DOI:
10.1039/C7RA09647J
(Paper)
RSC Adv., 2018,
8, 3803-3808
Self-exfoliation of 2D covalent organic frameworks: morphology transformation induced by solvent polarity†
Received
30th August 2017
, Accepted 9th January 2018
First published on 19th January 2018
Abstract
Recently, covalent organic nanosheets (CONs) have emerged as functional two-dimensional (2D) materials for versatile applications. Strong interaction among layers and the instability of borate ester in moisture are the major hurdles to obtain few layered boron-containing CONs by exfoliation of their bulk counterparts. In this paper, we report a facile approach for preparation of few layered borate ester-containing CONs based on electrostatic repulsion of ions. We incorporated organic ionic groups into porous covalent organic frameworks (COFs) and it has been proved that the COFs with quaternary ammonium group could self-exfoliate into few layered ionic covalent organic nanosheets (iCONs) in polar organic solvents. Interestingly, the morphology of the iCOFs-A could be changed from a multilayered aggregation to nanocapsules, or 2D sheets when solvents with different polarity were used. In contrast, non-ionic covalent organic frameworks COFs-B could not self-exfoliate in various solvents. In addition, the self-exfoliated nanosheets could be used to fabricate uniform thin films on SiO2 wafer and the film exhibited explicit optical and electrical properties.
Introduction
Inspired by the discovery of graphene,1 efforts to fabricate two-dimensional (2D) polymers have never stopped.2–9 By far, one of the most investigated class of 2D polymers is covalent organic frameworks (COFs) due to their advantages, such as large surface areas, tunable properties and functionality. In general, COFs can be classified into three categories: boron-containing,10–18 triazine-based,19–23 and imine-based COFs.24–27 Among these COFs, the aromatic boron-containing COFs are particularly promising materials for many applications, such as organic electronics,28–32 in part because they incorporate two distinct molecular components that allow their composition to be varied independently. However, the insoluble characteristics seriously hinder their characterization and processing for practical applications.
In recent years, considerable efforts have been made to synthesize covalent organic nanosheets (CONs) by exfoliating their bulk counterparts through conventional exfoliation methods such as ultrasonication,33,34 mechanical delamination35 and chemically delamination.36 However, these exfoliation approaches unavoidably cause some defects in structure of the materials, which obviously affect their properties and limit their applications. Therefore, it is highly desired to develop a facile approach for preparation of 2D CONs in solution.
It is well known that the interaction of the polymer chains with inbuilt ionic character will be decreased due to electrostatic repulsion. On this account, it is possible to develop a self-exfoliating approach for preparation of 2D CONs. For example, self-exfoliation of imine-based COFs with positive charges was reported in water without external stimuli.37 However, it should be noticed that borate ester is not stable in moisture38 or in water,39 thus, exfoliation in water or aqueous solution is not feasible for the boron-based COFs. Actually, it is quite a challenge to achieve self-exfoliation of 2D boron-containing COFs in solution.
The COFs of boronate esters, the most investigated materials among the family of COFs, are prepared by condensation of boronic acids and catechols. However, it is well known that catechols are prone to oxidation and have a poor solubility in most organic solvents. We presumed that the in situ formation of catechols from protected catechols could prevent oxidation of catechols, and moreover, the protected catechols would possess higher solubility than the catechols in organic solvents. On the other hand, it is not easy to introduce ionic groups into the COFs of boronate esters because borate ester is not stable towards water or moisture. Fortunately, we found that BF3·OEt2 as a Lewis acid catalyst could not only catalyze the formation of boron-containing COFs,40,41 but also coordinate with nitrogen atoms to form organic ion pairs. Therefore, we successfully prepared the iCOFs of boronate esters by one-pot method using BF3·OEt2 as catalyst. This one-pot process is a more convenient way for preparation of boronate iCOFs. The results demonstrated that few-layered ionic covalent organic nanosheets (iCONs) were obtained via two steps, formation of the iCOFs in low polar organic solvents and their self-exfoliation in high polar organic solvents. Moreover, the iCONs could be well dispersed in solution and a uniform thin film of the exfoliated iCONs was easily fabricated on SiO2 wafer, which exhibited explicit optical and electrical properties. To the best of our knowledge, this is the first report on the self-exfoliation of boron-containing COFs in solution.
Experimental
General
All solvents were dried following the standard procedures before use. Unless otherwise indicated, all starting materials were obtained from commercial suppliers and used without further purification.
