DOI:
10.1039/C7RA11811B
(Paper)
RSC Adv., 2018,
8, 8950-8960
Adsorption of Cd2+ by an ion-imprinted thiol-functionalized polymer in competition with heavy metal ions and organic acids
Received
26th October 2017
, Accepted 12th February 2018
First published on 28th February 2018
Abstract
The simultaneous presence of heavy metals and organic acids in nature and wastewaters and their competition for adsorption sites determine the migration, transformation and fate of pollutants in the environment. A Cd2+-ion-imprinted polymer (Cd2+-IIP) with a thiol-functional group was hydrothermally synthesized by a surface imprinting technique combined with ultrasonic heating for selective adsorption of Cd2+ from wastewaters. The adsorbent was characterized by SEM, EDS, XPS, BET and FT-IR measurements. The experimental results concerning Cd2+ adsorption from single-, binary-, ternary- and quaternary-metal aqueous solutions containing Cu2+, Ni2+ and Zn2+ revealed high selectivity. In binary-metal solutions, relative selectivity coefficients for Cd2+ in respect to Cd2+/Cu2+, Cd2+/Ni2+, and Cd2+/Zn2+ were as high as 3.74, 5.73 and 4.15, respectively. In multi-metal solutions, competing heavy metal ions had little effect on the adsorption of Cd2+ attributed to the high selectivity of Cd2+-IIP towards Cd2+ determined by its coordination geometry. The effect of low-molecular weight organic acids on the Cd2+ adsorption was also studied and the results showed that the presence of tartaric, citric and oxalic acids as admixtures in Cd2+ aqueous solutions noticeably reduced the cation adsorption in a wide range of concentrations with the minor exception of low contents of citric and tartaric acids slightly improving adsorption.
Introduction
Cadmium, known as one of the most toxic heavy metals, brings damage to living organisms and humans even at low concentrations,1–3 resulting in kidney damage, impairment of enzymes, disruption of calcium metabolism, and changes in cell membrane permeability.4 The upper reaches of the Beijiang River in Guangdong Province have been experiencing the problem of cadmium pollution for nearly half a century due to the exploitation of mines, threatening the water supply in downstream cities. In polluted natural waters, cadmium almost never occurs alone but is accompanied with other heavy metals (Cu2+, Zn2+, Ni2+, Pb2+, Cr3+/Cr(VI), Mn2+, Fe2+/Fe3+) and organic substances (grease, oil and organic acids), competing with adsorption of the target cation.5 Hence, the efficient elimination and separation of cadmium from its composite wastewater is urgently required. The objective of this study is to explore a suitable adsorbent to remove cadmium form its composite wastewater. Attention towards adsorption is paid due to its simplicity, cost efficiency and the removal performance of cadmium at low concentrations,3,6–9 although other technologies including electrochemical,10 membrane filtration,11 ion-exchange,12 and selective precipitation13 are also used for the removal of cadmium from wastewater.
In practical applications, if adsorbents have an ability to adsorb the target pollutants from complex pollutant mixtures selectively will be better. Ion imprinting technology (IIP) is used to manufacture polymeric adsorbents with improved binding selectivity towards heavy metal ions.14 The selectivity of these adsorbents is achieved by the choice of specific ligands providing coordination geometry and coordination numbers suitable for the adsorbed ions, their charges and sizes.15 Surface ion imprinted technique is one of the most promising synthetic methods of IIP adsorbents, having advantages in simplicity, convenient preparation, and high selectivity.16 Recently, a few IIP adsorbents have been produced combining the surface imprinting technique with the sol–gel process, immobilizing the functional group on the surface of adsorbent material for selective removal of heavy metals from aqueous solutions.17–19 For example, Li et al.20 synthesized a high-selectivity 3-thiocyanatopropyltriethoxysilane (TCPTS)-based Cd2+-ion imprinted material IIP-TCPTS/SiO2 attached to the surface of silica gel particles. IIP-TCPTS/SiO2 showed a higher adsorption capacity and selectivity for Cd2+ ion than Cd2+ non-imprinted polymer. Singh et al.21 used Cd2+-ion imprinted phenol-formaldehyde-Cd2+-2-(p-sulphophenylazo)-1,8-dihydroxynaphthalene-3,6-disulphonate (PF–Cd2+–SPANDS) for selective adsorption of Cd2+ from aqueous solutions: the relative selectivities for Cd2+ in the presence of competing heavy metal cations comprised the row of descending order Zn2+ > Hg2+ > Cu2+.
In recent years, consideration attention has been devoted to study the coadsorption behavior of heavy metal ions and organic pollutants.22 Low molecule weight organic acids, a typical representative of organic contaminants, mainly come from the decomposition of organisms and organic matter, the response and secretion of plant roots, the metabolic synthesis of microorganisms and the emission from human activities.23,24 Oxalic acid, citric acid and tartaric acid are the most common three low molecule weight organic acids and they can react with heavy metals by complexation, ion exchange and adsorption, thus influencing the adsorption behavior of adsorbent to heavy metal ions.25 The effect of organic acids on the adsorption of heavy metals at the non-ionic imprinted adsorbents was extensively studied,26–28 although the ion-imprinted materials received less attention. To our best knowledge, the publications on Cd2+ ion-imprinted thiol-functional silica-based polymer for selective removal Cd2+ in consideration of both co-exist heavy metal ions (Cu2+, Ni2+ and Zn2+) and organic acids (oxalic acid, citric acid and tartaric acid) still remain rare. The fully understanding the interactions between ion-imprinted adsorption material and co-exist heavy metals/low molecule weight organic acids will be good for the evaluation of behavior and effects of ion-imprinted adsorbent in its practical application.
The present study considers a synthesized thiol-functional Cd2+-imprinted silica-based polymer Cd2+-IIP for Cd2+ adsorption competing Cu2+, Ni2+ and Zn2+ cations together with organic acids – oxalic, citric and tartaric. The gradient separation and removal of target Cd2+-ion were studied determining the factors of synergistic and inhibitory effects of the above-mentioned admixtures. An ultrasonic-assisted hydrothermal method combined with surface imprinting technique was used to prepare the Cd2+-IIP adsorbent. Characterization of the adsorption performance and selectivity were studied in detail.
Materials and methods
Reagents
Silica gel (80–100 mesh) was obtained from Qingdao Ocean Chemical Co., China. 3-Mercaptopropyltrimethoxysilane (MPTS) was provided by Shanghai Macklin Biochemical Co., Ltd., China. All the other reagents were of analytical grade and purchased from ANPEL Laboratory Technologies Inc., China.
