DOI:
10.1039/C8QM00190A
(Research Article)
Mater. Chem. Front., 2018,
2, 1383-1388
Near-infrared light-driven locomotion of a liquid crystal polymer trilayer actuator†
Received
25th April 2018
, Accepted 18th May 2018
First published on 18th May 2018
Abstract
A new and general design is demonstrated for a liquid crystal polymer network (LCN)-based actuator to perform near-infrared (NIR) light-guided locomotion. The actuator is a structured trilayer, comprising a thin reduced graphene oxide (RGO) top layer, an inactive polymer middle layer and an active LCN bottom layer. Exposing the RGO side to a moving NIR laser, a moving wave along the strip actuator is generated, which makes the strip an effective caterpillar walker whose light-driven locomotion has appealing attributes. On one hand, in contrast to most LCN-based actuators that move on a ratcheted substrate surface or need a supporting plastic frame, the trilayer actuator moves on either horizontal or inclined untreated surfaces. On the other hand, while known actuators using the photothermal effect are usually prepared by mixing the nanofiller used as a NIR light heater with the polymer, which may reduce the reversible deformation degree and increase the compatibility concerns, the easy trilayer fabrication method laminates directly a “sheet” of RGO on thick polymer layers, which circumvents the potential problems.
Introduction
In recent years, soft active materials have drawn a great deal of attention because of their envisioned applications in biomedicine,1,2 artificial muscles3,4 and soft robots.5–8 Unlike the rigid materials that need mechanical force to be applied to realize motion or deformation, soft active materials can move or deform under thermal,9–12 optical13–16 or electrical stimulation.17–19 Among them, photo-actuated liquid crystal polymer networks (LCNs, including liquid crystal elastomers) have emerged as a particularly promising material system. They can transform molecular level movements caused by photon energy absorption into macroscopic mechanical motions.20–27 A common strategy for designing photo-responsive LCNs is based on using azobenzene mesogens in the polymer whose reversible trans–cis photoisomerization generates a significant photomechanical effect.13,20–26,28–31 Besides the trans–cis photochemical reaction of azobenzene, the near-infrared (NIR) light induced photothermal effect has been the basis of another effective method for the actuation of photo-responsive LCNs related to LC-to-isotropic phase transition. Until now, various photothermal agents have been incorporated into LCN matrices for this purpose, such as carbon nanotubes (CNTs),27,32–37 gold nanorods (AuNRs)38–40 and graphene oxide (GO).41–43 They can transform optical energy into heating to induce the LC-to-isotropic phase transition that drives the LCN actuator to deform macroscopically. However, due to generally poor compatibility with the organic polymers, the concentrations of the added photothermal agents are limited, which can restraint the photothermal effect and weaken the photoactuation speeds of the LCN actuators. Directly incorporating a NIR dye into the polymer structure has been proposed to address this issue.44 In some of the above-mentioned LCN actuators, the light-triggered deformation was converted into locomotion (caterpillar or inchworm-like walking). Generally speaking, to achieve light-driven motions, the photodeformable LCN actuator was used as a hinge to build a device,27,45 or needs a supporting plastic frame,21 and the surface on which the actuator moves needs to be treated.23,27,46,47 Therefore, it is still challenging to fabricate LCN actuators such that a free piece can perform light-driven caterpillar-type motion on untreated surfaces.
Herein, we describe the design, preparation and investigation of an LCN-based trilayer actuator that can address the challenges. The design is depicted in Fig. 1A. The top photothermal thin layer is chosen to be reduced graphene oxide (RGO) considering its highly efficient photothermal conversion and thermal conductivity,48–50 and it is separated from the bottom LCN by an inactive polymer layer. The LCN has uniaxial orientation of mesogens, it contracts along the orientation direction upon LC-to-isotropic phase transition and it extends on cooling to the LC phase. If the RGO layer is directly connected to the LCN without the middle polymer, when it is exposed to NIR light, the photothermally induced heating on the LCN side in contact with RGO should bend the bilayer towards the light with concave deformation of the RGO layer. While by inserting the middle layer with appropriate thickness and mechanical strength, the RGO-released heat upon NIR light irradiation has to diffuse through the inactive polymer to heat the LCN side for LC-to-isotropic phase transition. With a soft LCN, the contraction should bend the trilayer actuator away from the light with convex deformation for the RGO layer with respect to the incident light. As will be demonstrated further on, the purpose of this design is to use NIR light to a generate moving wave along a strip of the actuator with RGO on top to capture the light. This design thus avoids the incorporation of RGO in the LCN matrix.