Synthesis of compound 1
A solution of 4-bromobenzaldehyde (5.55 g, 30 mmol) and veratrole (2.8 g, 20 mmol) in chloroform (20 mL) was added dropwise to a freshly prepared Eaton's reagent (7.7 wt% phosphorus pentoxide solution in methanesulfonic acid) in an ice-bath. After addition, the suspension was stirred for 2 h under reflux and then cooled to room temperature. Then DDQ (1 equiv.) was added to the mixture and the reaction was heated at 40 °C for 2 h. Subsequently, the organic mixture was poured into iced water and neutralized with 2 M NaOH. After extraction with chloroform, the solvent was concentrated under reduced pressure and the residue was recrystallized from a methanol–chloroform mixture to give 1 with a yield of 25%. 1H NMR (300 MHz, CDCl3): δ 7.74 (d, 4H), 7.37 (d, 4H), 6.75 (s, 4H), 3.76 (s, 12H). 13C NMR (100 MHz, CDCl3): δ 149.2, 138.7, 132.8, 132.1, 131.9, 125.7, 121.8, 103.6, 55.7.
Synthesis of compound 2
Compound 1 (1.5 g, 2.47 mmol) was suspended in 47% HBr (aq) (200 mL) and HOAc (200 mL) and the suspension was deoxygenated by passing a stream of Ar through the suspension for 5 min. Then, the mixture was refluxed overnight under Ar. After 24 h, the dark solution was allowed to cool slowly under Ar. The product as a crystalline solid was filtered, washed with water and dichloromethane in sequence. Since it is somewhat sensitive to air, the solid should be stored under Ar. Compound 2 was obtained in a yield of 83.3% (1.15 g). 1H NMR (300 MHz, d6-DMSO): δ 7.80 (d, 4H), 7.34 (d, 4H), 6.62 (s, 4H). 13C NMR (100 MHz, d6-DMSO): δ 146.4, 139.3, 133.2, 131.7, 129.1, 124.9, 120.5, 106.1.
Synthesis of compound C2
1 g (1.8 mmol) of compound 2 was dissolved in 75 mL of benzene. To this solution was added 15 mL of acetone and 300 mg of p-toluenesulfonic acid. The solution was heated to reflux overnight in a Dean-Stark apparatus. After the collection of water ceased, the reaction solution was diluted with 200 mL of CH2Cl2 and washed with water (2 × 100 mL). The organic layers were dried over Na2SO4 and the solvent was removed by rotary evaporation. The residue was purified by column chromatography using silica gel with a mixture of petroleum ether and dichloromethane (1
:
1, v/v) as eluent to give C2. Compound C2 was obtained in a yield of 88% (1.0 g). 1H NMR (CDCl3, 300 MHz): δ 7.70 (d, 4H), 7.28 (d, 4H), 6.66 (s, 4H), 1.65 (s, 12H). 13C NMR (100 MHz, CDCl3): δ 147.3, 138.8, 132.9, 131.8, 128.3, 126.7, 121.5, 118.1, 100.8, 26.0.
Synthesis of compound A2
A Schlenk flask was charged with compound C2 (1.0 mmol), N-methyl piperazine (2.4 mmol), sodium tertbutoxide (2.8 mmol), tris(dibenzylideneacetone)dipalladium-(0) (0.02 mmol, 2 mol%), BINAP (0.06 mmol), and toluene (9 mL) under argon. The flask was immersed in an oil bath and heated to 80 °C with stirring until the starting material had been completely consumed as judged by GC analysis. The mixture was allowed to cool to room temperature, taken up in CHCl3 (150 mL), filtered, and concentrated. The crude product was then purified further by flash chromatography on silica gel with a mixture of CHCl3 and CH3OH (10
:
1, v/v) as eluent to give A2. 1H NMR (CDCl3, 300 MHz): δ 7.31 (d, 4H), 7.11 (d, 4H), 6.78 (s, 4H), 3.46 (m, 8H), 2.87 (m, 8H), 2.56 (s, 6H), 1.64 (s, 12H).