Preparation of Cd2+-IIP adsorbent
Silica gel in amount of 8.00 g was mixed with 60 mL of 33% methanesulfonic acid and refluxed under stirring for 8 h in order to activate the silica gel surface. The solid product was recovered by filtration, washed with distilled water to the neutral reaction, and dried under vacuum at 70 °C for 12 h. The Cd-template solution was prepared as follows: 3.08 g of Cd(NO3)2·4H2O were dissolved in 60 mL of methanol under stirring and heating at 40 °C for 20 min, and the solution was mixed with 4 mL of MPTS reacting for 1 h under ultrasonic heating at 60 °C. After that, 6.00 g of activated silica gel was added to the solution, sealed in a 100 mL Teflon-lined stainless steel autoclave and maintained at 120 °C for 24 h. Subsequently, 4 mL of epichlorohydrin were added to the mixture, which was then heated at 60 °C for 2 h. The product was filtered, washed with ethanol, HCl and distilled water to neutral pH, and dried at 60 °C for 24 h in vacuum. The final product of Cd2+-IIP was stored in the desiccator. The chemical reaction outline of preparation processes is shown in Fig. 1.
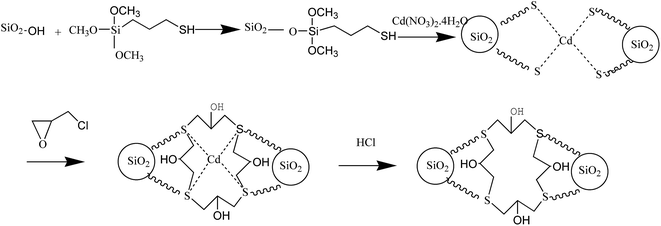 |
| Fig. 1 Outline of Cd2+-IIP adsorbent synthesis. | |
Analytical instruments
The surface morphology of the imprinted adsorbents was examined by scanning electron microscopy (SEM, MERLIN, Carl Zeiss AG, Germany) at the desired magnification, and the equipped energy dispersal X-ray spectroscopy (EDS). Fourier transform infrared (FT-IR) spectroscopy within 4000–400 cm−1 wavelength using KBr pellets at 2 cm−1 resolution was carried out using a Nexus Por Euro FT-IR spectrometer (Thermo Nicolet, USA). Surface areas were was carried out using NOVA3200e Brunauer–Emmett–Teller (BET) surface area analyzer (Micromeritics, USA). Surface composition of the adsorbent samples was analyzed using X-ray photoelectron spectroscopy (XPS, Kratos Axis Ulra DLD, UK). The concentrations of Cd2+, Cu2+, Ni2+ and Zn2+ in aqueous solutions were measured using the AA-6300C flame atomic absorption spectrometer (FAAS, Shimadzu, Japan). Concentrations of organic acids were determined using TOC-VCPN total organic carbon analyzer (TOC, Shimadzu, Japan).
Adsorption experiments
The adsorption experiments were carried out in the batch mode. Certain amount of Cd2+-IIP adsorbent was placed to a 100 mL beaker containing aqueous solutions of Cd2+, Cu2+, Ni2+ and Zn2+ ions in various combinations. After adsorption for 4 h at 25 °C, the solutions were filtered through the 0.45 μm polypropylene injection filters and the filtrate was analyzed using FAAS. The initial molar concentration ratios of heavy metal ions were set at unity for all the binary-, ternary- and quaternary-metal solutions. The solutions were adjusted to the desired pH by adding sodium hydroxide or nitric acid solutions.
To determine the adsorption capacity of Cd2+-IIP adsorbent in respect to organic acids, experiments were conducted with various, from 1 to 100 mg L−1, concentrations of the adsorbates, organic acids. The adsorption mixtures were equilibrated at pH 7.00 for 4 h. The data obtained in the experiments were used for follow-up discussion. In experiments targeting the impact of organic acids on Cd2+ adsorption, the adsorption of Cd2+ was studied at its initial concentration of 10 mg L−1, having organic acids dissolved in concentrations of 1–100 mg L−1.
Calculations
The adsorption capacity of Cd2+-IIP adsorbent was calculated by eqn (1):where Q represents the adsorption capacity, mmol g−1 or mg g−1; C0 and Ce are the initial and equilibrium concentrations of adsorbates, mmol L−1 or mg L−1, respectively; V is the volume of the solution sample, L; W is the mass of used adsorbent, g.
The linearized forms of Langmuir29 and Freundlich30 isotherms are expressed by eqn (2) and (3), respectively:
|
Ce/Qe = Ce/Qmax + 1/(QmaxKL)
| (2) |
|
log Qe = (1/n)log Ce + ln KF
| (3) |
where
Qe is the adsorption capacity at equilibrium, mmol g
−1,
Qmax is the maximum amount of adsorption, mmol g
−1,
KL is the adsorption equilibrium constant, L mg
−1.
KF is the constant representing the adsorption capacity and
n is the constant depicting the adsorption intensity.
The distribution and selectivity coefficients of Cd2+ with respect to Cu2+, Ni2+ and Zn2+ can be obtained from the equilibrium binding data according to eqn (4) and (5):2
where
Kd represents the distribution coefficient;
k is the selectivity coefficient, and X
2+ represents competing ions of Cu
2+, Ni
2+ and Zn
2+.
Results and discussion
Characterization of Cd2+-IIP adsorbent
SEM study. As displayed in Fig. 2(a and b), the surface of activated silica gel was smooth, while separate aggregates were visible on the surface of Cd2+-IIP, Fig. 2(c and d), which left the three-dimensional network structure of silica gel unchanged as a result of surface imprinting. Compared with silica gel, the surface of Cd2+-IIP was fluffier and rougher. Obviously, irregular particles are seen in Cd2+-IIP, which might be the functional monomers that could provide sufficient recognition sites for chelating heavy metal ions. Besides, holes also appeared in Cd2+-IIP, the structure and size of which would determine the ion radius and type of the targeted heavy metal ions.
