DOI:
10.1039/C7RA12579H
(Paper)
RSC Adv., 2018,
8, 2824-2828
Facile continuous process for gas phase halogen exchange over supported alkyl phosphonium salts
Received
19th November 2017
, Accepted 5th January 2018
First published on 12th January 2018
Abstract
Chloride–bromide halogen exchange was realized when a mixture of an alkyl chloride and an alkyl bromide were reacted over a supported molten alkyl phosphonium catalyst. Conversion was found to be near equilibrium in a tubular flow reactor at 150 °C and 1500 GHSV. The catalyst was prepared by impregnation of alumina or silica support and found to be highly stable for relatively long periods of time. A pathway for the catalytic cycle is proposed.
Introduction
The halogen exchange of alkyl and aryl halides is a fundamental reaction in pharmaceutical and agrochemical industries.1–4 The alkyl and aryl halide functional groups can readily be used in a wide range of cross-coupling reactions,5 organometallic reagents,6 physical and biological properties modification of aromatic rings7,8 and generation of free-radical intermediates precursor.1,9 Gas phase halogen exchange is mainly used for preparing alkyl bromides or iodides, which are more reactive and can be readily employed in numerous transformations.9–12 For halogen exchange reaction, Finkelstein reactions13 have been reported specifically along with alkyl lithium,14 Grignard reagents,15 and copper-catalyzed alkylation for the conversion of alkyl chlorides to the corresponding bromides or iodides.16–18 However a large excess of the metal halide makes the Finkelstein reaction procedure uneconomical at industrial level.19,20 Few groups reported the use of ionic liquids for the halogen exchange reactions.21–23 Others exhibited phase transfer catalysts (PTC) to accelerate halogen exchange between metal salts and alkyl halides in organic phase. Nonetheless, these reactions still suffer from the disadvantage of reversibility, with the demand of large excess donor halide salt for obtaining significant yields of the alkyl halide product.24–27
In the same context; halogen exchange reactions between alkyl bromides and alkyl chlorides were homogeneously catalyzed by tertiary amines at 110 °C.28,29 Yonovich and Sasson studied bromide–chloride exchange between alkyl halide and metal halide salts under phase transfer conditions.30 These authors have observed that calcium bromide can be effectively used as brominating agent for primary alkyl halides in the presence of lipophilic quaternary ammonium salts as phase transfer catalysts. Sasson et al. stated that role of metal cation in solid liquid phase transfer catalysis conditions was much more critical than that of the anions which were generally considered the main effect in phase transfer reactions. Further in an economic approach Sasson and his coworkers effectively demonstrated the use of phase transfer catalyst in halogen exchange reaction at industrial level and laboratory-scale to convert inorganic bromide salts from the respective chloride salts by reaction with 1,2 dibromoethane.31 The described simple technique avoids the corrosive and exothermic conditions of the conventional processes.32
In continuations of the same study in the present work; we tried to establish a protocol for halogen exchange reaction as a convenient way to prepare a desired alkyl halide from corresponding alkyl halides using readily available and cheap alkyl phosphonium salts on supported solid phase (alumina or silica) for continuous gas phase process.
In the present study halogen exchange reaction between alkyl chloride and alkyl bromide substrates in the gas phase is reported by using supported molten quaternary phosphonium salts as catalysts at temperature between 140–160 °C and at atmospheric pressure. Quaternary phosphonium salts are known to be more resilient to high temperature in comparison with the corresponding ammonium salts. 1,2-Dibromo (EDB) was used as the brominating agent, since it is an available and cheap source of bromine.
Experimental
Chemicals used in the experiments: alkyl phosphonium bromide, dichloromethane (Merck), alumina (Strem chemicals), silica (Alfa products), tetrabutyl phosphonium bromide (Aldrich), hexadecyl tributyl phosphonium bromide (Fluka). Ethylene dibromide and alkyl chlorides (Aldrich) were applied as received without further purifications.
Catalyst preparation
The catalyst was prepared by impregnation method. A solution containing the appropriate amount of the alkyl phosphonium bromide in a suitable amount of dichloromethane (Merk) was prepared. The alkyl phosphonium solution was added to the alumina (Strem chemicals) or silica (Alfa products) used as the supports. The mixture was evaporated to dryness at 50 °C in a flash evaporator and dried in air at 120 °C. Catalysts containing between 2% and 20% by weight of phosphonium salt were prepared by this method. The tetrabutyl phosphonium bromide (Aldrich) and the hexadecyl tributyl phosphonium bromide (Fluka) were used as obtained from suppliers.