Synthesis of compound 3
A flask charged with palladium chloride (0.16 g, 0.9 mmol), 1,1-bis(diphenylphosphino)ferrocene (DPPF) (0.50 g, 0.9 mmol), potassium acetate (8.83 g, 90 mmol), and 4,4,5,5,4,4,5,5-octamethyl-[2,2]bi[[1,3,2]dioxaborolanyl] (8.38 g, 33 mmol) was flushed with nitrogen. DMSO (120 mL) and 1,3,5-tribromobenzene (3.15 g, 10 mmol) were then added. After being stirred at 80 °C for an appropriate period, the product was extracted with benzene, washed with water, and dried over anhydrous magnesium sulfate. Benzene was removed and the residue was purified by column chromatography using silica gel with a mixture of petroleum ether and dichloromethane (3
:
2, v/v) as eluent to give 3, yield: 87% (colorless needle crystal). 1H NMR (CDCl3, 300 MHz), δ (ppm): 1.33 (s, 36H), 8.36 (s, 3H). 13C NMR (100 MHz, CDCl3): δ 144.1, 83.7, 24.9.
Synthesis of compound B3
The synthesis of 1,3,5-benzenetriboronic acid was carried out by a modified method of a published procedure. To the solution of pinacol 1,3,5-benzenetriboronic ester S7 (1.0 g, 2.19 mmol) in THF (24 mL) and water (6 mL) sodium periodate (4.22 g, 19.71 mmol) was added. The cloudy white suspension was stirred overnight at room temperature. Hydrochloric acid (2 M, 0.5 mL) was added and the mixture stirred for another 24 hours. Methanol (100 mL) was added and the mixture was filtered to remove salts. The filtrate was concentrated by rotator evaporation and hydrochloric acid (1 M, 30 mL) was added. The mixture was stirred at room temperature for 2 hours and the resulting precipitate was collected by filtration and dried in vacuum to give triboronic acid compound B3 (0.44 g, 95%) as white solid. 1H NMR (300 MHz, d6-DMSO), δ (ppm): 8.20 (s, 3H), 7.86 (s, 6H). 13C NMR (100 MHz, CD3OD): δ (ppm) = 142.5.
Synthesis of iCOFs-A
Compound A2 (144 mg, 0.214 mmol) and compound B3 (30 mg, 0.143 mmol) were loaded into a 1 dram screw-cap vial and suspended in a mixture of mesitylene and dioxane (1
:
1, 40 mL). The dark-blue mixture was sonicated for 15 minutes. Boron trifluoride etherate (15 mL, 0.12 mmol) was added, and the mixture was sonicated for another 15 minutes. The dark, heterogeneous mixture was flash frozen in a liquid nitrogen bath. The ampoule neck was flame-sealed in air using a propane torch, which reduced the total length by 20–30 mm. After warming to room temperature the suspension was placed in a gravity convection oven at 120 °C for six days. The reaction was cooled to room temperature, the ampoule was broken at the scored neck and the mixture was poured onto qualitative filter paper and filtered under vacuum. The resulting dark solid was washed with anhydrous mesitylene and dioxane, and dried in air.
Synthesis of COFs-B
Compound C2 (144 mg, 0.214 mmol) and compound B3 (30 mg, 0.143 mmol) were loaded into a 1 dram screw-cap vial and suspended in a mixture of mesitylene and dioxane (1
:
1, 40 mL). The dark-blue mixture was sonicated for 15 minutes. Boron trifluoride etherate (15 mL, 0.12 mmol) was added, and the mixture was sonicated for another 15 minutes. The dark, heterogeneous mixture was flash frozen in a liquid nitrogen bath. The ampoule neck was flame-sealed in air using a propane torch, which reduced the total length by 20–30 mm. After warming to room temperature the suspension was placed in a gravity convection oven at 120 °C for six days. The reaction was cooled to room temperature, the ampoule was broken at the scored neck and the mixture was poured onto qualitative filter paper and filtered under vacuum. The resulting dark solid was washed with anhydrous CH3CN and dried in air.
