DOI:
10.1039/C8QM00266E
(Research Article)
Mater. Chem. Front., 2018,
2, 2201-2211
On structure and phase transformation of uranium doped La2Hf2O7 nanoparticles as an efficient nuclear waste host†
Received
30th May 2018
, Accepted 22nd June 2018
First published on 26th June 2018
Abstract
The design and development of efficient and stable nuclear waste hosts has drawn intensive interest for long-lived lanthanides and actinides. A detailed investigation of their structure and potential structural evolution are crucial. In this study, we have synthesized lanthanum hafnate La2Hf2O7 nanoparticles (NPs) doped with uranium at different concentrations (0–10%) and investigated their structural transition. We have discovered that in our La2Hf2O7:U NPs, the uranium dopants are stabilized at both U4+ and U6+ oxidation states in which the U6+ oxidation state exists in octahedral uranate UO66− form. We also confirmed that the U4+ ions substituted the Hf4+ ions with a lifetime of ∼1.0 μs and the UO66− ions resided at the La3+ sites with a lifetime of ∼9.0 μs. More interestingly, the proportion of the U4+ ions in the La2Hf2O7:U NPs was higher than that of the UO66− ions at low doping level, but at the doping level higher than 2.5%, the fraction of the UO66− ions was greater than that of the U4+ ions. Furthermore, we studied the structural phase transformation from order pyrochlore to cotunnite of these La2Hf2O7:U NPs with increasing uranium doping level, and found that ordered pyrochlore phase favors the U4+ ions whereas disordered cotunnite phase favors the UO66− ions. We further used in situ Raman spectroscopy to confirm the reversible cotunnite to pyrochlore phase transformation of the La2Hf2O7:10%U NPs at 900 °C. Therefore, this work demonstrated the successful development of uranium doped La2Hf2O7 NPs and thorough characterization of the fundamental spectra of uranium ions, doping induced phase transformation, and structure–optical property correlation.
1. Introduction
Compounds with a general formula of A2B2O7 have received intense attention due to their high thermodynamic stability, high radiation stability, capability to incorporate lanthanides and actinides, and ability to form antisite defects by swapping A and B positions.1–6 Among these compounds, rare-earth hafnates RE2Hf2O7 possess various desirable properties, which are very important for different technological applications, such as computer tomography (CT),7 positron emission tomography (PET),8 high-energy radiation detectors,9 scintillation host materials,10 magnetic materials,11 among others.4,9,12–15
Uranium and its radioactive isotopes contribute to a high-level of nuclear waste, which needs to be properly disposed. Uranium ion has multiple oxidation states (i.e. +3, +4, +5 and +6), all of which are luminescence active with characteristics emission.16–19 Speciation studies of uranium ion in A2B2O7 compounds will be highly beneficial for fundamental uranium chemistry and nuclear industry. However, because of its complex nature and various existing valence states, the incorporation mechanism, oxidation state and structural environment of uranium ions in A2B2O7 compounds are uncharted and vague. For example, Zhang and coworkers investigated the phase evolution of U doped Y2Ti2O7 and Gd2Ti2O7.20 They have carried out detailed phase evolution investigation from Ln2Ti2O7 pyrochlores to Ln0.5U0.5Ti2O6 (Ln = Y and Gd) brannerites in glasses using various techniques such as X-ray diffraction, Raman spectroscopy, diffuse reflectance spectroscopy and electron microscopy. Shu et al. studied the effect of alpha irradiation on U doped Gd2Zr2O7.21 Their study revealed that main crystal structure does not change but weak structural ordering takes place on alpha irradiation. They have also found increase in radiation stability of Gd2Zr2O7 at high uranium concentrations. Lu et al. explored the effects of U3O8 on the phase and microstructure evolution of Gd2Zr2O7 and found that uranium is homogenously distributed in +4 and +6 oxidation states at Gd3+ and Zr4+ sites, respectively.22 One of us studied the speciation of uranium using luminescence spectroscopy for A2Zr2O7 (A = La, Nd and Gd) and the results show that uranium is stabilized as U4+ and UO66− in Gd2Zr2O7, only as UO66− in Nd2Zr2O7, and as both UO66− and UO22+ in La2Zr2O7.23–25 There are few more reports on uranium doped Gd2Zr2O7 wherein speciation of uranium was carried out in bulk phase using X-ray diffraction, Raman spectroscopy and X-ray photoelectron spectroscopy along with other suitable techniques.26–28 However, none of these reports includes studies on structure and phase evolution of uranium doped La2Hf2O7 nanoparticles (NPs).
Photoluminescence (PL) spectroscopy is an indispensable technique to probe optical properties and local structure of phosphors. It is also the most acceptable technique for detecting and estimating uranium in ultra-trace level in both solids as well as aqueous media.29 Meanwhile, Raman spectroscopy has been widely utilized as a tool to distinguish between the disordered fluorite and the ordered pyrochlore phases of A2B2O7 compounds. Due to its high sensitivity to oxygen-cation vibrations, Raman spectroscopy has the capability to probe local structure and to further identify disorder within the pyrochlore structure, which emerge from vacancies and defects that disrupt the translational symmetry. Moreover, X-ray photoelectron spectroscopy (XPS) is a surface-sensitive quantitative spectroscopic technique that measures the elemental composition, empirical formula, chemical state and electronic state of the elements that exist within a material. It is also one of the highly sensitive methods to probe oxygen vacancies. Therefore, in this work, we have used these three techniques together along with other traditional materials characterization techniques complementarily to investigate the structure and phase transition of the La2Hf2O7 NPs with different uranium doping levels. Lastly, for the first time, we observed reversible phase transformation in the La2Hf2O7:10%U NPs using in situ Raman measurement. Therefore, through the successful development of uranium doped La2Hf2O7 NPs and their thorough characterization of the fundamental spectra of uranium ions, doping induced phase transformation, and structure–optical property correlation, we believe this work open new research areas important for safe nuclear energy and sustainable environment.
2. Experimental
Fig. S1 (ESI†) depicts the schematic of the combined co-precipitation and molten-salt synthesis (MSS) procedure adopted to synthesize the La2Hf2O7:x mol%U NPs (x = 0.5–10.0).4,9,30,31 Additional synthesis and characterization details are provided in ESI† (S.1 and S.2).
3. Results and discussion
3.1 Phase, structure and morphological analysis
XRD is used to confirm the phase of the La2Hf2O7:x%U (x = 0, 0.5, 1, 2.5, 5, 7.5, and 10) NPs (Fig. S2, ESI†). Fourier transformed infrared (FTIR) spectra were collected (Fig. S3, ESI†) to identify the phase and rule out the formation of any additional phase of the La2Hf2O7:x%U NPs. Morphostructural characterization of the synthesized NPs was performed with scanning electron microscopy (SEM) and high-resolution transmission electron microscopy (HRTEM) as shown in Fig. S4 and S5 (ESI†). The presence of uranium dopant was confirmed by energy dispersive spectroscopy (EDS) (Fig. S6, ESI†). The doping efficiency and uniform distribution of constituent element were investigated using elemental mapping (Fig. S7, ESI†).