Procedure
The experimental set up is described in Fig. 1. In a typical run a mixture of an alkyl chloride and 1,2 dibromoethane (EDB) were introduced into the reactor by a peristaltic pump. The alkyl halides were gasified over glass beads before reaching the catalyst. The resulting mixture was collected in a cold trap at the outlet of the reactor and analyzed by GLC. Typical experiments were carried out at temperature between 140–160 °C, atmospheric pressure and GHSV between 1500–2000. The EDB (Riedel) used as the brominating agent and the alkyl chlorides (Aldrich or Fluka) were used as received.
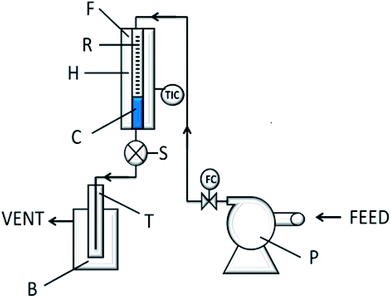 |
| Fig. 1 Flow diagram of the reaction system. F = furnace, R = pyrex reactor, H = preheater, C = catalyst bead, S = sampling device, T = trap, B = cold batch, P = peristaltic pump. | |
Results and discussion
The halogen exchange reaction between alkyl halides is an equilibrium reaction. In the present study, when a mixture of alkyl chloride and EDB were reacted in the gas phase in the presence of an alkyl phosphonium salt catalyst supported on alumina or silica, a mixture of alkyl halides was obtained. Molar conversions obtained per path were near to equilibrium conversions and were typically between 50–80%, depending on the nature of the alkyl halides.
Fig. 2 shows the behavior of the reaction of ethylene dichloride with ethylene dibromide at 150° over three different catalysts. 10% w/w tetrabutylphosphonium bromide on alumina, 10% w/w hexadecyltributylphosphonium bromide on alumina and 10% w/w of tetrabutylphosphonium bromide on silica. Al2O3 supported catalysts (∼85%) shows good conversion rather than the SiO2 (∼55%) supported catalysts shown in Fig. 2. However Al2O3 or SiO2 heterogeneous support alone do not show any conversion but with combination of tetrabutyl phosphonium or hexadecyl tributyl phosphonium with Al2O3 enhanced halogen exchange reaction multiple folds (upto 80% product conversion). Probably due to Al2O3 acidic group interaction with phosphonium bromide catalyst.33,34 We may conclude that the equilibrium conversion under these conditions is above 80%.
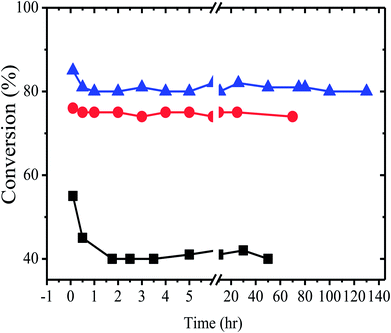 |
| Fig. 2 Activity of the alkyl phosphonium catalysts as a function of time at 150 °C, (a) (▲) = 10% tetrabutyl phosphonium/Al2O3, (b) (●) = 10% hexadecyl tributyl phosphonium/Al2O3, (c) (■) = 10% tetrabutyl phosphonium/SiO2. At 150 °C, 1 atm and 1500 GHSV. 1 : 1 molar mixture of reactants (EDB + EDC). | |
Typical results obtained over 10% (weight) tetrabutyl phosphonium bromide supported on the alumina catalyst are presented in Table 1, when molar mixture of 1
:
1 of the reactants were used. The conversion is easily increased by using an excess of EDB.