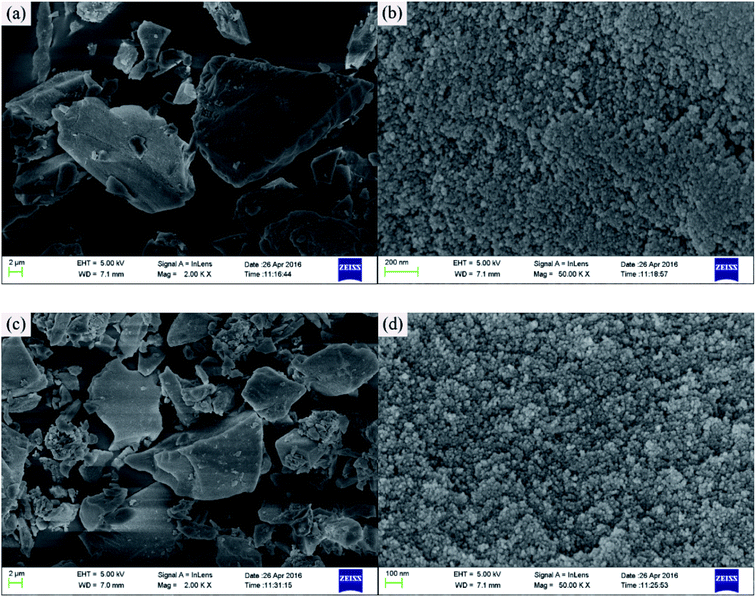 |
| Fig. 2 SEM photographs of activated silica gel (a, b) and Cd2+-IIP adsorbent (c, d). | |
EDS study. As shown in Fig. 3, the main elements in both activated silica gel and the Cd2+-IIP adsorbent were silica, oxygen and carbon, to which sulfur was added as a result of thiol imprinting. The sum contents of oxygen and carbon in Cd2+-IIP increased from 28.41 to 57.84%, respectively, the share of silica thus expectedly decreased. Since sulfur-containing functional groups had the ability to chelate heavy metal ions, so the increment in sulfur content would improve the adsorption capacity of Cd2+-IIP.
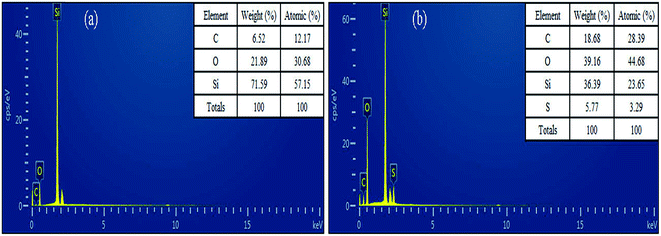 |
| Fig. 3 EDS analyses of activated silica gel (a) and Cd2+-IIP adsorbent (b). | |
FT-IR study. FT-IR spectra of silica gel and Cd2+-IIP are shown in Fig. 4. The peaks at 3454 and 1639 cm−1 correspond to the vibrations of
Si–OH and –OH in physisorbed water, respectively.31 The peak at 471 cm−1 was assigned to
Si–O–Si
stretching vibration,31 thus confirming the occurrence of silica matrices in the raw material. Changes in the FT-IR spectra were observed in Cd2+-IIP at the wavenumbers of 2931 and 2555 cm−1 attributed to the stretching vibration of –CH2 and –SH groups, respectively: band characteristic of –SH are known to fit into 2600–2450 cm−1.32 A broad absorption peak appeared at 1101 cm−1 corresponds to siloxane vibration of (SiO)n.33 The observed peaks consistently revealed MPTS successfully grafted onto the surface of silica gel in the imprinting processes.
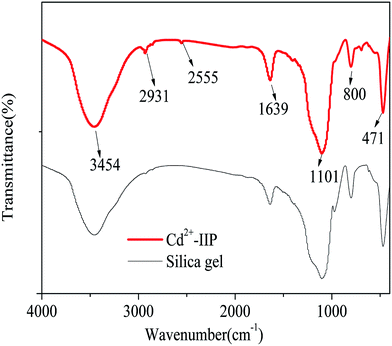 |
| Fig. 4 FT-IR spectra of Cd2+-IIP adsorbent and silica gel. | |
Surface area and pore size analysis. As shown in Fig. 5(a), the N2 adsorption–desorption isotherm of Cd2+-IIP can be categorized as type IV, meaning that Cd2+-IIP was a mesoporous structure adsorbent. The surface area of Cd2+-IIP calculated from Brunauer–Emmett–Teller (BET) was 197.6 m2 g−1 which was due to the specific recognition cavities for Cd2+ ions created on the sorbent surface. According to Fig. 5(b), the average pore diameter of Cd2+-IIP was 10.2 nm which could be obtained from Barrett–Joyner–Halenda (BJH) model. The pore size distributions of Cd2+-IIP was also mainly in the scope of mesopores with 2–50 nm.
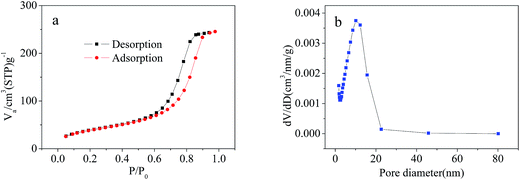 |
| Fig. 5 N2 adsorption–desorption isotherms of Cd2+-IIP at 77.3 K (a) and pore-size distribution curve of Cd2+-IIP (b). | |
XPS study. The full scan XPS spectrums showed the presence of Si at the binding energies of 156.85 eV and 105.7 eV for silica gel (Fig. 6(a)), and 164.6 eV and 105.7 eV (Fig. 6(b)) for Cd2+-IIP, respectively. The element of O could also be seen at the binding energy of 26.4 eV for silica gel and 29.1 eV for Cd2+-IIP, respectively. After modification, the elements of C and S were appeared in the XPS spectra of Cd2+-IIP, indicating the successful introduction MPTS onto silica gel. The content of S obtained from XPS study was 5.92% which was close to the results of EDS study. Herein, the theoretical content of thiol group was 1.85 mmol g−1, and the amount of MPTS anchored onto silica surface was about 36.3%.
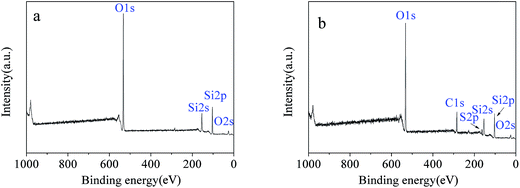 |
| Fig. 6 XPS survey spectra of silical gel and Cd2+-IIP. | |
Adsorption of Cd2+ at Cd2+-IIP: effect of pH and competing heavy metals
Adsorption of heavy metals from single-metal solutions. pH as a factor controlling the surface charge of the adsorbent and the ionization degree of the heavy metal ions is the most important factor of adsorption.34,35 Its study in the present research was realized within the pH range from 2.0 to 6.0 to avoid precipitation of heavy metal ions at higher values. The effect of pH on the adsorption capacity of Cd2+-IIP with regards to Cd2+, Cu2+, Ni2+ and Zn2+ in single-ion solutions is given in Fig. 7(a). It can be seen that the adsorption capacity increased with the pH: at low pH the protonation of adsorption sites and the net positive charge of the surface hamper heavy metal ions from approaching the surface of Cd2+-IIP. The affinity of Cd2+-IIP towards the ions lined up in the order Cd2+ > Cu2+ > Zn2+ > Ni2+ with the one to Cd2+ substantially higher than the others attributed to the pre-designed matching of Cd2+-IIP for Cd2+. The cationic radii of Cd2+, Zn2+, Cu2+ and Ni2+ was 0.97, 0.74, 0.73 and 0.69 Å,36 respectively, which was almost unanimous with the above order. Zn and Cu have radii pretty close to each other thus making the difference for the single- and binary-metal solutions minimal with the potential error of measurement.