3.2 Raman spectroscopy
It is well known that the ideal pyrochlore phase has six well-resolved Raman active vibrational modes in the range of 200–1000 cm−1 which are represented as ΓP = A1g + Eg + 4F2g, whereas the fluorite phase has mainly one Raman active mode that is ΓF = F2g.32 This is because seven oxygen ions are randomly distributed at eight anion positions, which leads to structural disordering in the fluorite phase, and hence all the fine peaks of pyrochlore collapsed into one broader peak. More specifically, phase transformation from A2B2O6O′ pyrochlore (Fd
m space group) to AO2 fluorite (Fm
m, Z = 4) structure takes place through the disappearance of A1g and Eg Raman modes and decrease in the number of F2g modes from 4 to 1.
Radius ratio (rA/rB) plays an important role in determining which structure of A2B2O7 compounds attain.33 It has been reported that fluorite phase is more likely to form if rA/rB < 1.46 and while ordered pyrochlore phase is more likely to be stabilized if rA/rB is greater than 1.46 at room temperature. It was propose that rA/rB for different A2B2O7 compositions follows this trend: disordered fluorite phase (DFP) rA/rB < 1.21 < δ-phase rA/rB < 1.42–1.44 < ordered pyrochlore phase (OPP) rA/rB < 1.78–1.83 < monoclinic pyrochlore rA/rB < 1.92.34 La2Hf2O7 is the most favorable candidate with radius ratio of 1.45 to be stabilized in pyrochlore phase. Fig. 1a shows the Raman spectra of the as-synthesized La2Hf2O7:U NPs with different uranium concentrations. Undoped sample has six well-resolved Raman peaks at 306, 324, 402, 501, 521 and 601 cm−1 pertaining to the vibrations of La–O and Hf–O bonds. These peaks are assigned to F2g, Eg, F2g, A1g F2g and F2g modes, respectively.35 The vibrational modes of F2g, Eg, and F2g at low frequency region of 300–400 cm−1 arise from vibrations of the La–O and Hf–O bonds. On the other hand, the high frequency bands at 501, 522 and 601 cm−1 arise from the stretching of the Hf–O bonds.35 However, the complete pyrochlore phase is preserved only up to 1.0% uranium doping level. After that, the fine OPP structure starts to collapse into broader peaks and disordered fluorite phase or cotunnite phase evolves and coexists with OPP. At 2.5% uranium doping level and above, there is complete disordering of the La2Hf2O7 pyrochlore phase and a broad peak around 715 cm−1 appears. Its intensity keeps increasing and even overpowers the broad fluorite peak at 7.5% and 10% uranium doping levels. This peak is not a fundamental Raman vibrational mode of pyrochlore-structured A2B2O7 type oxides. It is believed to appear due to the distortions of the BO6 octahedra.35,36 In La2Hf2O7, it has been attributed to distortion in HfO6 octahedra and other kind of structural defects due to doping of uranium at La/Hf site. The average metal–oxygen bond lengths in the fluorite phase is close to those existing in ideal pyrochlore structure, but is relatively much smaller than those in cotunnite-type A2B2O7 structure, suggesting that the fluorite phase has more covalent character than the cotunnite-type structure.37 In the cotunnite-type structure, coordination number of metal ions is generally 8- or 9-fold, whereas in the fluorite-type structures, metal ions coordinate with oxygen ion in 7- or 8-fold coordination. This suggests La2Hf2O7 has the tendency to form ionic bonding with higher coordination number at high uranium doping concentration. Furthermore, based on Raman spectroscopy data, the increase of uranium doping level induces the gradual phase transformation from pyrochlore to fluorite and then cotunnite as observed by the appearance of broad peaks at 307 and 715 cm−1. Zhang et al. have also observed such process in U doped Gd2Zr2O7 but by applying pressure of 22 GPa, which finally transformed into a disordered fluorite structure on release of pressure.28
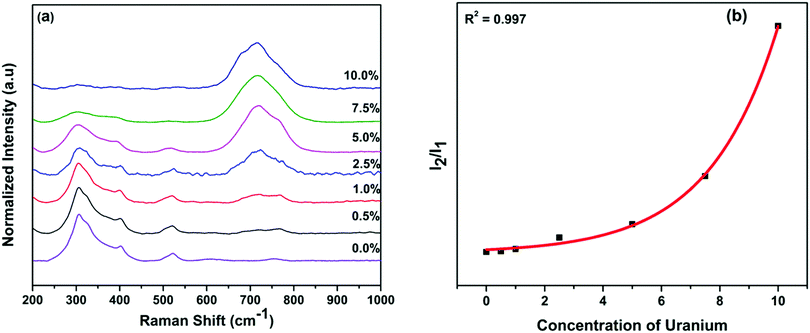 |
| Fig. 1 (a) Raman spectra of the La2Hf2O7:x%U NPs (x = 0, 0.5, 1, 2.5, 5, 7.5, and 10) and (b) corresponding integrated Raman intensity ratio of the distorted HfO6 octahedra (I2) and the main F2g Raman mode of the ordered pyrochlore (I1). This Raman intensity ratio serves as a qualitative indicator of the progression of anion disorder as a function of uranium doping concentration in the La2Hf2O7 host. | |
As a quantitative measure of the extent of anion disordering in the La2Hf2O7:U NPs as a function of uranium doping concentration, the ratio of integrated Raman intensity between Raman active vibration mode of the distorted HfO6 octahedra and the main F2g Raman mode at ω0 ≈ 307
cm−1 is plotted in Fig. 1b.38 Moreover, we annealed our samples at high temperature to enhance cation ordering and reduce strain, but there was little effect on the Raman spectra of our La2Hf2O7:U NPs.39 Thus, this ratio selectively indicates disorder on the anion sub-lattice.