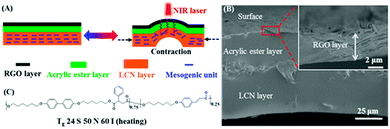 |
| Fig. 1 (A) Schematic illustration of the designed near-infrared (NIR) light-responsive trilayer actuator: a thin layer of reduced graphene oxide (RGO) on top, an inactive polymer middle layer and a liquid crystal network (LCN) layer at the bottom. The photothermally induced contraction of the LCN side on contact with the middle layer leads to bending of the actuator away from the NIR light with convex deformation of the RGO layer surface. (B) Cross-sectional SEM image of a trilayer actuator (the actuator surface is seen due to the imaging angle tilted by 30°). (C) Chemical structure and phase transition temperatures of the used main-chain liquid crystal polymer (Tg, glass transition; S: smectic phase; N: nematic phase; I: isotropic state). | |
Results and discussion
The scanning electron microscope (SEM) image in Fig. 1B shows the cross-section of a trilayer actuator investigated in this work, the fabrication of which is simple and convenient. The top RGO layer, prepared via vacuum filtration before cutting into a strip shape,51 has a thickness of about 4 μm, and the middle layer, about 30 μm thick, is an acrylic ester tape (double adhesive surfaces)52 that binds the RGO and the thickest LCN bottom layer (∼60 μm) firmly together without delamination. The LCN was prepared using a main-chain liquid crystal polymer (chemical structure in Fig. 1C) that was stretched at 55 °C (in the nematic phase) to 300% elongation to yield a monodomain of uniaxial LC orientation, followed by exposure to UV light (320–480 nm filter, 90 mW cm−2) at 47 °C on both sides for uniform photocrosslinking. The obtained LCN actuator displays large reversible contraction/extension (about 51%) along the strain direction upon heating to the isotropic state and cooling to the LC phase, respectively.11
The photoinduced bending behavior of the trilayer actuator was first investigated using an easy setup as depicted in Fig. 2A. The specimen was cut into a strip with dimensions of 15 mm × 2 mm × 94 μm, and one end of it was fixed. When NIR light was applied to the upper section of the strip, the photothermally induced LC-to-isotropic phase transition of the LCN can occur and result in deflection of the strip towards the light (due to light exposure from the LCN side). The observed fast bending of an actuator under NIR irradiation for 2 seconds and the subsequent recovery of the straight shape after turning off NIR light for 4 seconds are also shown in Fig. 2A. The defined bending angle at NIR light on/off could be measured from the snapshots of the recorded videos (Movie S1, ESI†). We prepared three trilayer actuators differing only in the thickness of the RGO layer, denoted as actuator-1 (∼1 μm thick for RGO), actuator-2 (∼3 μm) and actuator-3 (∼4 μm) (SEM images in Fig. S2, ESI†). Fig. 2B shows the plots of bending angle vs. time under NIR irradiation (980 nm, 1.87 W cm−2) and after turning off NIR for the three actuators. It appears that with a thicker RGO layer the activation is faster (reaching about 45° after 1.8 s irradiation for actuator-3), and the relaxation is quicker as well at the NIR off state. This is no surprise because a thicker RGO layer means greater photothermal effect that makes a larger portion of LCN undergo an LC-to-isotropic phase transition, resulting in larger photomechanical contraction/extension force for bending/unbending, respectively. Unless otherwise stated, actuator-3 was utilized for the other experiments. The effect of NIR light intensity on the photoinduced bending was also investigated. As shown in Fig. 2C, with increasing NIR light intensity, the increasing photothermal effect allowed a larger bending angle to be obtained after the same