Results and discussion
The anthracene-based ionic covalent organic frameworks iCOFs-A was solvothermally synthesized via condensation reaction between A2 (0.3 mmol) and B3 (0.2 mmol) (Fig. 1a) in a sealed Pyrex tube using dioxane/mesitylene in the ratio 1
:
1. To demonstrate the effect of ion pairs, we also synthesized the anthracene-based non-ionic covalent organic frameworks COFs-B via condensation reaction between C2 (0.3 mmol) and B3 (0.2 mmol) (Fig. 1a). Synthetic route of the A2, B3 and C2 was presented in Fig. S1.† Synthetic route of the A2, B3 and C2 was presented in Fig. S1.† In FTIR spectra of iCOFs-A and COFs-B, the appearance of the characteristic B–O and C–O stretching vibrations of the borate ester at 1375 cm−1 and 1231 cm−1 indicated the formation of condensation products (Fig. S2†). All the stretching and bending vibrations of the synthesized COFs were summarized in Table S1 and S2.† Moreover, compared 13C CP-MAS solid-state NMR spectra of iCOFs-A and COFs-B with that of A2 and C2, a very sharp peak at 20 ppm of the dimethyl group disappeared, which is a strong evidence for the formation of iCOFs-A and COFs-B (Fig. S3†). Since A2 contains N atoms and B3 does not, the C/N ratio of iCOFs-A can provide the information for the composition and the structure of the 2D polymer. Elemental analysis showed that the C/N ratio of iCOFs-A was 10
:
1 which was consistent with the theoretical value (Fig. S4†). In addition, TGA profiles of the iCOFs-A showed that the decomposition temperature was ∼237 °C (Fig. S5†).
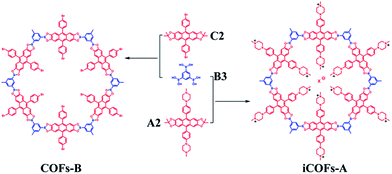 |
| Fig. 1 Synthesis of iCOFs-A and COFs-B. | |
The PXRD pattern of iCOFs-A displayed two intense peaks at 2θ = 7.27° and 7.79°, which correspond to the pore diameters of iCOFs-A (Fig. 2a and c). The broad peaks at 2θ = 17.6° and 20.95° were assigned to the interlayer π–π stacking distance in iCOFs-A measured to be ∼4.3–5.0 Å (Fig. 2b and d), which was larger than that (3.7 Å) of COFs-B (Fig. S6†). This could be attributed to the incorporation of the positively charged nitrogen-atoms, which decreased the π–π interaction and increased the layer spacing due to electrostatic repulsion. This result provides a positive feedback that it may be possible to achieve self-exfoliation of iCOFs in solution. Inspired by this result, we have successfully prepared ionic covalent organic nanosheets iCONs-A by self-exfoliation of iCOFs-A in polar solvents.
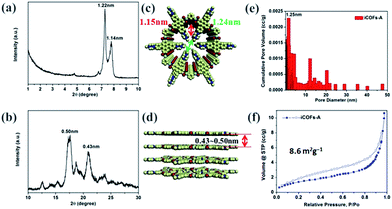 |
| Fig. 2 (a, b) Experimental PXRD patterns of iCOFs-A. (c, d). Simulated PXRD pattern of iCOFs-A in eclipsed mode. (e) Pore size distribution of iCOFs-A. (f) Nitrogen sorption isotherms for iCOFs-A at 77 K. | |
Permanent porosity of iCOFs-A and COFs-B were verified by N2 adsorption isotherms of the activated samples at 77 K, the results indicated that both of them followed type-II reversible adsorption isotherm. The Brunauer–Emmett–Teller (BET) surface area of iCOFs-A and COFs-B were calculated to be 8.6 m2 g−1 (Fig. 2f) and 16 m2 g−1 (Fig. S7†), respectively. The lower surface area of iCOFs-A could be ascribed to poor layer stacking, small pore diameter, and pore blocking by the counter anions.42 Pore size distribution was calculated on the basis of nonlocal density function theory (NLDFT). The pore diameters of iCOFs-A and COFs-B were calculated to be 1.25 nm (Fig. 2e) and 1.71 nm (Fig. S7†). Due to the lack of proper channelled pore structure and the pore blocking by counter anions, the size distribution of iCOFs-A was not as sharp as that in COFs-B.
It is well known that ionic organic molecules prefer to dissolve in polar solvents rather than nonpolar solvents due to the difference in solvation ability of the ionic molecules in the solvents. To explore self-exfoliation of iCOFs-A in solution, we performed dialysis of iCOFs-A in organic solvents with different polarities.