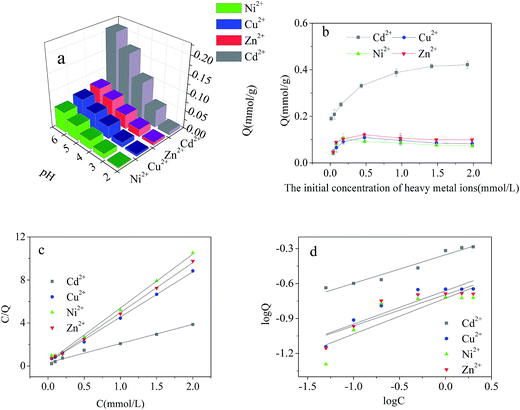 |
| Fig. 7 Effect of pH on the adsorption of Cd2+, Cu2+, Ni2+ and Zn2+ on Cd2+-IIP in single-metal solutions (a): C0 = 10 mg L−1, temperature = 25 °C, t = 4 h, the dosage of adsorbent = 0.02 g; dependence of Cd2+, Cu2+, Ni2+ and Zn2+ adsorption on the Cd2+-IIP adsorbent in single-metal solutions on the metals initial aqueous concentrations (b): pH = 5, C0 = 10–200 mg L−1, the dosage of adsorbent = 0.02 g, temperature = 25 °C, time = 4 h; the equilibrium isotherms: (c) the Langmuir model (d) the Freundlich model. | |
The effect of initial concentration of heavy metal ions on the adsorption capacity of Cd2+-IIP in single-metal solutions are given in Fig. 7(b). The selective character of the ion-imprinted Cd2+-IIP adsorbent in respect to Cd2+ was thus confirmed. For the purpose of fully understanding the adsorption behavior of Cd2+, Cu2+, Ni2+ and Zn2+ onto Cd2+-IIP in single system, Langmuir and Freundlich isotherms was used to fit the experimental data and the results were shown in Table 1 and Fig. 7(c, d). The high correlation coefficients (R2 ≥ 0.981) indicated that the adsorption of Cd2+, Cu2+, Ni2+ and Zn2+ onto Cd2+-IIP in compliance with Langmuir isotherm. The adsorption capacities of Cd2+-IIP towards Cd2+, Zn2+, Cu2+ and Ni2+ in Langmuir model were also close to the experimentally obtained values. According to the assumptions of Langmuir isotherm model,29 monolayer adsorption of Cd2+, Cu2+, Ni2+ and Zn2+ happened on the surface of Cd2+-IIP, and it was mainly chemical adsorption.
Table 1 Parameters of Langmuir and Freundlich isotherms for adsorption of Cd2+, Cu2+, Ni2+ and Zn2+ on Cd2+-IIP in single system
Adsorbate |
Qexp/(mmol g−1) |
Langmuir isotherm |
Freundlich isotherm |
Qmax/(mmol g−1) |
KL/(L mmol−1) |
R2 |
KF |
n |
R2 |
Cd2+ |
0.521 |
0.560 |
5.911 |
0.981 |
0.444 |
3.836 |
0.942 |
Zn2+ |
0.126 |
0.215 |
14.899 |
0.998 |
0.202 |
3.724 |
0.785 |
Cu2+ |
0.137 |
0.238 |
11.941 |
0.999 |
0.218 |
3.494 |
0.847 |
Ni2+ |
0.101 |
0.199 |
12.791 |
0.997 |
0.188 |
3.258 |
0.739 |
Adsorption of heavy metals from binary-metal solutions. The impact of pH on the adsorption in binary-metal solutions is shown in Fig. 8(a–c). Being negligible at pH 2.0, the difference in adsorption capacities of Cd2+-IIP towards Cd2+ and the competing ions showed a substantial increase at higher pH. Compared with the single-metal solutions, the adsorption capacity in binary solutions decreased in respect to all metals, having, however, the adsorption capacity towards Cd2+ remained the highest as for the pre-designed imprinted polymer to fit the adsorption sites to the target ions.37 Based on the prerequisite, the other heavy metals presumably compete for the nonspecific hydroxyl group sites of Cd2+-IIP in ion exchange and the electrostatic interaction. The order of the Cd2+-IIP affinity towards the metal ions in binary-metal solutions follows the one observed in the single-metal ones. The selectivity coefficients of Cd2+-IIP for Cd2+ in the binary solutions are given in Table 2 at pH 5.0. It can be seen that the selectivity in the ionic pairs followed the descending order Ni2+ > Zn2+ > Cu2+. It should be noticed that although Cu2+, Ni2+ and Zn2+ ions have their charge and size close to those of Cd2+ at high affinity to the sulfhydryl ligand used in the Cd2+-IIP adsorbent, the latter still exhibits high selectivity towards Cd2+ for the specific recognition cavities designed in the template synthesis.