3.3 XPS analysis
To confirm the oxidation state of uranium ion and other constituent elements in the La2Hf2O7:U NPs, XPS was carried out for the lowest and highest composition. Fig. S8 (ESI†) depicts the XPS spectra of La 3d, Hf 4f and O 1s core level electron for the La2Hf2O7:U NPs at various doping levels. Fig. S8a (ESI†) shows the La 3d XPS spectra that indicated the binding energies (BE) values for La 3d5/2 and La 3d3/2 are approximately 839.7 and 852 eV in addition to satellite peaks at 865.6 and 877.4
eV at 0.5% uranium doping. The La 3d X-ray photoelectron peak splits into 3d5/2 and 3d3/2 due to strong orbit coupling. There may be some additional contribution of ligand to metal charge transfer (oxygen to hafnium) which cause further splitting to these lines.40 The BE difference in 3d3/2 and 3d5/2 states is around 16.5 eV, which indicates the stabilization of lanthanum ion in +3 oxidation state.41 Most of the report on XPS spectra of HfO242–44 clearly shows spin–orbit coupling induced splitting in Hf 4f peak to 4f7/2 and 4f5/2 similar to what we have observed in our La2Hf2O7:U NPs (Fig. S8b, ESI†). The BE values for these two peaks is approximately around BE = 18.9 eV and BE = 20.6 eV for 0.5% uranium doped La2Hf2O7 NPs with 4f spin–orbit splitting energy values around ∼1.92 eV. All these data are in concordance with Hf in +4-oxidation state. Although it can be seen from Fig. S8b (ESI†) that the Hf 4f peaks become more pronounced for the higher doping concentrations of U, due to the overlap between the La 5p and Hf 4f spectra, the peak contributions are not clear. On increasing the concentration of uranium ion, there is slight variation in peak position for X-ray photoelectron of La-3d and Hf-4f due to slight doping inducing change in electronic localization as well as density. The Hf 4p and U 4f spectra have overlapping peaks. The O 1s XPS spectra are shown in Fig. S8c (ESI†). As to-be-confirmed by PL data that there is uranium distribution (both U4+ and U6+) at both La3+/Hf4+ site, there are large density of charge compensating defect formation in our La2Hf2O7:U NPs to take care of charge neutrality. One of predominant defects would be oxygen vacancy. The area under the surface hydroxyl/under-coordinated O 1s peak (Ov) is increasing with uranium doping concentration, suggesting the formation of oxygen vacancies due to charge neutrality.
Since XPS studies can distinguish different oxidation states of uranium according to the binding energy of 4f orbit, we have used XPS to confirm the oxidation state of uranium in our La2Hf2O7 NPs (Fig. 2a). From the XPS analysis, we confirmed the presence of both U4+ and U6+ in all samples with different concentrations. At higher concentrations the XPS spectra displayed two peaks at binding energies values of 382.5 and 393.3 corresponding to U 4f7/2 and U 4f5/2, respectively. This difference is because of spin orbit coupling. However, at lower doping concentrations (0.5%, and 1%) the U 4f5/2 peak was absent. Because the U 4f5/2 peak was not observed at lower concentrations, we were unable to calculate the fractional ratio of U4+ and U6+ species in the 0.5% and 1% uranium doped La2Hf2O7:U samples. The XPS parameters as calculated for the rest of the samples are shown in Table S2 (ESI†). From the ratio analysis, it is clearly evident that as the concentration of uranium dopant increases, there is an exponential decrease in the U4+/U6+ ratio (Fig. 2b), confirming that U4+ is dominating at lower concentrations, while at higher concentrations it is U6+. This observation is consistent with our PL emission spectroscopy and lifetime studies as discussed in the next section.
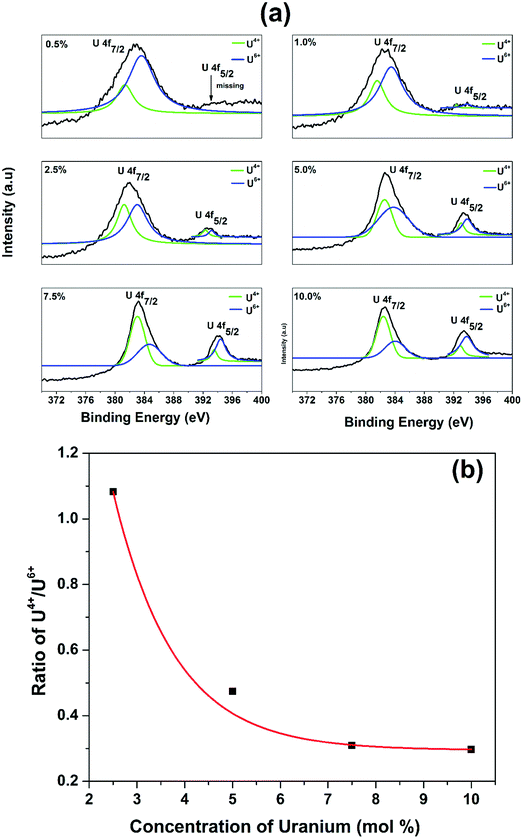 |
| Fig. 2 (a) Uranium 4f7/2 XPS spectra of the La2Hf2O7:U NPs at different doping levels and (b) variation of the U4+/U6+ ratio as a function of uranium doping levels (2.5–10%). | |
3.4 Photoluminescence
3.4.1 Emission and excitation spectroscopy.
PL emission spectrum (Fig. 3a) of the La2Hf2O7:1.0%U NPs at λex = 230 nm showed two different features in region a (Ra: 440–500 nm) and region b (Rb: 540–570 nm). In Ra, there is a cluster of four observable featureless bands centered at 451, 468, 482 and 493 nm in the blue region. In Rb, there is a single broadband at 550 nm in the green region.
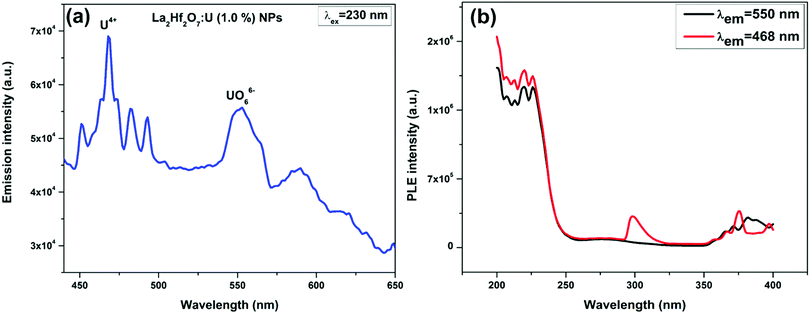 |
| Fig. 3 (a) Emission spectrum and (b) excitation spectrum of the La2Hf2O7:1.0 mol%U NPs. | |
The cluster of four bands in blue region is definitely not arising because of uranyl ions as they have unique signature of equally spaced vibronically coupled emission band whose value is close to symmetric stretching Raman mode of uranyl ion. Moreover, the position of the first band in the emission spectrum (υ0–0) is a unique signature for deciding the number of oxygen atom coordinated to uranium and its bond order, which is known as zero phonon line (ZPL). It corresponds to the electronic transition from triplet 3Πu state to singlet 1Σ+g (D∞h) state from oxygen to the non-bonding orbital of uranium (5fδu and 5fφu). In our spectrum, it is located at 451 nm, which is much lower than the normally observed in case of UO22+.45–47 This feature further supports the fact that the sharp features in Ra region corresponds to uranium in +4 oxidation state. It has been also reported previously that uranium has high tendency to be stabilized in both U4+ and U5+/U6+ in A2B2O7 type pyrochlore oxides.48,49 Indeed, in our earlier work on U doped Gd2Zr2O7, we could confirm that uranium has a tendency to stabilize in both U(+4) and U(+6) states.24 In our case here, the emission peaks in region Ra are attributed to f → f transitions of the U4+ 5f2 ion.24,50–52 The spectral splitting of the U(IV) bands is the result of ligand field induced by hafnate ion. This particular emission is attributed to the relaxation of the highest energy 3F2 charge-transfer excited-state manifold (5f16d1) to Russell–Saunders coupled 3H4(5f2) ground state and higher lying energy states derived from the 5f2 electronic configuration.24
On the other hand, the broad peak in the green emission zone Rb at 550 nm is originated from uranium ion in +6 oxidation state, which allowed for oxygen to uranium charge transfer.23–25,45–47,53,54 Therefore, it is due to ligand to metal charge transfer (LMCT) transition and is typical of uranium stabilizing in octahedral uranate ion UO66−.23,53 Visible emission in the green region emerges due to ligand to metal charge transfer (LMCT) involving bonding oxygen orbitals (Πu, Πg, Ωg, and Ωu) to nonbonding 5fδ and 5fφ orbitals of uranium ion.