The effect of solvent polarity on the self-exfoliation of the iCOFs-A was tracked by a combination of scanning electron microscopy (SEM), transmission electron microscopy (TEM), atomic force microscopy (AFM) and light-scattering (Fig. 3). First, iCOFs-A was dispersed in dioxane/mesitylene (1/1) and characterized by electron microscopy. TEM images (Fig. 3a) and SEM images (Fig. 3d) showed that iCOFs-A possessed a layer-by-layer structure with an average diameter of 3.5 ± 0.5 μm, which consistent with the results of dynamic light scattering (Fig. 3g). Then, the iCOFs-A dispersed in dioxane/mesitylene was dialyzed respectively in dioxane and acetonitrile through a dialysis membrane with a molecular weight cut-off (MWCO) of 1000. Interestingly, the results revealed that hollow nanospheres were formed in dioxane with an average diameter of 250 ± 50 nm (Fig. 3b, e and h) and an average thickness of 7.5 ± 0.5 nm (Fig. S8†), whereas nanosheets were obtained in acetonitrile with an average diameter of 20 ± 5 μm (Fig. 3c, f and i) and an average thickness of 3.5 ± 0.5 nm (Fig. S8†). According to the recent reports,43–45 polymers tend to form nanocapsules in poor solvents, whereas they prefer forming 2D films in good solvents. In our case (Fig. 4), since the mixture of dioxane/mesitylene (1/1) with low solvation power is not a good solvent for iCOFs-A, the multilayered iCOFs-A was obtained. When dioxane/mesitylene was replaced by dioxane, solvation of the iCOFs-A with dioxane led to a decrease in the interaction of two layers and an increase in the layer spacing, and finally, the bulk iCOFs-A were exfoliated into thin iCOFs-A sheets with a few layers or even a monolayer. However, the thin iCOFs-A sheets tend to curl into a ball to reduce the Gibbs-energy,43 this can be attributed to the solvation power and polarity of dioxane, both of which are not large enough to stabilize the peeled layers. Compared with dioxane, acetonitrile possesses stronger solvation ability, so not only the bulk iCOFs-A can be exfoliated by acetonitrile, but also the stable few layered iCONs-A sheets can be obtained in acetonitrile.
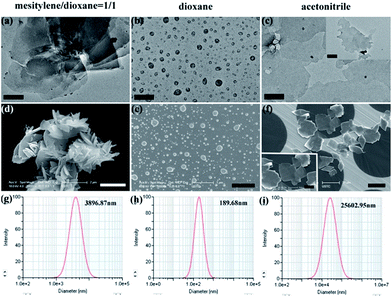 |
| Fig. 3 Monitoring the morphological transformation from iCOFs-A to iCONs-A by TEM (a–c), SEM (d–f) and DLS studies (g–i). Scale bars: (a) 0.5 μm, (b) 0.5 μm, (c) 0.2 μm (inlet image: 2 μm), (d) 2 μm, (e) 1 μm, (f) 20 μm (inlet image: 10 μm). | |
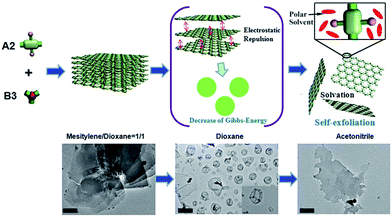 |
| Fig. 4 Schematic illustration of the formation of 2D ionic covalent organic nanosheets. Scale bars: 0.5 μm (left), 0.5 μm (middle), 2 μm (right). | |
The self-exfoliation experiments of iCOFs-A were performed in various organic solvents, but only a few of organic solvents with relatively strong polarity are effective for the self-exfoliation of iCOFs-A, including DMF, DMSO, CH3CN, and CH3OH (Fig. S11†). The weight and volume ratio between iCOFs-A and organic solvent for the self-exfoliation was o.5 mg mL−1. As a control experiment, we also performed dialysis of the COFs-B in solvents with different polarities, the results demonstrated that no change in morphology was observed (Fig. S9†), indicating no self-exfoliation occurred. Obviously, both electrostatic repulsion and solvation are important for the self-exfoliation of iCOFs-A. First, the electrostatic repulsion led to the weaker π–π interaction between the layers and the larger layer spacing, which are favorable for the intercalating of solvent. Then, the solvation of polar solvents with iCOFs-A resulted in their self-exfoliation. Therefore, the incorporation of ionic groups is of great significance for self-exfoliation of the COFs in solution.