irradiation time of 2 seconds, up to 60° with the highest intensity tested (2.70 W cm−2). Fig. 2D shows 40 cycles of bending/unbending 2 s after NIR light exposure (1.87 W cm−2) and 4 s after NIR turning off, respectively. Since the durability is important for practical use of an actuator, we tested 500 cycles using actuator-3. The deformation remained almost unchanged and the actuator showed no sign of deterioration in performance (see Fig. S4, ESI†). This result means that the fast photoinduced deformation of the trilayer actuator is robust and reliable. The actual NIR light-induced heating of the LCN layer in the actuator was analyzed by measuring the temperatures at the back surface of the LCN and the inactive polymer/LCN interface (using RGO/polymer bilayer) using an infrared camera. The temperatures achieved after 2 s NIR irradiation at different light intensities are shown in Fig. 2E, indicating clearly the formation of a temperature gradient across the thickness of the LCN layer, down from the polymer/LCN interface to the back side of the LCN layer. The overall temperature of the LCN increases with increasing NIR light intensity, and is effective to induce the LC-to-isotropic phase transition required for the actuation. In control tests using RGO/polymer and polymer/LCN bilayers, no bending actuation was observed under the same NIR light irradiation conditions, further confirming the actuation mechanism of the trilayer actuator.
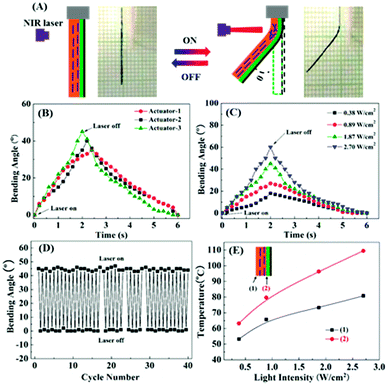 |
| Fig. 2 (A) Photos showing the fast bending/unbending of a trilayer strip with NIR light on/off respectively, while the schematic shows the experimental setup and defined bending angle. (B) Change in bending angle over time under NIR light (1.87 W cm−2) and after NIR turning off for three trilayer actuators with different thicknesses of the LCN layer (about 1, 3 and 4 μm for actuators 1, 2 and 3, respectively). (C) Change in bending angle over time for actuator-3 under NIR light of different intensities and after NIR turning off. (D) Repeated bending angle switch for 40 cycles with actuator-3 subjected to NIR light (1.87 W cm−2) for 2 seconds and after NIR turning off for 4 seconds. (E) Temperatures measured at the back surface of the LCN layer and at the inactive polymer/LCN interface (inset schematic) after 2 seconds of NIR light irradiation at different intensities, showing NIR light-induced heating of the LCN layer in the trilayer actuator. The dimensions of all actuator strips are 15 mm × 2 mm × 94 μm. | |
As described above, the bending behavior of the trilayer actuator under NIR light exposure is determined by an unbalanced contraction force across the thickness direction as a result of photothermally induced order-to-disorder phase transition in the LCN layer. This photomechanical response can be monitored by means of the isostrain testing method by applying NIR light to a strip-shaped sample held under constant strain in the sample holder of a tensile tester (Fig. S5, ESI†). Fig. 3 shows the contractile force generated by a trilayer actuator (15 mm × 2 mm × 94 μm) under 980 nm laser irradiation of different intensities. In all cases, when the laser is applied, a contractile force is generated quickly; and it basically drops to zero once the laser is turned off. It is visible that the actuation stress becomes larger with increasing the 980 nm laser intensity: reaching about 0.025, 0.06, 0.18 and 0.27 MPa for the light intensity of 0.38, 0.89, 1.87 and 2.70 W cm−2, respectively (the inset of Fig. 3 shows a plot using these data). The cycle of force up and down under NIR light on and off can be repeated many times; however, at relatively high laser intensities, the achievable force decreases slightly over time.