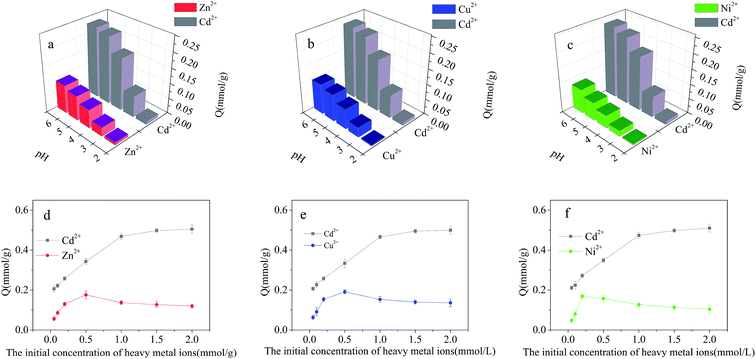 |
| Fig. 8 Effect of pH on the adsorption of Cd2+, Cu2+, Ni2+ and Zn2+ on Cd2+-IIP in binary-metal solutions: (a) Cd2+/Zn2+, (b) Cd2+/Cu2+, and (c) Cd2+/Ni2+(C0 = 10 mg L−1, temperature = 25 °C, t = 4 h, the dosage of adsorbent = 0.02 g); dependence of Cd2+, Cu2+, Ni2+ and Zn2+ adsorption on the Cd2+-IIP adsorbent in binary-metal solutions on the metals initial aqueous concentrations: (d) Cd2+/Zn2+, (e) Cd2+/Cu2+, (f) Cd2+/Ni2+ (pH = 5, C0 = 10–200 mg L−1, the dosage of adsorbent = 0.02 g, temperature = 25 °C, time = 4 h). | |
Table 2 The selectivity parameters of Cd2+-IIP for Cd2+ in binary system
Metals |
Kd(Cd2+) |
Kd(X2+) |
k |
Cd2+/Cu2+ |
4012 |
1073 |
3.74 |
Cd2+/Ni2+ |
4025 |
702 |
5.73 |
Cd2+/Zn2+ |
3983 |
959 |
4.15 |
The dependence of metallic ions adsorption at the Cd2+-IIP surface on their initial aqueous concentrations in binary-metal solutions (Cd2+/Cu2+, Cd2+/Ni2+, Cd2+/Zn2+) is given in Fig. 8(d, e and f), respectively. Similar to the single-metal solution, the adsorption of Cd2+ increased with increasing initial concentration of Cd2+. Unlike Cd2+, the adsorption Cu2+, Ni2+ and Zn2+ reached a maximum with further decrease and stabilization at a lower level with increasing aqueous concentration. At a relatively low concentration of contaminants, there was no competition between the metals, all the pollutants completely adsorbed on the surface of Cd2+-IIP, showing the adsorption equal to the one observed with the single-metal solutions. However, with the increasing initial concentration of metallic ions the competition was observed: Cu2+, Ni2+ and Zn2+ occupying the adsorption sites were substituted by Cd2+, making the surface concentrations of the ions competing Cd2+ decreased. This observation confirms the high selectivity of Cd2+-IIP towards Cd2+ originated from the template synthesis designing the specific adsorption sites for Cd2+ ions.
Adsorption of heavy metals from ternary- and quaternary-metal solutions. The effect of pH on the adsorption results from the ternary- (Cd2+/Cu2+/Ni2+, Cd2+/Cu2+/Zn2+ and Cd2+/Zn2+/Ni2+) and quaternary-metal (Cd2+/Cu2+/Ni2+/Zn2+) solutions are given in Fig. 9(a–d). Compared with the results obtained with the binary-metal solutions, the adsorption capacity of Cd2+-IIP adsorbent in respect to the heavy metal ions decreased to a various extent in the ternary- and quaternary-metal solutions. The affinity order also somewhat changed: the adsorption capacity descent observed in the row Cd2+ > Zn2+ > Cu2+ > Ni2+ being different from the observed for the binary-metal solutions.
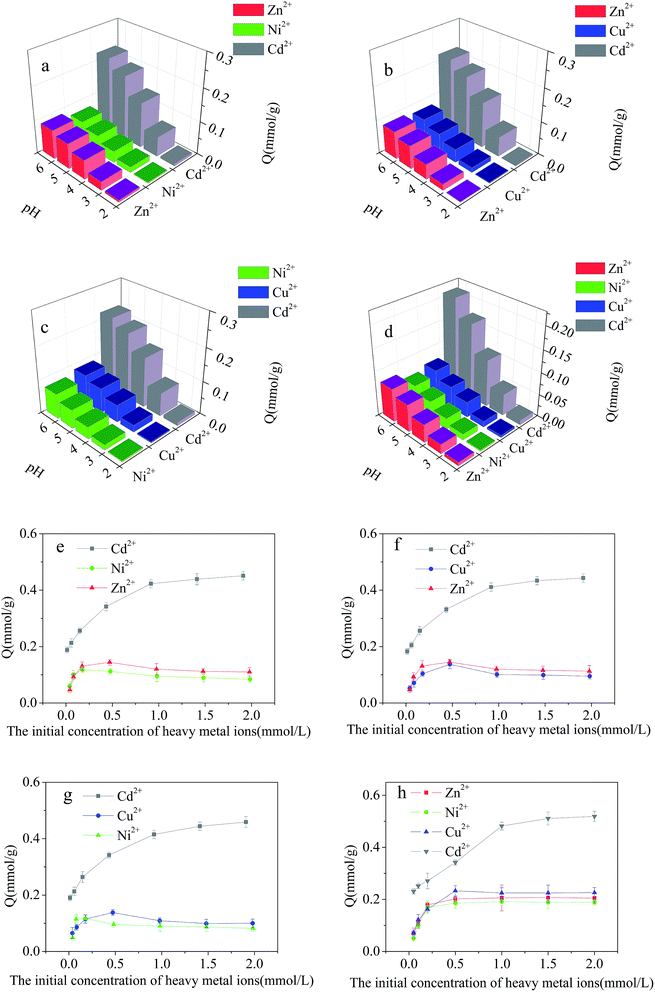 |
| Fig. 9 Effect of pH on the adsorption of Cd2+, Cu2+, Ni2+ and Zn2+ on Cd2+-IIP in ternary- and quaternary-metal solutions: (a) Cd2+/Zn2+/Ni2+, (b) Cd2+/Zn2+/Cu2+, (c) Cd2+/Cu2+/Ni2+ and (d) Cd2+/Zn2+/Cu2+/Ni2+(C0 = 10 mg L−1, temperature = 25 °C, t = 4 h, the dosage of adsorbent = 0.02 g); dependence of Cd2+, Cu2+, Ni2+ and Zn2+ adsorption on the Cd2+-IIP adsorbent in ternary- and quaternary-metal solutions on the metals initial aqueous concentrations: (e) Cd2+/Zn2+/Ni2+, (f) Cd2+/Zn2+/Cu2+, (g) Cd2+/Cu2+/Ni2+ and (h) Cd2+/Zn2+/Cu2+/Ni2+(pH = 5, C0 = 10–200 mg L−1, the dosage of adsorbent = 0.02 g, temperature = 25 °C, time = 4 h). | |
The effect of initial concentration of heavy metal ions on the results of the competitive adsorption from ternary- and quaternary-metal solutions onto Cd2+-IIP adsorbent are given in Fig. 9(e–h). The amount of Cd2+ adsorbed by Cd2+-IIP in multi-metal solutions exceeded the ones of other heavy metal ions at equal starting concentration. As for the other heavy metals, the adsorption isotherms of these lined up in adsorbed quantities in the descending order of Zn2+ > Cu2+ > Ni2+ consistent with cationic radii,36 respectively. The ion radius is thus apparently playing an important role in the selective adsorption performance of ion-imprinted Cd2+-IIP adsorbent, making the observation potentially useful in designing of adsorbent materials with the specific properties.