Since few-layered nanosheets of the iCONs-A can easily be obtained from self-exfoliation of the iCOFs-A in polar solvents, we have successfully prepared uniform, free-standing CON thin films of iCONs-A (Fig. S10a and b†), indicating a significant impact on processing and applications of COFs. Moreover, we investigated the electrical conductivity and photoconductivity of the self-exfoliated nanosheets by casting a thin film of iCONs-A on SiO2 wafer. The micro-gap electrodes were fabricated by photolithography silicon wafer covered with a 300 nm thick SiO2 dielectric layer. The gold electrode pair was 50 μm long and 5 μm wide and the film thickness was about 50 μm. Based on the obtained data, smaller current was observed with iCONs-A46 which may be ascribed to the weaker stacking (Fig. S10d†). Then, on irradiation with visible light from a xenon lamp, the characteristics curve showed larger current, which illustrated the photoresponse of the film. In order to investigate the photocurrent response, we measured the transient photocurrent of the device at a bias voltage of 2 V (Fig. S10c†). The photosensitivity was found to be 3.86. Transient photocurrents were steady and reproducible during on–off cycles of the visible light irradiation. The photoresponse of iCONs-A was likely due to their π–π overlap among re-accumulated nanosheets (Fig. S10d†).47 We believe that it is possible to obtain nanosheets of iCOFs with excellent electroconductive and photoconductive properties by rationally designing composition and structure of the iCONs.
Conclusions
In conclusion, both the anthracene-based ionic covalent organic frameworks iCOFs-A and non-ionic covalent organic frameworks COFs-B were designed and synthesized and their self-exfoliation was investigated in different organic solvents. It was demonstrated that iCOFs-A possesses self-exfoliating property in organic solvents with high polarity, but COFs-B does not. The results indicated that the incorporation of ionic moiety into COFs is of great significance for self-exfoliation of the COFs in solution. Based on electrostatic repulsion and solvation, ionic covalent organic nanosheets iCONs-A can be obtained from self-exfoliation of iCOFs-A in organic solvents with high polarity. Moreover, few layered iCONs-A was used to prepare uniform thin films on SiO2, which exhibited explicit electroconductive and photoconductive properties. Therefore, this work not only provided a new approach for self-exfoliation of boron-based COFs, but also opened a new perspective for processing and applications of COFs materials.
Conflicts of interest
There are no conflicts to declare.
Acknowledgements
The authors are grateful for financial support from the National Natural Science Foundation of China (No. 21674101).
Notes and references
- K. S. Novoselov, A. K. Geim, S. V. Morozov, D. Jiang, Y. Zhang, S. V. Dubonos, I. V. Grigorieva and A. A. Firsov, Science, 2004, 306, 666–669 CrossRef CAS PubMed.
- J. Sakamoto, J. V. Heijst, O. Lukin and A. D. Schlüter, Angew. Chem., Int. Ed., 2009, 48, 1030–1069 CrossRef CAS PubMed.
- P. Kissel, R. Erni, W. B. Schweizer, M. D. Rossell, B. T. King, T. Bauer, S. Götzinger, A. D. Schlüter and J. Sakamoto, Nat. Chem., 2012, 4, 287–291 CrossRef CAS PubMed.
- R. Bhola, P. Payamyar, D. J. Murray, B. Kumar, A. J. Teator, M. U. Schmidt, S. M. Hammer, A. Saha, J. Sakamoto, A. D. Schlüter and B. T. King, J. Am. Chem. Soc., 2013, 135, 14134–14141 CrossRef CAS PubMed.
- P. Kissel, D. J. Murray, W. J. Wulftange, V. J. Catalano and B. T. King, Nat. Chem., 2014, 6, 774–778 CrossRef CAS PubMed.
- M. J. Kory, M. Wörle, T. Weber, P. Payamyar, S. W. van de Poll, J. Dshemuchadse, N. Trapp and A. D. Schlüter, Nat. Chem., 2014, 6, 779–784 CrossRef CAS PubMed.
- X. D. Zhuang, Y. Y. Mai, D. Q. Wu, F. Zhang and X. L. Feng, Adv. Mater., 2015, 27, 403–427 CrossRef CAS PubMed.
- S. L. Cai, W. G. Zhang, R. N. Zuckermann, Z. T. Li, X. Zhao and Y. Liu, Adv. Mater., 2015, 27, 5762–5770 CrossRef CAS PubMed.
- W. Bai, Z. W. Jiang, A. E. Ribbe and S. Thayumanavan, Angew. Chem., Int. Ed., 2016, 55, 10707–10711 CrossRef CAS PubMed.