Apparently, uranyl ion (UO22+) vibronic features are very distinct from those that arises due to LMCT transitions. Normally LMCT induced vibronic transitions are very broad and often structureless. It is very difficult to identify the zero-phonon lines (ZPLs) and vibration modes from there. This is because of the fact that broadening due to LMCT induces lattice displacements and charge-hole relaxation in the valence band.55 Previously published work has shown that uranyl ion has a unique emission peak with constant spacing.23–25,45–47,53,54 The origin of such vibronic coupling is the strong interaction of the ground state Raman active O
U
O symmetric stretching mode with the 3Πu electronic triplet excited state (generally observed between 780 and 900 cm−1). Isolated uranyl ion has three Raman active modes at ground state vibrational frequencies of 830 (ω2), 920 (ω2) and 250 (ω3) cm−1 due to symmetric stretching, asymmetric stretching and bending, respectively. However, based on Franck–Condon principle only symmetric stretching modes are allowed to couple with the electric dipole transition of uranyl ion.56 Therefore, as a unique signature of the uranyl ion, vibronic band progresses harmonically in the frequency of the O
U
O symmetric stretching. These are known as false origins:57ω2 and ω2 are coupled to the vibronic transitions of the symmetric stretching mode that progress in the frequency of ω1. No harmonics of asymmetric stretching ω2 and bending mode ω3 appear in the uranyl vibronic spectra because they are non Franck–Condon mode. Absence of such signature clearly rules out the stabilization of uranium ion as UO22+ in La2Hf2O7.
Among various stable forms, hexavalent uranium ions depending on the conditions of concentration, annealing temperature, and structure of host can have different molecular structures leading to tetrahedral uranate UO42−, octahedral uranate UO66− or uranyl UO22+ species. Of these uranyl ions, UO22+ have been studied most extensively, because it is the most prevalent form of natural uranium in the ecological system. Uranyl ions are characterized by uranium–oxygen partial triple bond character, whereas octahedral and tetrahedral uranate ions are characterized by uranium–oxygen single bond. Crystal lattice hosts that favor the formation of U–O bond (singly bonded) will stabilize uranium ion in the form of uranate ions while those that offer close packed environments favor shorter triply bonded uranium–oxygen bonds to stabilize uranium in the form of UO22+ ions.58 From our earlier work on magnesium aluminate spinel and strontium silicate,45,46 uranium stabilizes as UO22+ in MgAl2O4 whereas as both U(+4) and UO66− in Gd2Zr2O7.24 On the other hand, it tends to stabilize as UO66− in SrZrO3, Nd2Zr2O7, and Sr2CeO4.23,53,54 In this case here, uranium stabilizes as both +4 and +6 oxidation state in the form of U4+ ion as well as UO66− ion at 1.0% uranium doped La2Hf2O7.
The representative excitation bands corresponding to U4+ and UO66− emissions were taken from the La2Hf2O7:1.0 mol%U NPs (Fig. 3b). The distinct features in the two cases are another indication of the fact that the emission features in blue and green region has different origin altogether. The shoulder around 200–250 nm is attributed to oxygen to uranium charge transfer transition whereas the fine structure from 300–400 nm is the intra f–f band of uranium ion.
Fig. 4a depicts the emission spectra of the La2Hf2O7:U NPs for various uranium concentrations and Fig. 4b displayed the effect of uranium ion concentration on emission intensity for both U4+ emission at 458 nm and UO66− emission at 550 nm. The emission intensity increases up to 1.0% uranium doping, and after that, there is an emission intensity reduction. Such phenomenon is attributed to concentration quenching. At higher doping concentration, the distance between two-activator ions decreased to an extent where non-radiative energy transfer between them is facilitated. That distance in photophysical parlance is called critical distance. To confirm the non-radiative energy transfer mechanism, which leads to concentration quenching, the critical distance (Rc) needs to be determined using the following equation:
where
Rc is called critical distance and is defined as the minimum possible distance between the dopant ions at which non-radiative energy transfer takes place,
V is the volume of the unit cell,
Xc is the critical concentration of dopant ion, and
N is the number of cations present in one formula unit of host. The values of
V and
N for La
2Hf
2O
7 are 1250.32 Å
3 and 16, respectively. Considering
Xc = 1.0% (0.01), critical energy transfer distance
Rc in the La
2Hf
2O
7:1.0%U NPs was estimated to be 24.62 Å. In this case, non-radiative energy transfer takes place at the U–U distance greater than 10 Å
via electric multipolar interaction.