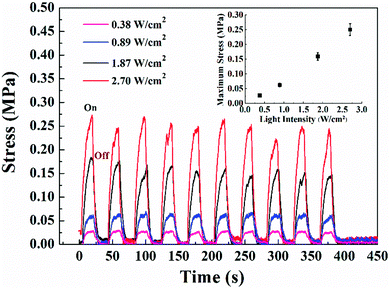 |
| Fig. 3 Photothermally induced actuation stress on an actuator-3 strip (15 mm × 2 mm × 94 μm) subjected to a number of cycles of on (15 s)–off (25 s) of 980 nm laser exposure of various intensities. The inset is a plot of maximum stress in the laser on state vs. laser intensity. | |
The fast NIR light triggered actuation was used to generate a moving wave along a strip of the trilayer actuator. In this case, the used strip has a large aspect ratio of 20 (dimension: 40 mm × 2 mm × 94 μm) to facilitate the observation. The two ends of the strip were fixed to the substrate (a fluorescent plate for viewing the NIR laser spot), at a separation distance of 35 mm. As shown in Fig. 4 by snapshots and illustrations, when the NIR laser is applied to the RGO top layer, the photothermally induced deformation generates a bump towards the light beam, and when the laser moves along the actuator surface, the bump follows. Guided by the laser movement, this wave can propagate from left to right (Fig. 4A, Movie S2, ESI†) or in the reverse direction (Fig. 4B, Movie S2, ESI†). This light-driven wave motion is robust. Within experimental uncertainty, the wave moving speeds in the two directions remain the same and constant (about 1.3 mm s−1) over 40 cycles of “going forward and backward”, indicating excellent NIR light-controlled mobility of our trilayer actuator (Fig. 4C).
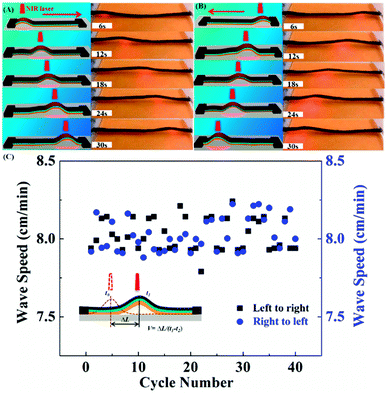 |
| Fig. 4 (A and B) Photos and schematics showing NIR light-guided wave propagation along a strip of the actuator whose two ends are fixed to the substrate: (A) moving forward (from left to right) and (B) moving backward (from right to left). (C) Wave moving speed (defined in the schematic) in the two directions obtained for 40 cycles of movement. NIR laser intensity: 1.87 W cm−2, dimensions of the actuator strip: 40 mm × 2 mm × 94 μm. | |
Polymer-based actuators or devices capable of caterpillar-like locomotion under stimulation have attracted much interest in recent years.21,23,27,45,46,53–62 The various types of materials reported in the literature include a chemically responsive polymer gel,55 a UV/visible light-activated LCN/polyethylene laminated bilayer,45 a humidity-responsive LCN film,60 NIR light-responsive devices using a CNT- LCN/silicone bilayer,27 a thermally activated poly(ethylene-co-vinyl acetate) (EVA) semicrystalline polymer,60 and an electro-responsive graphene/polydiacetylene (PDA) film.57 Here, we show that the fast and reversible NIR light-driven wave propagation of our trilayer actuator can be harnessed to fabricate an effective caterpillar walker on a smooth surface, using a free actuator strip and without any prior treatment of the substrate surface. Presented in Fig. 5 are photos and a schematic that show how the light-driven caterpillar locomotion works for a strip placed on the fluorescence plate. At the beginning, the laser spot is applied to the left side of the strip, causing it to arch up and forcing the left edge to bend inward. As the laser spot moves to right, the left part tends to recover to its original flat state. While the left edge experiences a larger frictional force against its motion to the left, the right part of the strip, where the laser spot moves to, arches up, which flattens the left section with the left edge acting as a stationary point. By repeating the laser movement from left to right, the propagating wave drives the strip to move toward the right direction. The NIR light-driven moving speed of the caterpillar walker is about 0.1 mm s−1 (Movie S3, ESI†), which is similar to what is known with UV and/or visible light-responsive LCN-base actuators,23,45,53 but much faster than electromechanical LCN (0.05 mm s−1)54 and chemically responsive polymer gels (0.003 mm s−1) on a flat surface.55
