DOI:
10.1039/C8QM00064F
(Research Article)
Mater. Chem. Front., 2018,
2, 1017-1023
An efficient yellow thermally activated delayed fluorescence emitter with universal applications in both doped and non-doped organic light-emitting diodes†
Received
7th February 2018
, Accepted 12th March 2018
First published on 13th March 2018
Abstract
Thermally activated delayed fluorescence (TADF) emitters have aroused serious attention for their use in organic light-emitting diodes (OLEDs). However, TADF emitters providing both doped and non-doped OLEDs with high device performance are rare. In this work, we present a yellow TADF emitter (OPDPO) meeting the above requirements. The OPDPO-based doped OLEDs show a maximum external quantum efficiency (EQE) of 26.7%, which is amongst the highest ever reported for doped yellow TADF-based OLEDs. The EQE remains as high as 22.1% at a luminance of 1000 cd m−2. Meanwhile, the OPDPO-based non-doped OLEDs afford a maximum EQE of 16.6% at a high luminance of 3600 cd m−2, which is amongst the best performances of non-doped yellow TADF-based OLEDs. Moreover, simple and efficient white OLEDs are implemented by combining a blue conventional fluorescent emitter with either a doped or non-doped OPDPO emissive layer, confirming the universal applications of OPDPO in high-performance OLEDs.
Introduction
Thermally activated delayed fluorescence (TADF)-based emissive materials can harvest both singlet (S1) and triplet (T1) excitons for light emission by virtue of the T1 → S1 up-conversion process, leading to a high exciton utilization efficiency of 100%, similar to phosphorescent materials.1,2 Therefore, intensive research has been carried out regarding the design and synthesis of full-color TADF materials.3 Amongst the different colors, yellow TADF-based materials are essential because they can be used with blue emissive materials for the fabrication of complementary white organic light-emitting diodes (WOLEDs). Generally, the triplet excitons in TADF materials tend to trigger triplet–triplet and singlet–triplet annihilations, causing notorious efficiency roll-off at high current or luminance.4,5 In practice, achieving high efficiency at high luminance is very important for advanced lighting and display applications. In this regard, TADF materials should satisfy the demands of high efficiency and low efficiency roll-off. Host–guest systems are commonly utilized by commercial manufacturers to improve device efficiency, and can efficiently prevent the concentration quenching effect that most TADF materials exhibit. Although a variety of yellow TADF materials have been developed,6–18 as listed in Table S1 and Fig. S1 (ESI†), challenges still remain and there are very limited examples of yellow TADF materials that enable efficient electroluminescence (EL) performance (external quantum efficiency (EQE) above 20% at a luminance of 1000 cd m−2). For example, Wong et al.12 reported a yellow TADF material exhibiting a maximum EQE of 29.2% and an efficiency roll-off of 30% at 1000 cd m−2. On the other hand, suitable TADF materials providing high device efficiency for non-doped OLED systems have aroused considerable attention.
This is because it helps to simplify the OLED device structure and fabrication processes, which is critical for low cost products, such as devices used in fast-moving consumer goods. Therefore, TADF materials demonstrating excellent device performance in both doped and non-doped OLEDs would be more attractive, especially yellow-based TADF emissive materials due to their shortage. For example, Tang et al. have reported two yellow TADF materials that achieved maximum EQEs of 19.2%10/19.6%11 and 9.7%10/15.3%11 in doped and non-doped OLEDs, respectively, suggesting room for further efficiency improvement.11
With regards to yellow TADF materials, it should not only enable high-efficiency monochromatic OLEDs, but also high-performance WOLEDs. Many yellow TADF-based WOLEDs are fabricated by integration with blue TADF materials through layer-by-layer stacking of doped emissive material layers (EMLs),6–8,19,20 however, these device structures and fabrication processes are complex. Luckily, numerous blue fluorescence-based OLEDs that endow non-doped devices with high EQEs have been reported,21–24 particularly aggregation-induced emission (AIE)-active type materials that are ideally suitable for the non-doping strategy.25,26 In this regard, a device strategy adopting a blue AIE type material combined with a yellow TADF material was conceived to make simple and efficient WOLEDs.