Table 1 Bromide chloride exchange in the gas phase over 10% (C4H9)4PBr/Al2O3 catalyst at 150 °C, 1 atm and 1500 GHSV. 1
:
1 molar mixture of reactants
RCl + R′Br → R′Cl + RBr |
Entry |
Reaction |
Conversion per path (% mol) |
1. |
C6H5CH2Cl + (CH2)2Br2 → C6H5CH2Br + Br(CH2)2Cl + (CH2)2Cl2 |
70 |
2. |
CH3(CH2)5Cl + (CH2)2Br2 → CH3(CH2)5Br + Br(CH2)2Cl + (CH2)2Cl2 |
67 |
3. |
CH3(CH2)4Cl + (CH2)2Br2 → CH3(CH2)4Br + Br(CH2)2Cl + (CH2)2Cl2 |
62 |
4. |
CH3(CH2)3Cl + (CH2)2Br2 → CH3(CH2)3Br + Br(CH2)2Cl + (CH2)2Cl2 |
51 |
5. |
CH2Cl2 + (CH2)2Br2 → CH2CIBr + Br(CH2)2Cl + CH2Br2 + (CH2)2Cl2 |
∼5 |
6. |
BrCH2CH2Br + Cl(CH2)2Cl → 2BrCH2CH2Cl |
80 |
The catalysts were found to be highly stable, giving almost the same rate of reaction over relatively long periods of time. These results are clearly illustrated in Fig. 2, which describes the activity of the catalysts over a period of more than 100 h. As expected from the theory of SLPC29 there is an optimal value of the liquid loading on the catalyst. Table 2 and Fig. 3 presents the summarized results of a series of experiments in which the liquid loading was varied. From these results the effect of the liquid load is clearly observed. For example, it can be realized that for the TBP/Al2O3 catalyst the optimal liquid load is in between 5–10% (weight).
Table 2 Influence of the liquid loading on the activity of catalyst (load given in % weight of catalyst)
Entry |
Catalyst |
Specific activity (% mol conversion/gm catalyst) |
C16H33[CH3(CH2)3]3PBr |
1. |
10%/Al2O3 |
7.8 |
2. |
15%/Al2O3 |
6.7 |
3. |
20%/Al2O3 |
5.1 |
![[thin space (1/6-em)]](https://www.rsc.org/images/entities/char_2009.gif) |
[CH3(CH2)3]4PBr |
1. |
2.5%/Al2O3 |
4.8 |
2. |
5%/Al2O3 |
8.8 |
3. |
10%/Al2O3 |
8.4 |
4. |
15%/Al2O3 |
6.3 |
5. |
20%/Al2O3 |
5.4 |
6. |
5%/SiO2 |
5.2 |
7. |
10%/SiO2 |
4.3 |
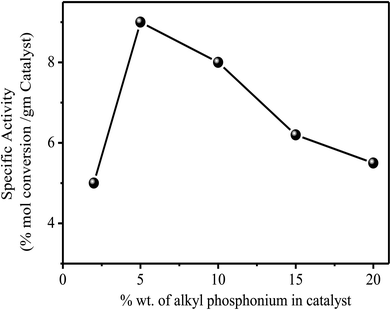 |
| Fig. 3 Influence of tetrabutyl phosphonium load on the activity of the catalyst in the reaction of EDB + EDC at 150 °C. At 150 °C, 1 atm and 1500 GHSV. 1 : 1 molar mixture of reactants. | |
Exchange reaction is
(CH2)2Cl2 + (CH2)2Br2 → 2(CH2)2BrCl |
1
![[thin space (1/6-em)]](https://www.rsc.org/images/entities/char_2009.gif)
:
![[thin space (1/6-em)]](https://www.rsc.org/images/entities/char_2009.gif)
1 molar mixture at 150 °C, 1 atm and 1500 GHSV.
Additional experiments are necessary to obtain accurate values of the liquid loading optimum. It is interesting to point out that neither the SiO2 nor the Al2O3 used as the supports were active in this reaction, when used unloaded. The alumina gives very small conversions for the exchange reaction (<5%) and mainly products of elimination reactions were detected. Therefore from Table 2 it is very clear that support (SiO2 or Al2O3) is not playing active role in halogen exchange reaction. A possible pathway for the exchange reaction over the alkyl phosphonium catalyst is summarized in the following scheme.
|
RCl + QBr → RBr + QCl
| (1) |
|
R′Br + QCl → R′Cl + QBr
| (2) |
The overall catalytic cycle is summarized by the reaction;
|
RCl + R′Br → RBr + R′Cl
| (3) |
The proposed catalytic sequence is supported by the results obtained in a series of experiments (Fig. 4) in which the alkyl phosphonium bromide catalyst was reacted first with the alkyl chloride converting the alkyl phosphonium bromide into the chloride and producing the respective alkyl bromide (reaction (1)). The alkyl phosphonium chloride was then reacted with the alkyl bromide according to reaction (2).