Effect of organic acids on adsorption of Cd2+
Adsorption of organic acids on Cd2+-IIP. When the concentration of low molecule weight organic acids was in the range of 0–100 mg L−1, the adsorption performance of citric, tartaric and oxalic acids on the Cd2+-IIP adsorbent from single-acid solutions in pH = 7 is illustrated in Fig. 10(a). The affinity of acids to Cd2+-IIP followed the descending order oxalic > tartaric > citric acid, consistent with their molecular mass growth: the smaller the molecular weight of the acid the more of it adsorbs on the Cd2+-IIP surface by hydrogen bond, van der Waals' force and/or electrostatic interaction. Hydrogen bond is one of the most important interaction force in the adsorption process of silica gel which has hydroxyl groups and silanol groups.38 Cd2+-IIP contain –OH and –SH group, while the organic acid contain –COOH and –OH group, so hydrogen bond may exist in the adsorption process of Cd2+-IIP towards organic acids. Electrostatic interaction also plays an important role in the acids adsorption by Cd2+-IIP. Generally, the adsorbed amounts of the acids were relatively low which might be due to the negative charge of dissociated acid anions39 repelled by thiol-functionalized group so it might have a relatively strong repulsion forces with Cd2+-IIP. According to previous report, oxalate, tartrate and citrate ions can form surface chelates with 5- or 6-memberated ring structures, which are far more stable than mono-dentate complexes.39 So oxalate, tartrate and citrate ions may chelate the surface of Cd2+-IIP with 5- or 6-memberated ring structures. The chelation may also be therefore responsible for the acids adsorption.
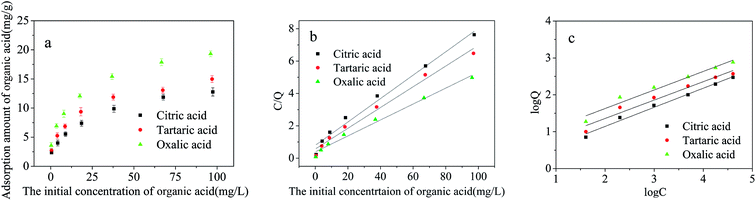 |
| Fig. 10 (a) Dependence of citric, tartaric and oxalic acid adsorption on the Cd2+-IIP adsorbent on their initial aqueous concentrations (pH = 7, C0 = 1–100 mg L−1, the dosage of adsorbent = 0.02 g, temperature = 25 °C, t = 4 h); the equilibrium isotherms: (b) the Langmuir model (c) the Freundlich model. | |
The parameters of Langmuir and Freundlich isotherms of small molecular organic acids are given in Table 3 and Fig. 10(b, c). Since the correlation coefficients (R2) of Freundlich model exceeding 0.99 surpass those of the Langmuir one, the adsorption seems to follow the multilayer pattern40 with consistently increasing surface acid concentration with the increased aqueous concentration of adsorbate.
Table 3 Parameters of Langmuir and Freundlich isotherms for adsorption of citric acid, tartaric acid and oxalic acid onto Cd2+-IIP
Adsorbate |
Langmuir isotherm |
Freundlich isotherm |
Qmax/(mg g−1) |
KL/(L mg−1) |
R2 |
KF |
n |
R2 |
Citric acid |
14.104 |
0.076 |
0.984 |
2.296 |
2.612 |
0.996 |
Tartaric acid |
15.949 |
0.090 |
0.988 |
2.858 |
2.693 |
0.992 |
Oxalic acid |
20.921 |
0.091 |
0.989 |
3.749 |
2.691 |
0.994 |
Effect of organic acids on Cd2+ adsorption isotherms. The effect of citric, tartaric and oxalic acid admixtures in binary solutions on the adsorption capacity of Cd2+ is shown in Fig. 11(a). The initial concentration of acids was kept at 50 mg L−1 and the initial concentration of Cd2+ ranged from 0 to 200 mg L−1. Compared with the adsorption of Cd2+ from the single-metal solution, the adsorption capacity of Cd2+-IIP adsorbent towards Cd2+ in presence of citric, tartaric and oxalic acids decreased for 32.9%, 43.9% and 64.7%, respectively. Strong impact of acids is seen in the ability of these to form stable complexes with metallic ions obstructing adsorption of free metal cations at the adsorbent surface. The difference between acids is explained due to the molecular size, the number of carboxyl moieties and electric charge characteristics of the organic acids, determining stability of their complexes with Cd2+. From the parameters of isotherm models (Table 4 and Fig. 11(b, c)). It can be seen that the Langmuir model fitting to the experimental Cd2+ adsorption data characterizing the surface of the adsorbent as uniform and energetically homogeneous41 providing monolayer adsorption of Cd2+. The adsorption capacities of Cd2+-IIP towards Cd2+ in presence of the acids of the Langmuir model were also close to the experimentally obtained values.
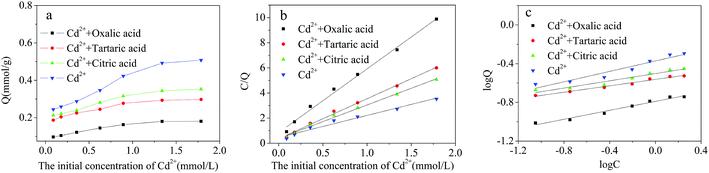 |
| Fig. 11 (a) Dependence of Cd2+ adsorption on the Cd2+-IIP adsorbent on its initial aqueous concentration in presence of citric, tartaric and oxalic acids in single-acid solutions (pH = 7, C0 = 10–100 mg L−1, the dosage of adsorbent = 0.02 g, temperature = 25 °C, t = 4 h); the equilibrium isotherms: (b) the Langmuir model (c) the Freundlich model. | |
Table 4 Parameters of Langmuir and Freundlich isotherms for adsorption of Cd2+ onto Cd2+-IIP in the presence of citric acid, tartaric acid and oxalic acid
Coexisting organic acids |
Langmuir isotherm |
Freundlich isotherm |
Qexp/(mmol g−1) |
Qmax/(mmol g−1) |
KL/(L mmol−1) |
R2 |
KF |
n |
R2 |
Control group (Cd2+) |
0.508 |
0.570 |
3.837 |
0.976 |
0.139 |
3.719 |
0.936 |
Citric acid |
0.353 |
0.377 |
6.780 |
0.993 |
0.070 |
5.336 |
0.949 |
Tartaric acid |
0.297 |
0.314 |
8.953 |
0.997 |
0.051 |
6.045 |
0.982 |
Oxalic acid |
0.181 |
0.197 |
5.989 |
0.994 |
0.013 |
4.325 |
0.977 |
Effect of the initial concentration of organic acids on the adsorption of Cd2+. The adsorption of Cd2+ decreasing in presence of organic acids (Fig. 11) requires closer insight to characterize the impact of acid admixtures to the adsorbent performance. Fig. 12 presents the dependence of Cd2+ adsorption from the solutions containing 10 mg L−1 of the metal cation on the content of organic acids (10–100 mg L−1). The adsorption of Cd2+ increased with the increasing initial concentration of citric and tartaric acids in single-acid solutions from 0 to 10 mg L−1, and then decreased with the further increasing acid concentration. The adsorption of Cd2+ was, however, only reduced in the presence of oxalic acid, the increase in adsorption was not observed. The inhibitory effect of acids strengthened with the increased acid concentration in respect of all acids. Moreover, from the achieved results, it is possible to affirm that IIP is prone to interference by matter organic and can not be used for Cd2+ removal from real samples when the initial concentration of organic acid was higher than 10 mg L−1.