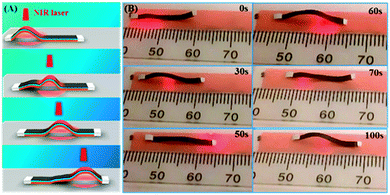 |
| Fig. 5 Schematic (A) and photos (B) showing the locomotion of a trilayer strip through a propagating wave generated by a NIR laser scan from left to right. NIR light intensity: 1.87 W cm−2, and strip dimensions: 20 mm × 2 mm × 94 μm. | |
More interestingly, our moving-wave walker can also crawl on a smooth slope, which is difficult to achieve without a treated surface and hinge-based device. As can be seen in Fig. 6, a strip was placed on a piece of a fluorescent plate set at a 15° incline. By moving the NIR laser upward along the actuator (1.87 W cm−2), the strip could climb the incline at an average speed of 0.06 mm s−1 (Movie S4, ESI†). Although the incline is relatively small, the result is significant. Indeed, an inchworm-type walker was shown to be able to climb a 50° incline under on/off cycles of IR light irradiation, which was a device fabricated with actuating hinges of CNT (SWNT)-loaded LCN/silicone bilayer and the inline surface was ratcheted.27 Our actuator is very easy to make, just putting the three layers together and cutting it into a strip shape, and it can crawl up the incline through the moving-wave mechanism with no need to ratchet the incline surface.
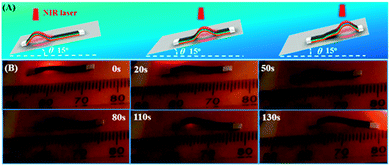 |
| Fig. 6 Schematic (A) and photos (B) showing a trilayer strip climbing a 15° incline through a moving wave generated by an upward NIR laser scan. NIR light intensity: 1.87 W cm−2, and strip dimensions: 20 mm × 2 mm × 94 μm. | |
Conclusions
We have demonstrated a facile and general approach to fabricating a NIR light-driven actuator capable of light-guided locomotion. The trilayer actuator has a thin RGO top layer, an inactive polymer middle layer and an LCN bottom layer, obtained by simply packing them together with the middle layer serving as a connection adhesive. By placing a strip of the actuator on a substrate, turning on a NIR laser on the RGO surface and moving it from one side to another can generate a moving wave along the strip, as a result of fast upward bending and downward unbending at the laser spot on (photothermally induced heating and LC-to-isotropic phase transition in the bottom LCN layer) and off state (cooling), respectively. We show that this light-driven moving wave makes the actuator strip an effective caterpillar-type walker to undergo locomotion, on both level or inclined surfaces, controlled by a directional laser scan on it. Our NIR light-driven actuator has the attributes of easy fabrication and capability of locomotion on untreated surfaces, which may be exploited for applications. To our knowledge, this is the first LCN-based actuator design with graphene paper (RGO or GO) directly laminated on polymer to harness the photothermal effect for light-guided locomotion.
Conflicts of interest
The authors herein declare no conflicts of interest.
Acknowledgements
Y. Z. acknowledges the financial support from the Natural Sciences and Engineering Research Council of Canada (NSERC) and le Fonds de recherche du Québec: Nature et technologies (FRQNT). L. L. D. thanks the China Scholarship Council (CSC) for awarding him a scholarship and the Innovation Project for College Graduates of Jiangsu Province (KYLX16_0783). M. C. thanks the National Natural Science Foundation of China (NNSFC) for financial support (51173072 and 21571084). H. Z. acknowledges the financial support from NNSFC (21504031) and the Natural Science Foundation of Jiangsu Province (BK20150135).