Herein, a highly efficient yellow TADF emitter 4-((10H-phenothiazin-10-yl)phenyl)(4-(diphenylphosphoryl)phenyl)methanone (OPDPO, inset of Fig. 1a)27 containing a phenothiazine28 donor group, a benzophenone core10,11 and a diphenylphosphoryl29,30 unit acceptor group, was synthesized by our group and was explored for its applications in OLEDs. With the use of doping and non-doping techniques, the OPDPO-based yellow devices afford impressive device efficiencies and low efficiency roll-off, which are amongst the best results ever reported for yellow OLEDs relating to TADF emitters. Significantly, by combination of OPDPO and a non-doped blue AIE-based emitter, simple and efficient non-doped WOLEDs are also realized.
 |
| Fig. 1 (a) UV-vis absorption spectra of OPDPO in tetrahydrofuran solution (10−5 M) and in a solid-state film at room temperature. Inset: Chemical structure of OPDPO. (b) Thermogravimetric analysis (TGA) curve recorded under N2 at a heating rate of 20 °C min−1. Inset: Differential scanning calorimetry (DSC) curve measured under N2. (c) Steady-state and time-resolved PL spectra of a yellow TADF OPDPO film recorded at 11 K with and without 677 ns delay. | |
Experimental
Materials
The yellow TADF emitter OPDPO was synthesized according to our previous method,27 wherein the phenothiazine group acts as the donor and the central benzophenone core acts as an electron acceptor, enabling a charge transfer state with a broad emission spectrum to improve the color rendering index (CRI) of two-color WOLEDs. The blue AIE fluorophore 9,9′-((2-(4′-(9H-carbazol-9-yl)-[1,1′-biphenyl]-4-yl)ethene-1,1-diyl)bis(4,1-phenylene))bis(9H-carbazole) (2CzTPEPCz) was also synthesized in our laboratory (see ESI†). PEDOT:PSS, 4,4′-bis(9H-carbazol-9-yl)biphenyl (CBP), m-bis(N-carbazolyl)benzene (mCP), bis(2-(diphenylphosphino)phenyl)ether oxide (DPEPO) and 1,3,5-tris(N-phenylbenzimidazol-2-yl)benzene (TPBI) were purchased from Xi’an Polymer Light Technology Co. Ltd (Xi’an, Shanxi province, China) and used without any further purification.
Device fabrication and characterization
All the devices were fabricated on clean ITO-coated glass substrates, which were treated by oxygen plasma for 15 minutes. Firstly, PEDOT:PSS was spin-coated on the ITO substrates at a speed of 2000 rpm for 1 minute, followed by thermal annealing for 15 minutes at 200 °C under an ambient environment. Then, the organic layers and alloy metals were sequentially deposited without breaking the vacuum, whilst keeping the pressure in the order of magnitude of 10−4 and 10−3 Pa, respectively, resulting in an active area of 2 × 10 mm2. Film thickness and deposition rates were monitored in situ by oscillating quartz thickness monitors. The current–voltage–luminance characteristics, Commission Internationale de l’Eclairage (CIE) coordinates, EL spectra and CRI of the unencapsulated devices were measured using a Keithley 2400 source combined with a Photo Research PR735 spectrometer at room temperature, whilst the EQEs were calculated using a computer program based on a previously reported theory.31 Photoluminescence (PL) and UV-vis absorption spectra were measured using a Shimadzu RF-5301PC spectrometer and a Hitachi U-3900 spectrophotometer, respectively. Time-resolved PL spectra of yellow TADF OPDPO film samples were investigated under vacuum conditions using a Horiba Scientific Fluorolog-3 spectrofluorometer equipped with a Cryocon 22C temperature controller at 11 K.
Results and discussion
Photo-physical and thermal properties
The UV-vis absorption spectra of OPDPO in solution and in the solid film-state are depicted in Fig. 1a. It can be observed that OPDPO in solution displays an absorption peak at 348 nm that is related to the intramolecular charge transfer (ICT) transition,32 whilst the absorption peak is red-shifted to 446 nm for OPDPO in the film-state. Time-resolved phosphorescence spectra of OPDPO films were measured at 11 K to determine their excited S1 and T1 states (Fig. 1c). The spectra show negligible changes with and without 677 ns delay, leading to a value of 2.61 and 2.59 eV for S1 and T1 of OPDPO, respectively, and a small energy difference (ΔEST = 0.02 eV) that is typical for efficient TADF materials. The highest occupied molecular orbital (HOMO) energy level was measured by ultraviolet photoemission spectroscopy (UPS, Fig. S2, ESI†), and calculated to be 5.5 eV. As a bandgap (Eg) of 2.3 eV was estimated from the onset of the UV-vis absorption spectrum of the OPDPO film, the lowest unoccupied molecular orbital (LUMO) energy level was determined by subtracting Eg from the HOMO level, resulting in a value of 3.2 eV. Thermal properties were investigated by thermogravimetric analysis (TGA) and differential scanning calorimetry (DSC) measurements. As shown in Fig. 1b, OPDPO is thermally stable with a high decomposition temperature (Td) of 428 °C (corresponding to 5% weight loss), along with a glass transition temperature (Tg) of 90.9 °C (Tg for 2CzTPEPCz is 162.5 °C). Thermostable materials are beneficial for good device operational stability. Table 1 summarizes the thermal and photo-physical properties of OPDPO.