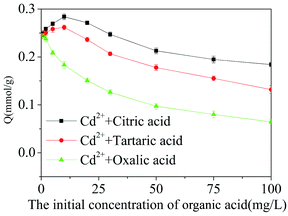 |
| Fig. 12 Effect of initial concentrations of organic acids on the adsorption of Cd2+ on Cd2+-IIP adsorbent (pH = 7, C0(Cd2+) = 10 mg L−1, the dosage of adsorbent = 0.02 g, temperature = 25 °C, t = 4 h). | |
The adsorption ability of Cd2+-IIP mainly depends on the active adsorption sites, electric charge of adsorbent surface and the properties of adsorbate. Organic acids contain carboxyl groups, which have the ability to coordinate with Cd2+-ions exhibiting their cumulative complexing stability constants in the descending order tartaric > citric > oxalic acid.42,43 The role of citric acid and tartaric acid in promoting the adsorption of Cd2+ may be due to (i) formation of water-soluble Cd2+-complexes of organic acids having the electric charge contrary to the one of adsorbent, and, probably, forming a dipole with its charge polarization,44 and/or (ii) formation of outer sphere Cd2+–acid complexes at the Cd2+-IIP adsorption sites: the multi-layer adsorption of acids described by the Freundlich model indirectly supports this hypothesis (Fig. 10 and Table 4). The Cd2+ adsorption inhibited with acids may be explained by the discussed above formation of water-soluble Cd2+–acid complexes.41
Regeneration
The reuse ability of one adsorbent is a very important parameter for its in practical application.45 Therefore, the regeneration performance of Cd2+-IIP with thiol-functional groups was investigated. 0.02 g Cd2+ ion-imprinted adsorbent was immersed into 100 mL Cd2+ solution with the concentration of 0.1 mmol L−1 and stirred for 4 h at 25 °C. After adsorption, the used Cd2+-IIP was dried by filtration and isolation and then added to the conical flask with 1 mol L−1 HCl solution. Fig. 13 gave some details for regeneration experiment results. After reused for 5 times, Cd2+-IIP still showed good regeneration rate which was greater than 85%, indicating that Cd2+-IIP was a potential adsorbent for practical application.
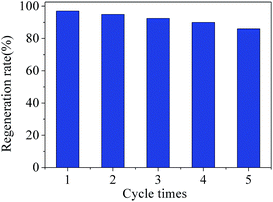 |
| Fig. 13 Recycling of Cd2+-IIP in the removal of Cd2+ from aqueous solutions (C0(Cd2+) = 0.1 mmol L−1, the dosage of adsorbent = 0.02 g, temperature = 25 °C, t = 4 h). | |
Conclusions
The Cd2+ ion-imprinted adsorbent Cd2+-IIP with thiol-functional groups was successfully synthesized applying the surface ion imprinting technique combined with ultrasonic heating and hydrothermal method using silica gel as substrate. The Cd2+-IIP adsorbed the target Cd2+-ion from aqueous solutions with high degree of selectivity. The latter was achieved on account of Cd2+ interaction with specific proper-size recognition cavities in Cd2+-IIP adsorbent originated from the synthetic procedure: the template synthesis left Cd2+-ion proper size voids in the carrier material. The selectivity was demonstrated in respect to mixtures with Zn2+, Cu2+ and Ni2+ ions of the size and charge close to the target Cd2+. The description of metal ions adsorption at the Cd2+-IIP adsorbent is satisfactorily described by the Langmuir model. The presence of tartaric, citric and oxalic acids as admixtures in Cd2+ aqueous solutions noticeably reduced the cation adsorption in wide range of concentrations with the minor exception of low contents of citric and tartaric acids slightly improving adsorption. The impairment of adsorption may be explained by formation of poorly adsorbable water-soluble complexes switching off the template-designed Cd2+-size cavities from adsorption. Preliminary decomposing of metal–acid complex compounds with, e.g. oxidation, liberating free metallic cations prior to adsorption may appear necessary.
Conflicts of interest
There are no conflicts to declare.
Acknowledgements
The authors gratefully acknowledge the financial support provided by the Research and Development Foundation of Applied Science and Technology of Guangdong Province, China (No. 2015B020235005), the National Natural Science Foundation of China (No. 51278199) and Joint Key Funds of the National Natural Science Foundation of Guangdong Province, China (No. U1201234).
References
- S. Cen, W. Li, S. Xu, Z. Wang, Y. Tang, H. Wang and C. Wei, RSC Adv., 2017, 7, 7996–8003 RSC.
- J. Wang and F. Liu, Chem. Eng. J., 2014, 242, 117–126 CrossRef CAS.
- H. Ge and J. Wang, Chemosphere, 2017, 169, 443–449 CrossRef CAS PubMed.
- H. K. Boparai, M. Joseph and D. M. O'Carroll, J. Hazard. Mater., 2011, 186, 458–465 CrossRef CAS PubMed.
- E. M. Traudt, J. F. Ranville and J. S. Meyer, Environ. Sci. Technol., 2017, 51, 4471–4481 CrossRef CAS PubMed.
- S. Mallakpour, A. Abdolmaleki and H. Tabebordbar, Polym. Bull., 2017, 74, 2957–2973 CrossRef CAS.
- P. Chakravarty, N. Sen Sarma and H. P. Sarma, Chem. Eng. J., 2010, 162, 949–955 CrossRef CAS.
- G. Zeng, Y. Liu, L. Tang, G. Yang, Y. Pang, Y. Zhang, Y. Zhou, Z. Li, M. Li, M. Lai, X. He and Y. He, Chem. Eng. J., 2015, 259, 153–160 CrossRef CAS.