Notes and references
- H. Yuk, S. Lin, C. Ma, M. Takaffoli, N. X. Fang and X. H. Zhao, Nat. Commun., 2017, 8, 14230 CrossRef PubMed.
- K. Malachowski, M. Jamal, Q. R. Jin, B. Polat, C. J. Morris and D. H. Gracias, Nano Lett., 2014, 14, 4164 CrossRef PubMed.
- A. Miriyev, K. Stack and H. Lipson, Nat. Commun., 2017, 8, 596 CrossRef PubMed.
- S. M. Mirvakili and I. W. Hunter, Adv. Mater., 2018, 30, 1704407 CrossRef PubMed.
- M. Wehner, R. L. Truby, D. J. Fitzgerald, B. Mosadegh, G. M. Whitesides, J. A. Lewis and R. J. Wood, Nature, 2016, 536, 451 CrossRef PubMed.
- H. W. Huang, M. S. Sakar, A. J. Petruska, S. Pané and B. J. Nelson, Nat. Commun., 2016, 7, 12263 CrossRef PubMed.
- H. Zeng, P. Wasylczyk, D. S. Wiersma and A. Priimagi, Adv. Mater., 2017 DOI:10.1002/adma.201703554.
- W. Q. Hu, G. Z. Lum, M. Mastrangeli and M. Sitti, Nature, 2018, 554, 81 CrossRef PubMed.
- K. Yu, Q. Ge and H. J. Qi, Nat. Commun., 2014, 5, 3066 CrossRef PubMed.
- T. Xie, Nature, 2010, 464, 267 CrossRef PubMed.
- R. Yang and Y. Zhao, Angew. Chem., Int. Ed., 2017, 56, 14202 CrossRef PubMed.
- V. Stroganov, S. Zakharchenko, E. Sperling, A. K. Meyer, O. G. Schmidt and L. Ionov, Adv. Funct. Mater., 2014, 24, 4357 CrossRef.
- J. A. Lv, Y. Y. Liu, J. Wei, E. Q. Chen, L. Qin and Y. L. Yu, Nature, 2016, 537, 179 CrossRef PubMed.
- X. Zhang, Z. Yu, C. Wang, D. Zarrouk, J. W. T. Seo, J. C. Cheng, A. D. Buchan, K. Takei, Y. Zhao, J. W. Ager, J. Zhang, M. Hettick, M. C. Hersam, A. P. Pisano, R. S. Fearing and A. Javey, Nat. Commun., 2014, 5, 2983 CrossRef PubMed.
- H. W. Zhou, C. G. Xue, P. Weis, Y. Suzuki, S. L. Huang, K. Koynov, G. K. Auernhammer, R. Berger, H. J. Butt and S. Wu, Nat. Chem., 2017, 9, 145 CrossRef PubMed.
- T. Seki, J. Mater. Chem. C, 2016, 4, 7895 RSC.
- S. Taccola, F. Greco, E. Sinibaldi, A. Mondini, B. Mazzolai and V. Mattoli, Adv. Mater., 2015, 27, 1668 CrossRef PubMed.
- G. H. Kwon, J. Y. Park, J. Y. Kim, M. L. Frisk, D. J. Beebe and S. H. Lee, Small, 2008, 4, 2148 CrossRef PubMed.
- L. Hines, K. Petersen and M. Sitti, Adv. Mater., 2016, 28, 3690 CrossRef PubMed.
- A. H. Gelebart, G. Vantomme, E. W. Meijer and D. J. Broer, Adv. Mater., 2017, 29, 1606712 CrossRef PubMed.
- A. H. Gelebart, D. J. Mulder, M. Varga, A. Konya, G. Vantomme, E. W. Meijer, R. L. B. Selinger and D. J. Broer, Nature, 2017, 546, 632 CrossRef PubMed.