- A. P. CÔté, A. I. Benin, N. W. Ockwig, A. J. Matzger, M. O'Keeffe and O. M. Yaghi, Science, 2005, 310, 1166–1170 CrossRef PubMed.
- H. M. El-Kaderi, J. R. Hunt, J. L. Mendoza-Cortes, A. P. CÔté, R. E. Taylor, M. O'Keeffe and O. M. Yaghi, Science, 2007, 316, 268–272 CrossRef CAS PubMed.
- A. P. CÔté, H. M. El-Kaderi, H. Furukawa, J. R. Hunt and O. M. Yaghi, J. Am. Chem. Soc., 2007, 129, 12914–12915 CrossRef PubMed.
- R. W. Tilford, S. J. Mugavero III, P. J. Pellechia and J. J. Lavigne, Adv. Mater., 2008, 20, 2741–2746 CrossRef CAS PubMed.
- S. Wan, J. Guo, J. Kim, H. Ihee and D. Jiang, Angew. Chem., Int. Ed., 2008, 47, 8826–8830 CrossRef CAS PubMed.
- S. Wan, J. Guo, J. Kim, H. Ihee and D. Jiang, Angew. Chem., Int. Ed., 2009, 48, 5439–5442 CrossRef CAS PubMed.
- E. L. Spitler and W. R. Dichtel, Nat. Chem., 2010, 2, 672–677 CrossRef CAS PubMed.
- A. Nagai, Z. Guo, X. Feng, S. Jin, X. Chen, X. Ding and D. Jiang, Nat. Commun., 2011, 2, 536–543 CrossRef PubMed.
- D. N. Bunck and W. R. Dichtel, Angew. Chem., Int. Ed., 2012, 51, 1885–1889 CrossRef CAS PubMed.
- P. Kuhn, M. Antonietti and A. Thomas, Angew. Chem., Int. Ed., 2008, 47, 3450–3453 CrossRef CAS PubMed.
- R. Palkovits, M. Antonietti, P. Kuhn, A. Thomas and F. Schüth, Angew. Chem., Int. Ed., 2009, 48, 6909–6912 CrossRef CAS PubMed.
- M. J. Bojdys, J. Jeromenok, A. Thomas and M. Antonietti, Adv. Mater., 2010, 22, 2202–2205 CrossRef CAS PubMed.
- C. E. Chan-Thaw, A. Villa, P. Katekomol, D. Su, A. Thomas and L. Prati, Nano Lett., 2010, 10, 537–541 CrossRef CAS PubMed.
- C. E. Chan-Thaw, A. Villa, L. Prati and A. Thomas, Chem.–Eur. J., 2011, 17, 1052–1057 CrossRef CAS PubMed.
- F. J. Uribe-Romo, J. R. Hunt, H. Furukawa, C. Klock, M. O'Keeffe and O. M. Yaghi, J. Am. Chem. Soc., 2009, 131, 4570–4571 CrossRef CAS PubMed.
- F. J. Uribe-Romo, C. J. Doonan, H. Furukawa, K. Oisaki and O. M. Yaghi, J. Am. Chem. Soc., 2011, 133, 11478–11481 CrossRef CAS PubMed.
- S.-Y. Ding, J. Gao, Q. Wang, Y. Zhang, W.-G. Song, C.-Y. Su and W. Wang, J. Am. Chem. Soc., 2011, 133, 19816–19822 CrossRef CAS PubMed.
- S. Wan, F. Gandara, A. Asano, H. Furukawa, A. Saeki, S. K. Dey, L. Liao, M. W. Ambrogio, Y. Y. Botros, X. Duan, S. Seki, J. F. Stoddart and O. M. Yaghi, Chem. Mater., 2011, 23, 4094–4097 CrossRef CAS.
- S. Wan, J. Guo, J. Kim, H. Ihee and D. L. Jiang, Angew. Chem., Int. Ed., 2008, 47, 8826–8830 CrossRef CAS PubMed.
- S. Wan, F. Gandara, A. Asano, H. Furukawa, A. Saeki, S. K. Dey, L. Liao, M. W. Ambrogio, Y. Y. Botros, X. F. Duan, S. Seki, J. F. Stoddart and O. M. Yaghi, Chem. Mater., 2011, 23, 4094–4097 CrossRef CAS.