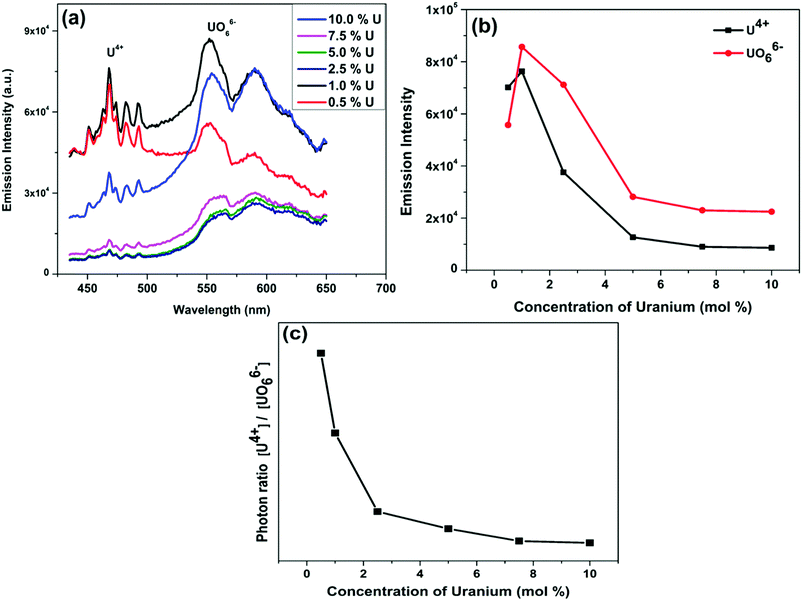 |
| Fig. 4 (a) Emission spectra of the La2Hf2O7:U NPs with various uranium doping concentrations with λex = 230 nm, and (b) corresponding plot of emission intensity and (c) ratio of emission intensity from the U4+ and UO66− ions as a function of the uranium doping concentration. | |
In addition, uranium was stabilized as both U4+ and UO66− ions in all doping concentrations in the La2Hf2O7 host, but their relative intensity changes (Fig. 4a). At low concentrations, the fraction of U4+ is more than that of UO66− whereas the trends reverses at high doping concentrations as plotted in Fig. 4c. This in an interesting observation consistent with XPS data, which can be correlated to some kind of structural changes. At low uranium doping, A2B2O7 structure favors the stabilization of U4+ over that of UO66−. At high doping level, A2B2O7 structure favors UO66− over U4+. It has been reported that the structure of host materials plays huge roles in stabilizing different oxidation states and coordinations of doping ions. Perovskite structure tends to stabilize the U(VI) oxidation state in UO66− coordination53 whereas spinel structure tends to favor the same oxidation state but in a different geometry as UO22+ ion.45 On the other hand, fluorite type A2B2O7 composition favors the stabilization of both U4+ and U6+.24 In this case, our Raman spectroscopy results are assimilate our PL observations, supporting the fact that oxidation stabilization of uranium is dependent on the structural and phase evolution of the NPs.
3.4.2 Lifetime spectroscopy.
Investigating the local site occupied by U ions in the A2B2O7 type pyrochlore structure is very important from the point of view of nuclear waste immobilization. In ideal pyrochlore lattice, there are two cationic sites having 16c and 16d Wyckoff positions with different coordination numbers (CNs). In addition, there are two anionic sites at 8a and 48f Wyckoff positions. Dopant ion occupancy on these sites depends on their ionic radii. The 16c cation site having CN of six anions on the 48f sites and two on the 8a sites is usually occupied by large size dopant ions, whereas the 16d cationic site having CN of six anions on the 48f sites is usually occupied by small sized ions.28
As far as ionic radius is concerned, uranium in 8-coordination (100 pm) is shorter than 8-coordinated La3+ ion (116 pm), so it can easily reside on the lanthanum site. On the other hand, 6-coordinated Hf4+ ion (71 pm) is smaller, and its site is difficult to accommodate large sized uranium ion (89 pm). On the other hand, for U4+ ion substitution, Hf4+ site favors charge matching whereas there is a need for charge compensation at La3+ site. Charge matching is energetically more favorable situation than size matching. Therefore, U4+ ion feels less distortion at Hf4+ site relative to at La3+ site due to its same ionic charge with Hf4+ ion. Therefore, room temperature luminescence decay profiles of the La2Hf2O7:1.0%U NPs were measured with emission wavelengths of 468 nm and 552 nm after excited at 230 nm (Fig. 5a and b). The luminescence decay profile corresponding to U4+ ion (468 nm) displayed a biexponential behavior with two lifetime values. It indicates that U4+ ion is not homogeneously distributed in the La2Hf2O7 matrix.
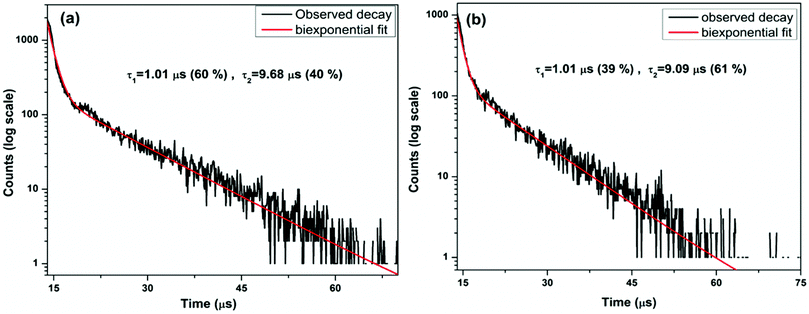 |
| Fig. 5 Luminescence decay profiles of the La2Hf2O7:1.0%U NPs with emission wavelengths of (a) 468 nm and (b) 552 nm after excited at 230 nm. | |
Based on the fitting data, the decay curve shows two different lifetime values of 1.01 and 9.68 μs with magnitudes 60% and 40%, respectively. The long lifetime measured from the La2Hf2O7:1.0%U NPs (9.68 μs) is ascribed to uranate ion localized at the La3+ site whereas the fast decaying uranyl ion (1.01 μs) is attributed to U4+ ion localized at Hf4+ site. The PL decay profile corresponding to UO66− ion (550 nm) also displayed a biexponential behavior with lifetime values of 1.01 μs (39%) and 9.09 μs (61%). Here the fast decaying uranium is attributed to U(IV) ion localized at the Hf4+ site whereas the slow decaying uranium ion is attributed to UO66− ion at La3+ site. In this case, one U6+ ion replaces two La3+ sites and charge-compensating defects are cation vacancies.
3.5 Structural evolution of the La2Hf2O7:10%U NPs by in situ Raman spectroscopy
To further investigate the structural evolution of our La2Hf2O7:x%U NPs, we took in situ Raman spectra of La2Hf2O7:10%U NPs as an example with our initial effort. From the discussion above, the La2Hf2O7:10%U NPs exists in complete cotunnite structure. The average metal–oxygen bond length in the fluorite-phase is much closer to those existing in ideal pyrochlore structures, but is relatively much smaller than that in cotunnite-type A2B2O7 structures, suggesting that the fluorite phases have more covalent character than the cotunnite-type.37 In the cotunnite-type structure though, coordination number of metal ion is generally 8 or 9-fold whereas in the fluorite-type structures metal ion coordinates with oxygen ion in 7 or 8 fold coordination. This suggest that at high uranium concentration hafnate pyrochlore has tendency to form ionic bonding with higher coordination number.
With increasing temperature from 25 °C to 950 °C (Fig. 6a), this particular the La2Hf2O7:10%U sample underwent phase transformation from cotunnite phase to ordered pyrochlore phase. At the highest reached measurement temperature, i.e. 950 °C, all the Raman modes expected for ordered pyrochlore phase were found from the heated sample with the almost complete disappearance of the 715 cm−1 peak originated from the structurally disorder cotunnite phase (top panel of Fig. 6a) while the disordered fluorite phase was probably unseen. The complete phase transformation took place around 900 °C. Even more interestingly and for the first time, we found that the phase change is reversible, i.e., the Raman band corresponding to cotunnite phase reappear after the sample was cooled down back to room temperature (Fig. 6b). With the initial exciting Raman results collected so far, further investigations are undergoing, including in situ Raman studies of other composition along with in situ PL, X-ray absorption spectroscopy and neutron diffraction measurements, and will be reported separately in the near future.