- M. Xu, P. Hadi, G. Chen and G. McKay, J. Hazard. Mater., 2014, 273, 118–123 CrossRef CAS PubMed.
- J. E. D. V. Segundo, G. R. Salazar-Banda, A. C. O. Feitoza, E. O. Vilar and E. B. Cavalcanti, Sep. Purif. Technol., 2012, 88, 107–115 CrossRef CAS.
- J. Gao, S. Sun, W. Zhu and T. Chung, J. Membr. Sci., 2016, 499, 361–369 CrossRef CAS.
- W. M. Wang and V. Fthenakis, J. Hazard. Mater., 2005, 125, 80–88 CrossRef CAS PubMed.
- S. Mauchauffee, E. Meux and M. Schneider, Sep. Purif. Technol., 2008, 62, 394–400 CrossRef CAS.
- H. Zhu, J. Pan, J. Cao, Y. Ma, F. Qiu, W. Zhang and Y. Yan, J. Ind. Eng. Chem., 2017, 49, 198–207 CrossRef CAS.
- T. P. Rao, S. Daniel and J. M. Gladis, TrAC, Trends Anal. Chem., 2004, 23, 28–35 CrossRef.
- B. Gao, J. Meng, Y. Xu and Y. Zhang, J. Ind. Eng. Chem., 2015, 24, 351–358 CrossRef CAS.
- Z. Li, H. Fan, Y. Zhang, M. Chen, Z. Yu, X. Cao and T. Sun, Chem. Eng. J., 2011, 171, 703–710 CrossRef CAS.
- H. Fan, X. Sun, Z. Zhang and W. Li, J. Chem. Eng. Data, 2014, 59, 2106–2114 CrossRef CAS.
- N. Khoddami and F. Shemirani, Talanta, 2016, 146, 244–252 CrossRef CAS PubMed.
- Z. Li, H. Fan, Y. Zhang, M. Chen, Z. Yu, X. Cao and T. Sun, Chem. Eng. J., 2011, 171, 703–710 CrossRef CAS.
- D. K. Singh and S. Mishra, J. Hazard. Mater., 2009, 164, 1547–1551 CrossRef CAS PubMed.
- N. Zhao, C. Zhao, Y. Lv, W. Zhang, Y. Du, Z. Hao and J. Zhang, Chemosphere, 2017, 186, 422–429 CrossRef CAS PubMed.
- C. Wang, Z. Wang, L. Lin, B. Tian and Y. Pei, J. Hazard. Mater., 2012, 203, 145–150 CrossRef PubMed.
- Z. Wang, Y. Gao, S. Wang, H. Fang, D. Xu and F. Zhang, Environ. Sci. Pollut. Res., 2016, 23, 10938–10945 CrossRef CAS PubMed.
- X. Hu, Y. Liu, H. Wang, G. Zeng, X. Hu, Y. Guo, T. Li, A. Chen, L. Jiang and F. Guo, Chem. Eng. Res. Des., 2015, 93, 675–683 CrossRef CAS.
- L. Song, X. Zhao, J. Fu, X. Wang, Y. Sheng and X. Liu, J. Hazard. Mater., 2012, 199, 433–439 CrossRef PubMed.
- Y. Wang, J. Chen, Y. Cui, S. Wang and D. Zhou, J. Hazard. Mater., 2009, 162, 1135–1140 CrossRef CAS PubMed.
- L. Huang, H. Hu, X. Li and L. Y. Li, Appl. Clay Sci., 2010, 49, 281–287 CrossRef CAS.
- I. Langmuir, J. Am. Chem. Soc., 1918, 1361–1403 CrossRef CAS.
- H. M. F. Freundlich, J. Phys. Chem., 1906, 1100–1107 Search PubMed.
- A. M. Donia, A. A. Atia, A. M. Daher, O. A. Desouky and E. A. Elshehy, J. Radioanal. Nucl. Chem., 2011, 290, 297–306 CrossRef CAS.
- S. M. Evangelista, E. DeOliveira, G. R. Castro, L. F. Zara and A. G. S. Prado, Surf. Sci., 2007, 601, 2194–2202 CrossRef CAS.
- H. Fan, J. Li, Z. Li and T. Sun, Appl. Surf. Sci., 2012, 258, 3815–3822 CrossRef CAS.
- A. Aghababaei, M. C. Ncibi and M. Sillanpaa, Bioresour. Technol., 2017, 239, 28–36 CrossRef CAS PubMed.
- M. O. Ojemaye, O. O. Okoh and A. I. Okoh, Sep. Purif. Technol., 2017, 183, 204–215 CrossRef CAS.
- Z. Gao, T. J. Bandosz, Z. Zhao, M. Han and J. Qiu, J. Hazard. Mater., 2009, 167, 357–365 CrossRef CAS PubMed.
- M. N. Ahmad, M. Y. M. Sim, C. C. Cheen, A. K. M. S. Islam, Z. Ismail, M. Surif, A. Y. M. Shakaff and L. Lvova, Microchim. Acta, 2008, 163, 113–119 CrossRef CAS.
- A. A. Christy, Vib. Spectrosc., 2010, 54, 42–49 CrossRef CAS.
- L. Huang, H. Hu, X. Li and L. Y. Li, Appl. Clay Sci., 2010, 49, 281–287 CrossRef CAS.
- Z. Yu, Q. Dang, C. Liu, D. Cha, H. Zhang, W. Zhu, Q. Zhang and B. Fan, Carbohydr. Polym., 2017, 172, 28–39 CrossRef CAS PubMed.
- M. Malandrino, O. Abollino, A. Giacomino, M. Aceto and E. Mentasti, J. Colloid Interface Sci., 2006, 299, 537–546 CrossRef CAS PubMed.
- O. Abollino, M. Aceto, M. Malandrino, C. Sarzanini and E. Mentasti, Water Res., 2003, 37, 1619–1627 CrossRef CAS PubMed.
- H. Zhu, X. Cao, Y. He, Q. Kong, H. He and J. Wang, Carbohydr. Polym., 2015, 129, 115–126 CrossRef CAS PubMed.
- L. Huang, H. Hu, X. Li and L. Y. Li, Appl. Clay Sci., 2010, 49, 281–287 CrossRef CAS.
- G. Yuan, H. Tu, J. Liu, C. Zhao, J. Liao, Y. Yang, J. Yang and N. Liu, Chem. Eng. J., 2018, 333, 280–288 CrossRef CAS.
|
This journal is © The Royal Society of Chemistry 2018 |
Click here to see how this site uses Cookies. View our privacy policy here.