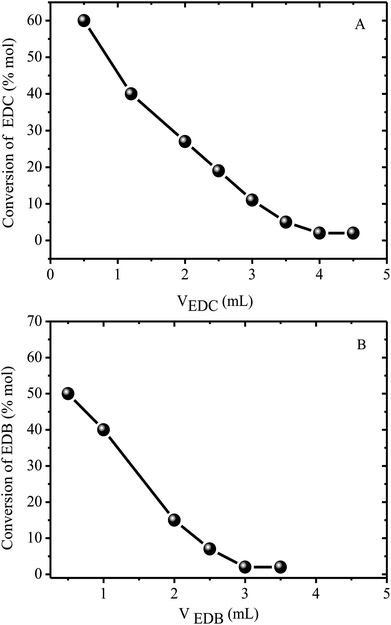 |
| Fig. 4 Reaction of the alkyl halides with the alkyl phosphonium catalyst at 150 °C. (A) = reaction of 1,2 dichloro ethane (EDC) with tetrabutyl phosphonium bromide (reaction (1)). (B) = reaction of 1,2 dibromoethane (EDB) with tetrabutyl phosphonium chloride (reaction (2)). | |
The reaction between 1,2 dichloro ethane (EDC) and 1,2 dibromoethane (EDB) was used to illustrate the proposed mechanism because of its relative simplicity giving only one possible reaction product according to the equation;
|
BrC2H4Br + ClC2H4Cl → 2BrC2H4Cl
| (4) |
Additionally evidence supporting the proposed catalytic cycle could be obtained from preliminary kinetic investigation carried out in this laboratory. From the results obtained, the reaction seems to follow a second order reversible kinetics, according to the equation (Fig. 5);
Xe = equilibrium conversion,
k1 = second order rate reaction,
V = reactor volume,
F = molar flow rate,
CA0 = concentration of A at the entrance of reactor,
X = conversion.
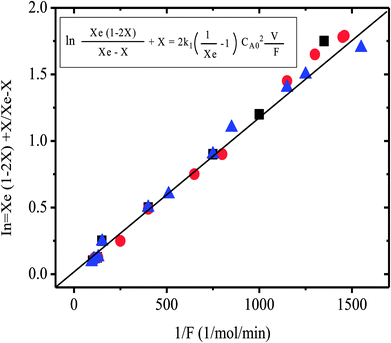 |
| Fig. 5 Plot of variables for the second order reversible kinetics for the halide exchange reaction (4), over 10% tetrabutyl phosphonium/Al2O3 at 148 °C. Symbols are for different experiments. | |
A plot of this kinetic equation using the experimental results is presented in Fig. 5 (second order reversible kinetics). The equilibrium conversion required was estimated by method described elsewhere.35 However a more detailed kinetic investigation should be made before conclusive evidence could be obtained. Such a kinetic investigation is under study in this laboratory in order to determine the accurate rate equation of this reaction.
Conclusions
Herein we demonstrated an efficient equilibrium conversion method for halogen exchange (chloride to bromide) using molten alkyl phosphonium catalyst without any additional material usage in multiple cycles in a long continuous flow. Alkyl phosphonium catalyst reactivity screened under various optimization parameters viz; loading (2–20%), supported over (silica or alumina) for halogen exchange reaction. The silica and alumina based phase-transfer catalyst easily regenerated and reused several times without loss of their activity. The products of the exchange reactions could be simply separated by distillation under normal pressure.
Conflicts of interest
There are no conflicts of interest in the present research work.
Acknowledgements
The authors warmly acknowledge Dr Leonardo Mendelovici for his help to accomplish the present research work.
References
- T. D. Sheppard, Org. Biomol. Chem., 2009, 7, 1043–1052 CAS.
- Y. Mizukami, Z. Song and T. Takahashi, Org. Lett., 2015, 17, 5942–5945 CrossRef CAS PubMed.
- J. San Filippo, A. F. Sowinski and L. J. Romano, J. Org. Chem., 1975, 40, 3295–3296 CrossRef CAS.
- M. Namavari, N. Satyamurthy, M. E. Phelps and J. R. Barrio, Tetrahedron Lett., 1990, 31, 4973–4976 CrossRef CAS.
- C.-L. Sun and Z.-J. Shi, Chem. Rev., 2014, 114, 9219–9280 CrossRef CAS PubMed.
- R. Bloch, Chem. Rev., 1998, 98, 1407–1438 CrossRef CAS PubMed.
- C. Fischer and B. Koenig, Beilstein J. Org. Chem., 2011, 7, 59–74 CrossRef CAS PubMed.
- T. Kihlberg and B. Långström, J. Org. Chem., 1999, 64, 9201–9205 CrossRef CAS.