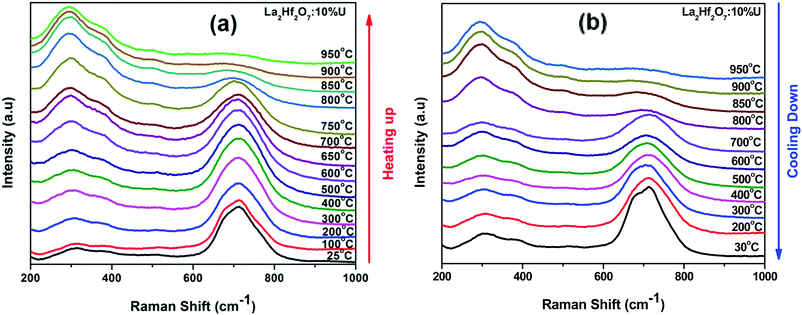 |
| Fig. 6
in situ Raman spectra of the La2Hf2O7:10%U NPs in consecutive heating and cooling cycle. | |
4. Conclusion
In this work, uranium doped La2Hf2O7 nanoparticles were successfully synthesized using a combined co-precipitation and molten salt synthesis method. XRD and FTIR demonstrated the formation of pure La2Hf2O7 phase with particle size in the nanodomain as confirmed using electron microscopy. Emission and lifetime spectroscopy further suggested the existence of U4+ ions along with U6+ ions as UO66− species. The same has been further corroborated by more oxidation state sensitive XPS. Luminescence lifetime measurement confirmed the stabilization of fast decaying U4+ at Hf4+ site whereas slowly decaying U6+ tends to stabilize at La3+ site. Further concentration dependence studies showed maximum emission output for 1.0% uranium doped samples and the concentration quenching in these NPs was attributed to non-radiative energy transfer via multipolar interaction. Another interesting phenomenon was observed from the emission spectra as well as XPS: at low uranium doping concentration, the proportion of U4+ ion was more than that of U6+, whereas at high uranium doping concentration, the opposite happened. We successfully explained this phenomenon using Raman spectroscopy, which demonstrated a structural phase transition from order pyrochlore to cotunnite with increasing uranium doping concentration. It was concluded that ordered pyrochlore phase favors uranium stabilization in +4-valence state whereas disordered cotunnite phase energetically favors octahedral uranate ions UO66−. To further understand the structural evolution, in situ Raman measurements were carried out on the La2Hf2O7:10%U NPs between room temperature and 950 °C. A reversible structural transition was found at 900 °C between ordered pyrochlore phase and disorder cotunnite phase. Therefore, it is expected that this work open new research areas of fundamental solid-state spectroscopy of uranium, doping induced pyrochlore to fluorite phase transition, and structure–optical property correlation in doped A2B2O7 with urgent importance to nuclear power industry and sustainable environment.
Conflicts of interest
There are no conflicts to declare.
Acknowledgements
The authors thank the financial support by the National Science Foundation under CHE (award #1710160) and DMR (grant #1523577) and the USDA National Institute of Food and Agriculture (award #2015-38422-24059, for the Integrating Food Science/Engineering and Education Network (IFSEEN) program). The Department of Chemistry at the University of Texas Rio Grande Valley is grateful for the generous support provided by a Departmental Grant from the Robert A. Welch Foundation (Grant No. BX-0048). SKG would like to thanks United States-India Education Foundation (USIEF) and Institute of International Education (IIE) for his Fulbright Nehru Postdoctoral Fellowship (award# 2268/FNPDR/2017). The in situ Raman measurements were conducted at the Center for Nanophase Materials Science, which is a DOE Office of Science User Facility, and the authors thank Dr A. Puretzky for technical assistance. Authors would also like to thank Ms Melonie Thomas for HRTEM and Pragathi Darapaneni, Louisiana State University for XPS measurements.
References
- S. Zhang, H. Zhang, F. Zhao, M. Jiang, H. Xiao, Z. Liu and X. Zu, Impact of isovalent and aliovalent substitution on the mechanical and thermal properties of Gd2Zr2O7, Sci. Rep., 2017, 7, 6399 CrossRef PubMed.
- Y. Feng, S. Zhu, J. Bian, F. Chen, S. Chen, C. Ma, H. Liu and B. Fang, Magnetic and electrical transport properties of the pyrochlore iridate Bi2−xCoxIr2O7, J. Magn. Magn. Mater., 2018, 451, 283–287 CrossRef.
- R. Radha, R. Kumar, M. Sakar and S. Balakumar, Understanding the lattice composition directed in situ structural disorder for enhanced visible light photocatalytic activity in Bismuth iron niobate pyrochlore, Appl. Catal., B, 2018, 225, 386–396 CrossRef.
- M. Pokhrel, K. Wahid and Y. Mao, Systematic studies on RE2Hf2O7:5% Eu3+ (RE = Y, La, Pr, Gd, Er, and Lu) nanoparticles: effects of the A-site RE3+ cation and calcination on structure and photoluminescence, J. Phys. Chem. C, 2016, 120, 14828–14839 CrossRef.
- G. M. Mustafa, S. Atiq, S. K. Abbas, S. Riaz and S. Naseem, Tunable structural and electrical impedance properties of pyrochlores based Nd doped lanthanum zirconate nanoparticles for capacitive applications, Ceram. Int., 2018, 44, 2170–2177 CrossRef.
- K. Zhang, Z. He, L. Peng, H. Zhang and X. Lu, Self-propagating synthesis of Y2−xNdxTi2O7 pyrochlore and its aqueous durability as nuclear waste form, Scr. Mater., 2018, 146, 300–303 CrossRef.
- V. A. Vorozhtcov, V. L. Stolyarova, S. I. Lopatin, E. P. Simonenko, N. P. Simonenko, K. A. Sakharov, V. G. Sevastyanov and N. T. Kuznetsov, Vaporization and thermodynamic properties of lanthanum hafnate, J. Alloys Compd., 2018, 735, 2348–2355 CrossRef.
- G. Zhou, Z. Wang, B. Zhou, Y. Zhao, G. Zhang and S. Wang, Fabrication of transparent Y2Hf2O7 ceramics via vacuum sintering, Opt. Mater., 2013, 35, 774–777 CrossRef.
- K. Wahid, M. Pokhrel and Y. Mao, Structural, photoluminescence and radioluminescence properties of Eu3+ doped La2Hf2O7 nanoparticles, J. Solid State Chem., 2017, 245, 89–97 CrossRef.
- L. An, A. Ito and T. Goto, Fabrication of transparent Lu2Hf2O7 by reactive spark plasma sintering, Opt. Mater., 2013, 35, 817–819 CrossRef.