Table 1 Thermal and photo-physical properties of yellow TADF OPDPO
T
d
(°C) |
T
g
(°C) |
E
g
(eV) |
S1d (eV) |
T1d (eV) |
HOMOe (eV) |
LUMOf (eV) |
UV |
PL |
Solutiong (nm) |
Filmh (nm) |
Solutiong (nm) |
Filmh (nm) |
Thermal decomposition at 5% weight loss temperature measured by thermogravimetric analysis.
Glass-transition temperature measured by differential scanning calorimetry.
Energy bandgap measured from the UV-vis absorption spectrum of the solid-state film.
Singlet and triplet energy measured by time-resolved phosphorescence spectra of the solid-state film at 11 K.
Measured by ultraviolet photoemission spectroscopy.
Calculated from LUMO = HOMO + Eg.
Measured from tetrahydrofuran solution with a concentration of 10−5 M.
Measured from the solid-state film.
|
428 |
90.9 |
2.3 |
2.61 |
2.59 |
−5.5 |
−3.2 |
348 |
446 |
564 |
589 |
Device performance of doped yellow OLEDs
The OPDPO-based doped yellow OLEDs were fabricated with a device configuration as: ITO/PEDOT:PSS(40 nm)/CBP(20 nm)/CBP:OPDPO(X wt%, 15 nm)/TPBI(40 nm)/Mg:Ag, and optimized by changing the doping ratios of OPDPO, X = 5 wt% (device A), X = 10 wt% (device B), and X = 20 wt% (device C). Fig. 2a illustrates the current density–voltage–luminance curves of the devices. For the three devices, very low current density is observed at low voltages, indicating small leakage current. Meanwhile, the current density shows an increment tendency on increasing the doping ratio of OPDPO, implying that OPDPO plays a role in facilitating charge-carrier transport. The luminance of the devices is obviously enhanced on increasing the doping ratio from 5 wt% in device A to 10 wt% in device B, wherein a maximum luminance of 32
590 cd m−2 at a voltage of 10 V is obtained, while the luminance starts to decrease in device C with a higher doping ratio (20 wt%). Fig. 2b and c describe the current efficiency (CE)–power efficiency (PE)–luminance and EQE–luminance curves of the devices, respectively, and the device performance is summarized in Table 2. The device efficiencies are first improved as the doping ratio increases from 5 wt% to 10 wt%, and then decreased when the doping ratio reaches up to 20 wt%. As a result, device B with CBP:10 wt% OPDPO EML presents the best device performance, including maximum CE, PE and EQE of 73.1 cd A−1, 38.2 lm W−1 and 26.7%, respectively. These high efficiency values of device B are amongst the best performance of state-of-the-art yellow TADF-OLEDs reported thus far. As for the previous yellow OLEDs listed in Table S1 (ESI†), they suffer from either high efficiency roll-off (≥30%) or low EL efficiency (EQE < 20%). Remarkably, besides high efficiency, device B exhibits low efficiency roll-off. For example, at a luminance of 1000 cd m−2, the EQE maintains as high as 21.8%, indicating an 18% decrement of the efficiency. The suppressed efficiency roll-off might be due to the relatively short triplet exciton lifetime of OPDPO, which can also be supported by the fact that OPDPO has a small energy difference (0.02 eV) between the singlet (S1 = 2.61 eV) and triplet (T1 = 2.59 eV) excited states, which benefits the reverse inter-system crossing (RISC) process.33 The exciting EL performance of device B paves the way for its application in commercial OLEDs, expecting bright prospects of applying OPDPO in OLEDs. Fig. 2d depicts the normalized EL spectra of the devices driven at a voltage of 8 V. All the devices are yellow emissive without the presence of CBP emission, inferring complete energy transfer from the CBP host to the OPDPO guest. With increasing the doping ratios of OPDPO, the EL spectral peak obviously red-shifts from 542 nm (device A), to 552 nm (device B), and to 566 nm (device C), revealing some sensitivity of the EL spectra to the doping ratios. In addition, the EL spectra exhibit a wide full-wavelength at half maximum (FWHM) of ∼128 nm, which is beneficial for improving the CRI of two-color WOLEDs when combined with blue emitters. Although the photoluminescence quantum yield (PLQY) of the OPDPO powder was measured to be only a moderate 37%, the EQEs of the OPDPO devices are very high. This infers that the triplet excitons are the majority excitons electro-generated during the EL process and contribute to the radiative transition due to the fast RISC, resulting in enhanced device efficiency.