- R. Viswanathan, E. N. Prabhakaran, M. A. Plotkin and J. N. Johnston, J. Am. Chem. Soc., 2003, 125, 163–168 CrossRef CAS PubMed.
- A. S. Guram, R. A. Rennels and S. L. Buchwald, Angew. Chem., Int. Ed. Engl., 1995, 34, 1348–1350 CrossRef CAS.
- K. W. Anderson, T. Ikawa, R. E. Tundel and S. L. Buchwald, J. Am. Chem. Soc., 2006, 128, 10694–10695 CrossRef CAS PubMed.
- Y. Liu, Y. Jiang, H. Yin, W. Chen, Y. Shen, Y. Feng, L. Shen, A. Wang, T. Jiang and Z. Wu, Korean J. Chem. Eng., 2011, 28, 2250–2254 CrossRef CAS.
- Z. Wang, in Comprehensive Organic Name Reactions and Reagents, John Wiley & Sons, Inc., 2010 Search PubMed.
- C. Strohmann, B. C. Abele, K. Lehmen and D. Schildbach, Angew. Chem., Int. Ed., 2005, 44, 3136–3139 CrossRef CAS PubMed.
- M. Ohno, K. Shimizu, K. Ishizaki, T. Sasaki and S. Eguchi, J. Org. Chem., 1988, 53, 729–733 CrossRef CAS.
- K. W. Shimkin, P. G. Gildner and D. A. Watson, Org. Lett., 2016, 18, 988–991 CrossRef CAS PubMed.
- P. Ren, I. Salihu, R. Scopelliti and X. Hu, Org. Lett., 2012, 14, 1748–1751 CrossRef CAS PubMed.
- H.-Q. Do, S. Bachman, A. C. Bissember, J. C. Peters and G. C. Fu, J. Am. Chem. Soc., 2014, 136, 2162–2167 CrossRef CAS PubMed.
- W. J. Bailey and E. Fujiwara, J. Am. Chem. Soc., 1955, 77, 165–166 CrossRef CAS.
- E. D. Hughes, C. K. Ingold and J. D. H. Mackie, J. Chem. Soc., 1955, 3173–3177 RSC.
- A. Wang, Y. Jiang, W. Chen, H. Yin, Y. Liu, Y. Shen, T. Jiang and Z. Wu, J. Ind. Eng. Chem., 2012, 18, 237–242 CrossRef CAS.
- R. L. Vekariya, J. Mol. Liq., 2017, 227, 44–60 CrossRef CAS.
- E. Alcalde, I. Dinarès, A. Ibáñez and N. Mesquida, Molecules, 2012, 17, 4007 CrossRef CAS PubMed.
- P. Tundo, P. Venturello and E. Angeletti, J. Chem. Soc., Perkin Trans. 2, 1983, 485–491 RSC.
- C. M. Starks, J. Am. Chem. Soc., 1971, 93, 195–199 CrossRef CAS.
- P. N. Leelamma and K. S. Devaky, J. Appl. Polym. Sci., 2009, 112, 2750–2756 CrossRef CAS.
- T. Ido, T. Yamamoto, G. Jin and S. Goto, Chem. Eng. Sci., 1997, 52, 3511–3520 CrossRef CAS.
- Y. Sasson and M. Yonovich-Weiss, J. Mol. Catal., 1981, 10, 357–360 CrossRef CAS.
- P. Tundo, J. Org. Chem., 1979, 44, 2048–2049 CrossRef CAS.
- M. Yonovich-Weiss and Y. Sasson, Synthesis, 1984, 1984, 34–35 CrossRef.
- R. Aizenberg, O. Arrad and Y. Sasson, Ind. Eng. Chem. Res., 1992, 31, 431–434 CrossRef CAS.
- E. M. Pearce, J. Polym. Sci., Polym. Lett. Ed., 1978, 16, 248 CrossRef.
- C. Chizallet and P. Raybaud, Angew. Chem., Int. Ed., 2009, 48, 2891–2893 CrossRef CAS PubMed.
- K. Motokura, M. Tada and Y. Iwasawa, J. Am. Chem. Soc., 2007, 129, 9540–9541 CrossRef CAS PubMed.
- D. R. Stull, E. F. Westrum and G. C. Sinke, The chemical thermodynamics of organic compounds, J. Wiley, New York, 1969 Search PubMed.
|
This journal is © The Royal Society of Chemistry 2018 |
Click here to see how this site uses Cookies. View our privacy policy here.