- M. D. Alice, K. Peter and L. R. Corruccini, Heat capacity of the frustrated magnetic pyrochlores Gd2Zr2O7 and Gd2Hf2O7, J. Phys.: Condens. Matter, 2008, 20, 235208 CrossRef PubMed.
- S. Gu, S. Zhang, D. Xu, W. Li and J. Yan, Evolution of microstructure and hot corrosion performance of La2Hf2O7 ceramic in contact with molten sulfate-vanadate salt, Ceram. Int., 2018, 44, 2048–2057 CrossRef.
- Y. Ji, D. Jiang and J. Shi, La2Hf2O7:Ti4+ ceramic scintillator for X-ray imaging, J. Mater. Res., 2005, 20, 567–570 CrossRef.
- R. A. Hansel, S. Desai, S. W. Allison, A. Heyes and D. G. Walker, Emission lifetimes of europium-doped pyrochlores for phosphor thermometry, J. Appl. Phys., 2010, 107, 016101 CrossRef.
- G. R. Lumpkin, K. R. Whittle, S. Rios, K. L. Smith and N. J. Zaluzec, Temperature dependence of ion irradiation damage in the pyrochlores La2Zr2O7 and La2Hf2O7, J. Phys.: Condens. Matter, 2004, 16, 8557 CrossRef.
- E. Hashem, J. A. Platts, F. Hartl, G. Lorusso, M. Evangelisti, C. Schulzke and R. J. Baker, Thiocyanate complexes of uranium in multiple oxidation states: a combined structural, magnetic, spectroscopic, spectroelectrochemical,
and theoretical study, Inorg. Chem., 2014, 53, 8624–8637 CrossRef PubMed.
- L. S. Natrajan, Developments in the photophysics and photochemistry of actinide ions and their coordination compounds, Coord. Chem. Rev., 2012, 256, 1583–1603 CrossRef.
- M. Sobczyk, J. Drożdżyński, R. Lisiecki and W. Ryba-Romanowski, Near infrared and visible luminescence of U3+-doped PbCl2 single crystals, J. Lumin., 2008, 128, 185–189 CrossRef.
- R. Steudtner, T. Arnold, K. Großmann, G. Geipel and V. Brendler, Luminescence spectrum of uranyl(V) in 2-propanol perchlorate solution, Inorg. Chem. Commun., 2006, 9, 939–941 CrossRef.
- Y. Zhang, L. Kong, R. D. Aughterson, I. Karatchevtseva and R. Zheng, Phase evolution from Ln2Ti2O7 (Ln = Y and Gd) pyrochlores to brannerites in glass with uranium incorporation, J. Am. Ceram. Soc., 2017, 100, 5335–5346 CrossRef.
- X. Shu, L. Fan, Y. Xie, W. Zhu, S. Pan, Y. Ding, F. Chi, Y. Wu and X. Lu, Alpha-particle irradiation effects on uranium-bearing Gd2Zr2O7 ceramics for nuclear waste forms, J. Eur. Ceram. Soc., 2017, 37, 779–785 CrossRef.
- X. Lu, C. Hou, Y. Xie, X. Shu, Y. Ding, D. Ma, W. Ren and L. Bian, High capacity immobilization of U3O8 in Gd2Zr2O7 ceramics via appropriate occupation designs, Ceram. Int., 2017, 43, 3015–3024 CrossRef.
- S. K. Gupta, C. Reghukumar, M. Keskar and R. Kadam, Revealing the oxidation number and local coordination of uranium in Nd2Zr2O7 pyrochlore: a photoluminescence study, J. Lumin., 2016, 177, 166–171 CrossRef.
- S. K. Gupta, C. Reghukumar, N. Pathak, K. Sudarshan, D. Tyagi, M. Mohapatra, P. Pujari and R. Kadam, Speciation of uranium and doping induced defects in Gd1.98U0.02Zr2O7: photoluminescence, X-ray photoelectron and positron annihilation lifetime spectroscopy, Chem. Phys. Lett., 2017, 669, 245–250 CrossRef.
- M. Mohapatra, B. Rajeswari, N. Hon and R. Kadam, Uranium luminescence in La2Zr2O7: effect of concentration and annealing temperature, Luminescence, 2016, 31, 1519–1523 CrossRef PubMed.
- K. G. Kutty, R. Asuvathraman, R. R. Madhavan and H. Jena, Actinide immobilization in crystalline matrix: a study of uranium incorporation in gadolinium zirconate, J. Phys. Chem. Solids, 2005, 66, 596–601 CrossRef.
- X. Shu, X. Lu, L. Fan, R. Yang, Y. Ding, S. Pan, P. Zhou and Y. Wu, Design and fabrication of Gd2Zr2O7-based waste forms for U3O8 immobilization in high capacity, J. Mater. Sci., 2016, 51, 5281–5289 CrossRef.
- F. Zhang, M. Lang, C. Tracy, R. C. Ewing, D. J. Gregg and G. Lumpkin, Incorporation of uranium in pyrochlore oxides and pressure-induced phase transitions, J. Solid State Chem., 2014, 219, 49–54 CrossRef.
- M. C. Phillips, B. E. Brumfield, N. LaHaye, S. S. Harilal, K. C. Hartig and I. Jovanovic, Two-dimensional fluorescence spectroscopy of uranium isotopes in femtosecond laser ablation plumes, Sci. Rep., 2017, 7, 3784 CrossRef PubMed.
- Y. Mao, X. Guo, J. Y. Huang, K. L. Wang and J. P. Chang, Luminescent nanocrystals with A2B2O7 composition synthesized by a kinetically modified molten salt method, J. Phys. Chem. C, 2009, 113, 1204–1208 CrossRef.
- Y. Mao, T. J. Park, F. Zhang, H. Zhou and S. S. Wong, Environmentally friendly methodologies of nanostructure synthesis, Small, 2007, 3, 1122–1139 CrossRef PubMed.
- K. M. Turner, D. R. Rittman, R. A. Heymach, C. L. Tracy, M. L. Turner, A. F. Fuentes, W. L. Mao and R. C. Ewing, Pressure-induced structural modifications of rare-earth hafnate pyrochlore, J. Phys.: Condens. Matter, 2017, 29, 255401 CrossRef PubMed.
- M. Subramanian, G. Aravamudan and G. S. Rao, Oxide pyrochlores—a review, Prog. Solid State Chem., 1983, 15, 55–143 CrossRef.
- C. Stanek, C. Jiang, B. Uberuaga, K. Sickafus, A. Cleave and R. Grimes, Predicted structure and stability of A4B3O12 δ-phase compositions, Phys. Rev. B: Condens. Matter Mater. Phys., 2009, 80, 174101 CrossRef.
- N. Garg, K. Pandey, C. Murli, K. Shanavas, B. P. Mandal, A. Tyagi and S. M. Sharma, Decomposition of lanthanum hafnate at high pressures, Phys. Rev. B: Condens. Matter Mater. Phys., 2008, 77, 214105 CrossRef.