- X. L. Lu, S. W. Guo, X. Tong, H. S. Xia and Y. Zhao, Adv. Mater., 2017, 29, 1606467 CrossRef PubMed.
- H. Zeng, O. M. Wani, P. Wasylczyk and A. Priimagi, Macromol. Rapid Commun., 2018, 39, 1700224 CrossRef PubMed.
- M. Yamada, M. Kondo, J. Mamiya, Y. Yu, M. Kinoshita, C. J. Barrett and T. Ikeda, Angew. Chem., Int. Ed., 2008, 47, 4986 CrossRef PubMed.
- S. Iamsaard, S. J. Aßhoff, B. Matt, T. Kudernac, J. J. L. M. Cornelissen, S. P. Fletcher and N. Katsonis, Nat. Chem., 2014, 6, 229 CrossRef PubMed.
- H. K. Bisoyi and Q. Li, Chem. Rev., 2016, 116, 15089 CrossRef PubMed.
- R. R. Kohlmeyer and J. Chen, Angew. Chem., Int. Ed., 2013, 52, 9234 CrossRef PubMed.
- H. F. Yu and T. Ikeda, Adv. Mater., 2011, 23, 2149 CrossRef PubMed.
- D. D. Han, Y. L. Zhang, J. N. Ma, Y. Q. Liu, B. Han and H. B. Sun, Adv. Mater., 2016, 28, 8328 CrossRef PubMed.
- C. Huang, J. Lv, X. Tian, Y. Wang, Y. Yu and J. Liu, Sci. Rep., 2015, 5, 17414 CrossRef PubMed.
- H. Zeng, O. M. Wani, P. Wasylczyk, R. Kaczmarek and A. Priimagi, Adv. Mater., 2017, 29, 1701814 CrossRef PubMed.
- Y. Yang, Z. Pei, Z. Li, Y. Wei and Y. Ji, J. Am. Chem. Soc., 2016, 138, 2118 CrossRef PubMed.
- M. Wang, S. M. Sayed, L. X. Guo, B. P. Lin, X. Q. Zhang, Y. Sun and H. Yang, Macromolecules, 2016, 49, 663 CrossRef.
- J. E. Marshall, Y. Ji, N. Torras, K. Zinoviev and E. M. Terentjev, Soft Matter, 2012, 8, 1570 RSC.
- Y. Ji, Y. Y. Huang, R. Rungsawang and E. M. Terentjev, Adv. Mater., 2010, 22, 3436 CrossRef PubMed.
- C. S. Li, Y. Liu, X. Z. Huang and H. R. Jiang, Adv. Funct. Mater., 2012, 22, 5166 CrossRef.
- L. Q. Yang, K. Setyowati, A. Li, S. Q. Gong and J. Chen, Adv. Mater., 2008, 20, 2271 CrossRef.
- X. Y. Liu, R. B. Wei, P. T. Hoang, X. G. Wang, T. Liu and P. Keller, Adv. Funct. Mater., 2015, 25, 3022 CrossRef.
- A. W. Hauser, D. Q. Liu, K. C. Bryson, R. C. Hayward and D. J. Broer, Macromolecules, 2016, 49, 1575 CrossRef.
- K. G. G. Cuevas, L. Wang, C. M. Xue, G. Singh, S. Kumar, A. Urbas and Q. Li, Chem. Commun., 2015, 51, 9845 RSC.
- Y. K. Yang, W. J. Zhan, R. G. Peng, C. G. He, X. C. Pang, D. Shi, T. Jiang and Z. Q. Lin, Adv. Mater., 2015, 27, 6376 CrossRef PubMed.
- R. B. Wei, Z. C. Wang, H. X. Zhang and X. B. Liu, Liq. Cryst., 2016, 43, 1009 CrossRef.
- C. S. Li, Y. Liu, X. Z. Huang, C. H. Li and H. R. Jiang, Mol. Cryst. Liq. Cryst., 2015, 616, 83 CrossRef.