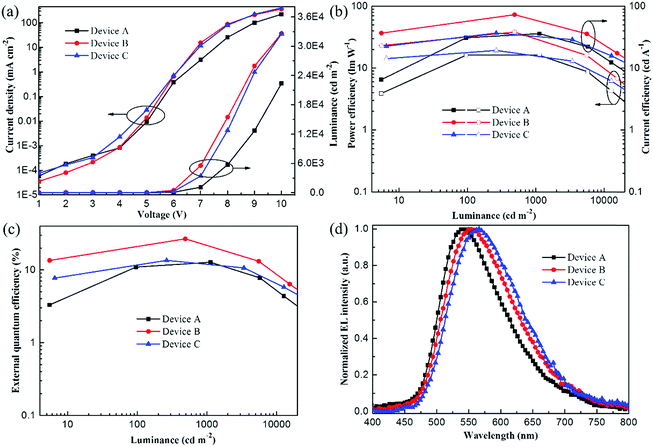 |
| Fig. 2 (a) Current density–voltage–luminance curves, (b) current efficiency–power efficiency–luminance curves, (c) EQE–luminance curves and (d) normalized EL spectra at 8 V for doped yellow OLEDs based on OPDPO (devices A, B and C). | |
Table 2 EL performance of the OLED devices
Device |
V
on
(V) |
Luminanceb (cd m−2) |
CE/PE/EQEb (cd A−1/lm W−1/%) |
CE/PE/EQEc (cd A−1/lm W−1/%) |
λ
EL
(nm) |
Voltage at a luminance of 1 cd m−2.
Measured at maximum value.
Measured at a luminance of 1000 cd m−2.
EL peak wavelength.
|
A |
5.0 |
22 400 |
36.1/16.2/12.7 |
35.9/16.4/12.6 |
542 |
B |
4.4 |
32 590 |
73.1/38.2/26.7 |
61.5/30.8/21.8 |
552 |
C |
4.2 |
32 530 |
36.9/19.3/13.5 |
32.6/16.2/12.0 |
566 |
D |
5.0 |
9460 |
15.6/7.6/6.8 |
10.6/4.8/4.6 |
586 |
E |
5.4 |
17 200 |
37.6/14.8/16.6 |
26.9/10.8/11.8 |
588 |
F |
5.6 |
18 460 |
29.0/10./12.8 |
26.5/8.9/11.7 |
588 |
W1 |
5.2 |
17 120 |
37.8/16.9/14.6 |
28.3/11.7/10.8 |
544 |
W2 |
6.0 |
15 130 |
24.0/9.4/10.7 |
22.4/8.9/9.8 |
586 |
Device performance of non-doped yellow OLEDs
Interestingly, it was found that OPDPO is strongly emissive in the solid film-state, therefore, this intrigued us to investigate the application of OPDPO in non-doped devices. Therefore, non-doped yellow OLEDs were investigated with a device configuration of: ITO/PEDOT:PSS(40 nm)/CBP(20 nm)/OPDPO(Y nm)/TPBI(40 nm)/Mg:Ag, and optimized by adjusting the thickness of the neat OPDPO EML, Y = 4 (device D), Y = 7 (device E), and Y = 10 (device F). Fig. 3a illustrates the current density–voltage–luminance curves of the non-doped devices. As the EML thickness increases from device D (4 nm) to device F (10 nm), the current density gradually decreases due to the increased total thickness of the device. Meanwhile, the maximum luminance enhances from 9460 (device D), to 17
200 (device E) and further reaches to 18
460 cd m−2 (device F), suggesting that more excitons are formed in the devices with a thicker OPDPO EML. Fig. 3b and c show the CE–PE–luminance curve and EQE–luminance curve of the devices, respectively. Compared to devices D and F, device E with a 7 nm OPDPO EML performs the best, achieving a maximum EQE of 16.6% at a high luminance of 3600 cd m−2, which is amongst the best performances of non-doped yellow-TADF OLEDs (9.2–18.4%).10,17,18 Meanwhile, the maximum EQEs of devices D and F are 6.8% and 12.8%, respectively. Fig. 3d shows the normalized EL spectra of the devices. It can be seen that the emission peak hardly changes when the thickness of the OPDPO EML increases, which is 586 nm for device D, and 588 nm for devices E and F. This implies that the EL spectra of these non-doped devices have negligible dependence on the thickness of the OPDPO EML, thus expanding the application of OPDPO as an efficient TADF emitter in non-doped OLED devices.