- M. De los Reyes, K. R. Whittle, Z. Zhang, S. E. Ashbrook, M. R. Mitchell, L.-Y. Jang and G. R. Lumpkin, The pyrochlore to defect fluorite phase transition in Y2Sn2−xZrxO7, RSC Adv., 2013, 3, 5090–5099 RSC.
- H. Y. Xiao, F. Zhang, F. Gao, M. Lang, R. C. Ewing and W. J. Weber, Zirconate pyrochlores under high pressure, Phys. Chem. Chem. Phys., 2010, 12, 12472–12477 RSC.
- D. R. Rittman, K. M. Turner, S. Park, A. F. Fuentes, C. Park, R. C. Ewing and W. L. Mao, Strain engineered pyrochlore at high pressure, Sci. Rep., 2017, 7, 2236 CrossRef PubMed.
- A. F. Fuentes, K. Boulahya, M. Maczka, J. Hanuza and U. Amador, Synthesis of disordered pyrochlores, A2Ti2O7 (A = Y, Gd and Dy), by mechanical milling of constituent oxides, Solid State Sci., 2005, 7, 343–353 CrossRef.
- D. A. Pawlak, M. Ito, M. Oku, K. Shimamura and T. Fukuda, Interpretation of XPS O (1s) in mixed oxides proved on mixed perovskite crystals, J. Phys. Chem. B, 2002, 106, 504–507 CrossRef.
- J. P. Zuniga, S. K. Gupta, M. Pokhrel and Y. Mao, Exploring the optical properties of La2Hf2O7:Pr3+ nanoparticles under UV and X-ray excitation for potential lighting and scintillating applications, New J. Chem., 2018, 42, 9381–9392 RSC.
- T.-M. Pan, F.-H. Chen and Y.-H. Shao, High-performance InGaZnO thin-film transistor incorporating a HfO2/Er2O3/HfO2 stacked gate dielectric, RSC Adv., 2015, 5, 51286–51289 RSC.
- S. Rudenja, A. Minko and D. Buchanan, Low-temperature deposition of stoichiometric HfO2 on silicon: analysis and quantification of the HfO2/Si interface from electrical and XPS measurements, Appl. Surf. Sci., 2010, 257, 17–21 CrossRef.
- H. Xie, Q. Liu, Y. Li, H. Lv, M. Wang, X. Liu, H. Sun, X. Yang, S. Long and S. Liu, Nitrogen-induced improvement of resistive switching uniformity in a HfO2-based RRAM device, Semicond. Sci. Technol., 2012, 27, 125008 CrossRef.
- S. K. Gupta, N. Pathak, P. Ghosh and R. Kadam, On the photophysics and speciation of actinide ion in MgAl2O4 spinel using photoluminescence spectroscopy and first principle calculation: a case study with uranium, J. Alloys Compd., 2017, 695, 337–343 CrossRef.
- S. K. Gupta, A. Yadav, S. Nigam, S. Jha, C. Mazumder, D. Bhattacharya and S. Thulasidas, Speciation and site occupancy of uranium in strontium orthosilicate by photoluminescence and X-ray absorption spectroscopy: a combined experimental and theoretical approach, Spectrochim. Acta, Part A, 2015, 151, 453–458 CrossRef PubMed.
- A. Rout, B. S. Panigrahi, C. Nayak, D. Bhattacharyya and S. N. Jha, Uranium speciation and its site occupancy in alkaline-earth borophosphates, J. Am. Ceram. Soc., 2017, 100, 2921–2931 CrossRef.
- D. J. Gregg, Y. Zhang, Z. Zhang, I. Karatchevtseva, M. G. Blackford, G. Triani, G. R. Lumpkin and E. R. Vance, Crystal chemistry and structures of uranium-doped gadolinium zirconates, J. Nucl. Mater., 2013, 438, 144–153 CrossRef.
- M. James, M. L. Carter, Z. Zhang, Y. Zhang, K. S. Wallwork, M. Avdeev and E. R. Vance, Crystal chemistry and structures of (Ca, U) titanate pyrochlores, J. Am. Ceram. Soc., 2010, 93, 3464–3473 CrossRef.
- E. Hashem, G. Lorusso, M. Evangelisti, T. McCabe, C. Schulzke, J. A. Platts and R. J. Baker, Fingerprinting the oxidation state of U (IV) by emission spectroscopy, Dalton Trans., 2013, 42, 14677–14680 RSC.
- E. Hashem, A. N. Swinburne, C. Schulzke, R. C. Evans, J. A. Platts, A. Kerridge, L. S. Natrajan and R. J. Baker, Emission spectroscopy of uranium (IV) compounds: a combined synthetic, spectroscopic and computational study, RSC Adv., 2013, 3, 4350–4361 RSC.
- L. S. Natrajan, The first structural and spectroscopic study of a paramagnetic 5f DO3A complex, Dalton Trans., 2012, 41, 13167–13172 RSC.
- S. K. Gupta, N. Pathak, R. Gupta, S. Thulasidas and V. Natarajan, Probing the oxidation state and coordination geometry of uranium ion in SrZrO3 perovskite, J. Mol. Struct., 2014, 1068, 204–209 CrossRef.
- M. Sahu, S. K. Gupta, D. Jain, M. Saxena and R. Kadam, Solid state speciation of uranium and its local structure in Sr2CeO4 using photoluminescence spectroscopy, Spectrochim. Acta, Part A, 2018, 195, 113–119 CrossRef PubMed.
- G. Liu, N. P. Deifel, C. L. Cahill, V. V. Zhurov and A. A. Pinkerton, Charge transfer vibronic transitions in uranyl tetrachloride compounds, J. Phys. Chem. A, 2012, 116, 855–864 CrossRef PubMed.
- G. Liu and M. Jensen, Theoretical analysis of optical spectra of uranyl in complexes, Chem. Phys. Lett., 2010, 499, 178–181 CrossRef.
- D. H. Metcalf, S. Dai, G. Del Cul and L. Toth, Luminescence spectra of single crystals of Cs2ZrCl6:UO2Cl42− at low temperatures. Vibronic structure of UO22+ doped in a cubic host, Inorg. Chem., 1995, 34, 5573–5577 CrossRef.
-
S. K. Gupta, N. Pathak, M. Mohapatra and R. Kadam, in Role of lattice indeed plays a major role in uranium electronic structure: a case study with pyrochlore, spinel and perovskite, Proceedings of the thirteenth DAE-BRNS nuclear and radiochemistry symposium, India, INIS, India, 2017.
Footnote |
† Electronic supplementary information (ESI) available. See DOI: 10.1039/c8qm00266e |
|
This journal is © the Partner Organisations 2018 |
Click here to see how this site uses Cookies. View our privacy policy here.