- L. Liu, M. H. Liu, L. L. Deng, B. P. Lin and H. Yang, J. Am. Chem. Soc., 2017, 139, 11333 CrossRef PubMed.
- M. Yamada, M. Kondo, R. Miyasato, Y. Naka, J. Mamiya, M. Kinoshita, A. Shishido, Y. L. Yu, C. J. Barrett and T. Ikeda, J. Mater. Chem., 2009, 19, 60 RSC.
- H. Zeng, P. Wasylczyk, C. Parmeggiani, D. Martella, M. Burresi and D. S. Wiersma, Adv. Mater., 2015, 27, 3883 CrossRef PubMed.
- X. Y. Liu, S. K. Kim and X. G. Wang, J. Mater. Chem. B, 2016, 4, 7293 RSC.
- Z. X. Cheng, T. J. Wang, X. Li, Y. H. Zhang and H. F. Yu, ACS Appl. Mater. Interfaces, 2015, 7, 27494 Search PubMed.
- T. Wang, J. H. Huang, Y. Q. Yang, E. Z. Zhang, W. X. Sun and Z. Tong, ACS Appl. Mater. Interfaces, 2015, 7, 23423 Search PubMed.
- Y. Yang, Y. Tan, X. L. Wang, W. L. An, S. M. Xu, W. Liao and Y. Z. Wang, ACS Appl. Mater. Interfaces, 2018, 10, 7688 Search PubMed.
- D. Li, M. B. Müller, S. Gilje, R. B. Kaner and G. G. Wallace, Nat. Nanotechnol., 2008, 3, 101 CrossRef PubMed.
- L. Z. Chen, M. C. Weng, P. D. Zhou, L. L. Zhang, Z. G. Huang and W. Zhang, Nanoscale, 2017, 9, 9825 RSC.
- M. Rogóż, H. Zeng, C. Xuan, D. S. Wiersma and P. Wasylczyk, Adv. Opt. Mater., 2016, 4, 1689 CrossRef.
- C. Yuan, D. J. Roach, C. K. Dunn, Q. Y. Mu, X. Kuang, C. M. Yakacki, T. J. Wang, K. Yu and H. J. Qi, Soft Matter, 2017, 13, 5558 RSC.
- S. Maeda, Y. Hara, T. Sakai, R. Yoshida and S. Hashimoto, Adv. Mater., 2007, 19, 3480 CrossRef.
- Y. Ma, Y. Y. Zhang, B. S. Wu, W. P. Sun, Z. G. Li and J. Q. Sun, Angew. Chem., Int. Ed., 2011, 50, 6254 CrossRef PubMed.
- J. J. Liang, L. Huang, N. Li, Y. Huang, Y. P. Wu, S. L. Fang, J. Oh, M. Kozlov, Y. F. Ma, F. F. Li, R. Baughman and Y. S. Chen, ACS Nano, 2012, 6, 4508 CrossRef PubMed.
- S. Banisadr and J. Chen, Sci. Rep., 2017, 7, 17521 CrossRef PubMed.
- M. Y. Ji, N. Jiang, J. Chang and J. Q. Sun, Adv. Funct. Mater., 2014, 24, 5412 CrossRef.
- F. J. Ge and Y. Zhao, Chem. Sci., 2017, 8, 6307 RSC.
- X. B. Zhang, Z. B. Yu, C. Wang, D. Zarrouk, J. W. T. Seo, J. C. Cheng, A. D. Buchan, K. Takei, Y. Zhao, J. W. Ager, J. J. Zhang, M. Hettick, M. C. Hersam, A. P. Pisano, R. S. Fearing and A. Javey, Nat. Commun., 2014, 5, 2983 CrossRef PubMed.
- S. M. Mirvakili and I. W. Hunter, Adv. Mater., 2017, 29, 1604734 CrossRef PubMed.
Footnote |
† Electronic supplementary information (ESI) available. See DOI: 10.1039/c8qm00190a |
|
This journal is © the Partner Organisations 2018 |
Click here to see how this site uses Cookies. View our privacy policy here.