- X. S. Ding, J. Guo, X. Feng, Y. Honsho, J. D. Guo, S. Seki, P. Maitarad, A. Saeki, S. Nagase and D. L. Jiang, Angew. Chem., Int. Ed., 2011, 50, 1289–1293 CrossRef CAS PubMed.
- X. S. Ding, L. Chen, Y. Honsho, X. Feng, O. Saengsawang, J. D. Guo, A. Saeki, S. Seki, S. Irle, S. Nagase, V. Parasuk and D. L. Jiang, J. Am. Chem. Soc., 2011, 133, 14510–14513 CrossRef CAS PubMed.
- X. Feng, L. L. Liu, Y. Honsho, A. Saeki, S. Seki, S. Irle, Y. Dong, A. Nagai and D. L. Jiang, Angew. Chem., 2012, 124, 2672–2676 CrossRef.
- I. Berlanga, M. L. Ruiz-Gonzalez, J. M. Gonzalez-Calbet, J. L. G. Fierro, R. Mas-Balleste and F. Zamora, Small, 2011, 7, 1207–1211 CrossRef CAS PubMed.
- S. Chandra, S. Kandambeth, B. P. Biswal, B. Lukose, S. M. Kunjir, M. Chaudhary, R. Babarao, T. Heine and R. Banerjee, J. Am. Chem. Soc., 2013, 135, 17853–17861 CrossRef CAS PubMed.
- D. N. Bunck and W. R. Dichtel, J. Am. Chem. Soc., 2013, 135, 14952–14955 CrossRef CAS PubMed.
- M. A. Khayum, S. Kandambeth, S. Mitra, S. B. Nair, A. Das, S. S. Nagane, R. Mukherjee and R. Banerjee, Angew. Chem., Int. Ed., 2016, 55, 15604–15608 CrossRef CAS PubMed.
- S. Mitra, S. Kandambeth, B. P. Biswal, A. Khayum, C. K. Choudhury, M. Mehta, G. Kaur, S. Banerjee, A. Prabhune, S. Verma, S. Roy, U. K. Kharul and R. Banerjee, J. Am. Chem. Soc., 2016, 138, 2823–2828 CrossRef CAS PubMed.
- Y. Li and R. T. Yang, AIChE J., 2008, 54, 269–279 CrossRef CAS.
- L. M. Lanni, R. W. Tilford, M. Bharathy and J. J. Lavigne, J. Am. Chem. Soc., 2011, 133, 13975–13983 CrossRef CAS PubMed.
- E. L. Spitler and W. R. Dichtel, Nat. Chem., 2010, 2, 672–677 CrossRef CAS PubMed.
- E. L. Spitler, M. R. Giovino, S. L. White and W. R. Dichtel, Chem. Sci., 2011, 2, 1588–1593 RSC.
- H. Ma, B. L. Liu, B. Li, L. M. Zhang, Y. G. Li, H. Q. Tan, H. Y. Zang and G. S. Zhu, J. Am. Chem. Soc., 2016, 138, 5897–5903 CrossRef CAS PubMed.
- K. Baek, I. Hwang, I. Roy, D. Shetty and K. Kim, Acc. Chem. Res., 2015, 48, 2221–2229 CrossRef CAS PubMed.
- J. Kim, K. Baek, D. Shetty, N. Selvapalam, G. Yun, N. H. Kim, Y. H. Ko, K. M. Park, I. Hwang and K. Kim, Angew. Chem., Int. Ed., 2015, 54, 2693–2697 CrossRef CAS PubMed.
- R. K. Shukla and K. K. Raina, Int. J. Mod. Phys. B, 2009, 23, 5075–5083 CrossRef CAS.
- S. Wan, J. Guo, J. Kim, H. Ihee and D. L. Jiang, Angew. Chem., Int. Ed., 2009, 48, 5439–5442 CrossRef CAS PubMed.
- X. J. Ma, Y. B. Zhang, Y. F. Zhang, C. Peng, Y. Che and J. C. Zhao, Adv. Mater., 2015, 27, 7746–7751 CrossRef CAS PubMed.
Footnote |
† Electronic supplementary information (ESI) available: 1H NMR, 13C NMR, FT-IR, PXRD, TGA, SEM, TEM, AFM and element analysis. See DOI: 10.1039/c7ra09647j |
|
This journal is © The Royal Society of Chemistry 2018 |
Click here to see how this site uses Cookies. View our privacy policy here.