 |
| Fig. 3 (a) Current density–voltage–luminance curves, (b) current efficiency–power efficiency–luminance curves, (c) EQE–luminance curves and (d) normalized EL spectra at 8 V for non-doped yellow OLEDs based on OPDPO (devices D, E and F). | |
Device performance of white OLEDs
With respect to OPDPO, exploration of its applications in WOLEDs was conducted in order to further evaluate the material's OLED device performance. When yellow TADF emitters have been previously utilized to fabricate WOLEDs, they are usually combined with blue TADF emitters requiring doping technique systems, which leads to complicated multiple doped-EML structures.19,20 However, to the best of our knowledge, there has been no reported work concerning WOLEDs that has focussed on the employment of blue AIE emitters combined with yellow TADF emitters. AIE emitters can simplify device fabrication by virtue of non-doping techniques,34,35 along with the advantages of low efficiency roll-off when compared with blue TADF emitters, such as the widely utilized 9,9-dimethyl-9,10-dihydroacridine-diphenylsulphone (DMACDPS), which tends to induce high efficiency roll-off.25 Therefore, to enable simple and efficient WOLEDs, an AIE-active emitter 2CzTPEPCz (Fig. 4a) synthesized by our lab was chosen, which could be evidenced by the obviously enhanced PL intensity for its THF/water mixture solutions when the water fraction gradually increases (Fig. S3, ESI†).
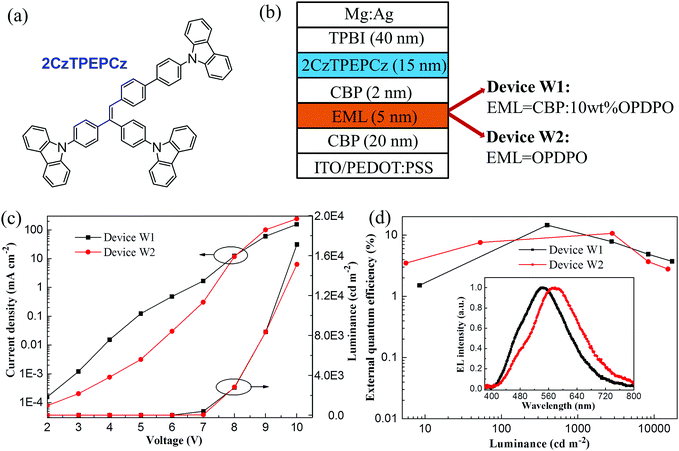 |
| Fig. 4 (a) Chemical structure of blue AIE 2CzTPEPCz. (b) Device configuration of WOLEDs (devices W1 and W2). (c) Current density–voltage–luminance and (d) EQE–luminance curves of devices W1 and W2. Inset of (d): normalized EL spectra of devices W1 and W2 at a voltage of 10 V. | |
Fig. 4b plots the device configuration of the two-color WOLEDs, including device W1 with a doped CBP:10 wt% OPDPO yellow EML and device W2 with a non-doped OPDPO yellow EML. Fig. 4c illustrates the current density–luminance–voltage curves of the WOLEDs. It is observed that device W1 exhibits higher current density compared to device W2 at low voltages, whilst device W2 presents higher current density at voltages beyond 8 V. With regards to the luminance behavior, device W1 exhibits higher luminance, especially at high voltages, such as a luminance of 17
120 cd m−2 for device W1 and 15
130 cd m−2 for device W2. Fig. 4d displays the EQE–luminance characteristics of the devices. Device W1 achieves a maximum EQE of 14.6%, which is amongst the best compared to the already reported WOLEDs from the literature (7.5–16.0%) based on conventional fluorescent and TADF hybrid emitters.36–38 Device W2 obtains a maximum EQE of 10.7%, which is still much higher than that of previous fluorescent WOLEDs fabricated with double non-doped EMLs.39–41 To investigate the role of the blue emitter in device performance, a 2CzTPEPCz-based blue OLED (device B1) was also constructed and the device performance is demonstrated in Fig. S4 (ESI†). Device B1 achieves a maximum EQE of 5.6% with low