bearpark, J. J. Heyd, E. Brothers, K. N. Kudin, V. N. Staroverov, R. Kobayashi, J. Normand, K. Raghavachari, A. Rendell, J. C. Burant, S. S. Iyengar, J. Tomasi, M. Cossi, N. Rega, J. M. Millam, M. Klene,J. E. Knox, J. B. Cross, V. Bakken, C. Adamo, J. Jaramillo, R. Gomperts, R. E. Stratmann, O. Yazyev, A. J. Austin, R. Cammi, C. Pomelli, J. W. Ochterski, R. L. Martin, K. Morokuma, V. G. Zakrzewski, G. A. Voth, P. Salvador, J. J. Dannenberg, S. Dapprich, A. D. Daniels, O. Farkas, J. B. Foresman, J. V. Ortiz, J. Cioslowski and D. J. Fox, Gaussian 09, Revision A.01, Gaussian, Inc., Wallingford, CT, 2009 Search PubMed;
(b) C. T. Lee, W. T. Yang and R. G. Parr, Phys. Rev. B: Condens. Matter Mater. Phys., 1988, 37, 785 CrossRef CAS;
(c) A. D. Becke, J. Chem. Phys., 1993, 98, 5648 CrossRef CAS;
(d) P. J. Hay, J. Phys. Chem. A, 2002, 106, 1634 CrossRef CAS;
(e) S. Chiodo, N. Russo and E. Sicilia, J. Chem. Phys., 2006, 125, 104107 CrossRef CAS PubMed;
(f) M. Cossi, N. Rega, G. Scalmani and V. Barone, J. Comput. Chem., 2003, 24, 669 CrossRef CAS PubMed.
- A. F. Rausch, M. E. Thompson and H. Yersin, Inorg. Chem., 2009, 48, 1928 CrossRef CAS PubMed.
-
(a) A. P. Wilde and R. J. Watts, J. Phys. Chem., 1991, 95, 622 CrossRef CAS;
(b) M. G. Colombo, T. C. Brunold, T. Riedener, H. U. Gudel, M. Fortsch and H. B. Burgi, Inorg. Chem., 1994, 33, 545 CrossRef CAS.
-
(a) J. Kalinowski, W. Stampor, J. Mezyk, M. Cocchi, D. Virgili, V. Fattori and P. Di Marco, Phys. Rev. B: Condens. Matter Mater. Phys., 2002, 66, 235321 CrossRef;
(b) W. S. Jeon, T. J. Park, S. Y. Kim, R. Pode, J. Jang and J. H. Kwon, Appl. Phys. Lett., 2008, 93, 063303 CrossRef.
- S. C. Tse, H. H. Fong and S. K. So, J. Appl. Phys., 2003, 94, 2033 CrossRef CAS.
-
(a) H. Scher and E. W. Montroll, Phys. Rev. B: Solid State, 1975, 12, 2455 CrossRef CAS;
(b) A. J. Pal, R. Osterbacka, K. M. Kallman and H. Stubb, Appl. Phys. Lett., 1997, 71, 228 CrossRef CAS.
- J. Lee, N. Chopra, S.-H. Eom, Y. Zheng, J. Xue, F. So and J. Shi, Appl. Phys. Lett., 2008, 93, 123306 CrossRef.
- S.-J. Su, T. Chiba, T. Takeda and J. Kido, Adv. Mater., 2008, 20, 2125 CrossRef CAS.
- M. Ikai, S. Tokito, Y. Sakamoto, T. Suzuki and Y. Taga, Appl. Phys. Lett., 2001, 79, 156 CrossRef CAS.
Footnote |
† Electronic supplementary information (ESI) available: Parameters associated with the single crystal diffraction data, selected bond lengths and angles, the cyclic voltammogram curves, the Contour plots, the TGA curves of Ir(tfmphiq)2(tpip) and Ir(tfmphqz)2(tpip). The transient EL signals for the device structure of ITO/TAPC (50 nm)/Ir complexes (60 nm) under different applied fields of Ir(tfmphiq)2(tpip) and Ir(tfmphqz)2(tpip). The external quantum efficiency–luminance curves of G1 and G2. The general measurements of the complexes and devices. CCDC 1582727. For ESI and crystallographic data in CIF or other electronic format see DOI: 10.1039/c8qm00060c |
|
This journal is © the Partner Organisations 2018 |
Click here to see how this site uses Cookies. View our privacy policy here.