efficiency roll-off, which is amongst the best EQE values for non-doped OLEDs regarding blue AIE emitters, and the EL spectrum shows a peak at 480 nm. Therefore, the excellent EL performance of 2CzTPEPCz plays an important role in the high device efficiency of the WOLED devices. The inset of Fig. 4d plots the normalized EL spectra of the WOLEDs driven at a voltage of 10 V. Both devices W1 and W2 present broad EL spectra, which are associated with the broad FWHM of OPDPO and small wavelength difference between the EL peak of 2CzTPEPCz and OPDPO. It is noteworthy that the EL peak of OPDPO is located at 544 nm in device W1 and red-shifted to 586 nm in device W2, which is in accordance with the red-shifting tendency of the EL peak from the previous doped yellow devices (devices A–C) to the non-doped ones (devices D–F). Due to stronger yellow emission than that of the blue emission shown in the EL spectra, devices W1 and W2 give off warm white-light emission, leading to CIE coordinates of (0.34, 0.44) and CRI of 70 for device W1, and CIE coordinates of (0.46, 0.46) and CRI of 73 for device W2.
Conclusions
In summary, we have demonstrated highly efficient OLEDs with low efficiency roll-off and universal applications regarding doped and non-doped type OLED devices by virtue of a yellow TADF emitter (OPDPO). The OPDPO-based doped OLEDs afforded state-of-the-art OLED performances, such as a maximum EQE of 26.7%, which remained as high as 22.1% even at a luminance of 1000 cd m−2, by dispersing OPDPO into a common host material. Meanwhile, OPDPO endowed its non-doped OLEDs with a maximum EQE of 16.6% at a high luminance of 3600 cd m−2, in which such high values are rarely reported for non-doped yellow-TADF OLEDs. When OPDPO was further combined with a blue AIE emitter (2CzTPEPCz), simple and efficient WOLEDs were successfully fabricated, supporting the effectiveness of the proposed TADF and AIE fluorescent hybrid device design strategy. The impressive EL performance of the OPDPO-based OLED devices highlights its bright prospects for applications in the area of optoelectronics.
Conflicts of interest
There are no conflicts to declare.
Acknowledgements
This work was financially supported by NSFC (51733010, 61605253, 21672267 and 51473185), Science and Technology Planning Project of Guangdong (2015B090913003 and 2015B090915003) and the Fundamental Research Funds for the Central Universities.
References
- H. Uoyama, K. Goushi, K. Shizu, H. Nomura and C. Adachi, Nature, 2012, 492, 234 CrossRef CAS PubMed.
- H. B. Zhao, Z. H. Wang, X. Y. Cai, K. K. Liu, Z. Z. He, X. Liu, Y. Cao and S.-J. Su, Mater. Chem. Front., 2017, 1, 2039 RSC.
- Z. Y. Yang, Z. Mao, Z. L. Xie, Y. Zhang, S. W. Liu, J. Zhao, J. R. Xu, Z. G. Chi and M. P. Aldred, Chem. Soc. Rev., 2017, 46, 915 RSC.
- C. Li, L. Duan, D. D. Zhang and Y. Qiu, ACS Appl. Mater. Interfaces, 2015, 7, 15154 CAS.
- J. J. Liao, Y. Li, Y. Yuan, S. H. Li, X. D. Zhu, S. Barlow, M.-K. Fung, Z.-Q. Jiang, S. R. Marder and L.-S. Liao, Mater. Chem. Front., 2018 10.1039/C7QM00605E.
- S. Y. Lee, T. Yasuda, Y. S. Yang, Q. S. Zhang and C. Adachi, Angew. Chem., Int. Ed., 2014, 53, 6402 CrossRef CAS PubMed.
- Y. C. Li, X. L. Li, D. J. Chen, X. Y. Cai, G. Z. Xie, Z. Z. He, Y.-C. Wu, A. Lien, Y. Cao and S.-J. Su, Adv. Funct. Mater., 2016, 26, 6904 CrossRef CAS.
- J. Li, D. X. Ding, Y. T. Tao, Y. Y. Wei, R. F. Chen, L. H. Xie, W. Huang and H. Xu, Adv. Mater., 2016, 28, 3122 CrossRef CAS PubMed.
- H. Wang, L. S. Xie, Q. Peng, L. Q. Meng, Y. Wang, Y. P. Yi and P. F. Wang, Adv. Mater., 2014, 26, 5198 CrossRef CAS PubMed.
- J. J. Guo, X.-L. Li, H. Nie, W. Luo, R. Hu, A. Qin, Z. Zhao, S.-J. Su and B. Z. Tang, Chem. Mater., 2017, 29, 3623 CrossRef CAS.
- J. Huang, H. Nie, J. J. Zeng, Z. Y. Zhuang, S. F. Gan, Y. J. Cai, J. J. Guo, S.-J. Su, Z. J. Zhao and B. Z. Tang, Angew. Chem., Int. Ed., 2017, 56, 12971 CrossRef CAS PubMed.
- K. C. Pan, S.-W. Li, Y.-Y. Ho, Y.-J. Shiu, W.-L. Tsai, M. Jiao, W.-K. Lee, C.-C. Wu, C.-L. Chung, T. Chatterjee, Y.-S. Li, K.-T. Wong, H.-C. Hu, C.-C. Chen and M.-T. Lee, Adv. Funct. Mater., 2016, 26, 7560 CrossRef CAS.
- Z. H. Wang, Y. C. Li, X. Y. Cai, D. C. Chen, G. Z. Xie, K. K. Liu, Y.-C. Wu, C.-C. Lo, A. Lien, Y. Cao and S.-J. Su, ACS Appl. Mater. Interfaces, 2016, 8, 8627 CAS.
- G. Z. Xie, X. L. Li, D. J. Chen, Z. H. Wang, X. Y. Cai, D. C. Chen, Y. C. Li, K. K. Liu, Y. Cao and S.-J. Su, Adv. Mater., 2016, 28, 181 CrossRef CAS PubMed.
- Q. S. Zhang, H. Kuwabara, W. J. Potscavage Jr., S. P. Huang, Y. Hatae, T. Shibata and C. Adachi, J. Am. Chem. Soc., 2014, 136, 18070 CrossRef CAS PubMed.
- Y. P. Xiang, S. L. Gong, Y. B. Zhao, X. J. Yin, J. J. Luo, K. L. Wu, Z.-H. Lu and C. L. Yang, J. Mater. Chem. C, 2016, 4, 9998 RSC.
- H. Tanaka, K. Shizu, H. Nakanotani and C. Adachi, Chem. Mater., 2013, 25, 3766 CrossRef CAS.
- K. Y. Sun, Y. B. Sun, T. Y. Huang, J. R. Luo, W. Jiang and Y. M. Sun, Org. Electron., 2017, 42, 123 CrossRef CAS.
- M. Zhang, K. Wang, C.-J. Zheng, W. Liu, H. Lin, S.-L. Tao and X.-H. Zhang, Org. Electron., 2017, 50, 466 CrossRef CAS.
- C. B. Duan, J. Li, C. M. Han, D. X. Ding, H. Yang, Y. Wei and H. Xu, Chem. Mater., 2016, 28, 5667 CrossRef CAS.
- J. Jayabharathi, A. Prabhakaran, V. Thanikachalam and M. Sundharesan, RSC Adv., 2016, 6, 62208 RSC.
- S. T. Zhang, L. Yao, Q. M. Peng, W. J. Li, Y. Y. Pan, R. Xiao, Y. Gao, C. Gu, Z. M. Wang, P. Lu, F. Li, S. J. Su, B. Yang and Y. G. Ma, Adv. Funct. Mater., 2015, 25, 1755 CrossRef CAS.
- Q. S. Zhang, D. Tsang, H. Kuwabara, Y. Hatae, B. Li, T. Takahashi, S. Y. Lee, T. Yasuda and C. Adachi, Adv. Mater., 2015, 27, 2096 CrossRef CAS PubMed.
- X. J. Zhan, Z. B. Wu, Y. X. Lin, Y. J. Xie, Q. Peng, Q. Q. Li, D. G. Ma and Z. Li, Chem. Sci., 2016, 7, 4355 RSC.
- L. Chen, G. W. Lin, H. R. Peng, S. Y. Ding, W. W. Luo, R. R. Hu, S. M. Chen, F. Huang, A. J. Qin, Z. J. Zhao and B. Z. Tang, Mater. Chem. Front., 2017, 1, 176 RSC.
- J. Huang, N. Sun, J. Yang, R. Tang, Q. Q. Li, D. Ma and Z. Li, Adv. Funct. Mater., 2014, 24, 7645 CrossRef CAS.
- Z. L. Xie, Q. Y. Huang, T. Yu, L. Y. Wang, Z. Mao, W. L. Li, Z. Yang, Y. Zhang, S. W. Liu, J. R. Xu, Z. G. Chi and M. P. Aldred, Adv. Funct. Mater., 2017, 27, 1703918 CrossRef.
- C. Poriel, J. Rault-Berthelot, S. Thiery, C. Quinton, O. Jeannin, U. Biapo, D. Tondelier and B. Geffroy, Chem. – Eur. J., 2016, 22, 17930 CrossRef CAS PubMed.
- J. Zhang, D. Ding, Y. Wei, F. Han and H. Xu, Chem. Sci., 2016, 7, 2870 RSC.
- J. Zhang, D. Ding, Y. Wei, F. Han, H. Xu and W. Huang, Adv. Mater., 2016, 28, 479 CrossRef CAS PubMed.
- S. R. Forrest, D. D. C. Bradley and M. E. Thompson, Adv. Mater., 2003, 15, 1043 CrossRef CAS.
- Z. L. Xie, C. C. Chen, S. D. Xu, J. Li, Y. Zhang, S. W. Liu, J. R. Xu and Z. G. Chi, Angew. Chem., Int. Ed., 2015, 54, 7181 CrossRef CAS PubMed.
- M. Inoue, T. Serevičius, H. Nakanotani, Y. Kou, T. Matsushima, S. Juršėnas and C. Adachi, Chem. Phys. Lett., 2016, 644, 62 CrossRef CAS.
- B. Q. Liu, H. Nie, X. B. Zhou, S. B. Hu, D. X. Luo, D. Y. Gao, J. H. Zou, M. Xu, L. Wang, Z. J. Zhao, A. J. Qin, J. B. Peng, H. L. Ning, Y. Cao and B. Z. Tang, Adv. Funct. Mater., 2016, 26, 776 CrossRef CAS.
- T. Zhang, J. Wang, T. Li, M. Liu, W. F. Xie, S. Y. Liu, D. L. Liu, C.-L. Wu and C.-T. Chen, J. Phys. Chem. C, 2010, 114, 4186 CAS.
- B. Zhao, T. Y. Zhang, W. L. Li, Z. S. Su, B. Chu, X. W. Yan, F. M. Jin, Y. Gao and H. R. Wu, Org. Electron., 2015, 23, 208 CrossRef CAS.
- X.-L. Li, G. Z. Xie, M. Liu, D. C. Chen, X. Y. Cai, J. B. Peng, Y. Cao and S.-J. Su, Adv. Mater., 2016, 28, 4614 CrossRef CAS PubMed.
- T. Higuchi, H. Nakanotani and C. Adachi, Adv. Mater., 2015, 27, 2019 CrossRef CAS PubMed.
- S. J. Liu, F. Li, Q. Diao and Y. G. Ma, Org. Electron., 2010, 11, 613 CrossRef CAS.
- S. M. Chen, Z. J. Zhao, B. Z. Tang and H. S. Kwok, J. Phys. D: Appl. Phys., 2010, 43, 095101 CrossRef.
- W. F. Xie, Z. J. Wu, S. Y. Liu and S. T. Lee, J. Phys. D: Appl. Phys., 2003, 36, 2331 CrossRef CAS.
Footnote |
† Electronic supplementary information (ESI) available. See DOI: 10.1039/c8qm00064f |
|
This journal is © the Partner Organisations 2018 |
Click here to see how this site uses Cookies. View our privacy policy here.