DOI:
10.1039/C7RA12779K
(Paper)
RSC Adv., 2018,
8, 5792-5796
An Au@Ag nanocube based plasmonic nano-sensor for rapid detection of sulfide ions with high sensitivity†
Received
25th November 2017
, Accepted 18th January 2018
First published on 2nd February 2018
Abstract
Based on the localized surface plasmon resonance (LSPR) technology, a novel plasmonic nanosensor with high sensitivity and high selectivity was prepared for the detection of trace sulfide ions on an individual Au@Ag nanoparticle. Furthermore, it could be used to monitor the sulfurization on an individual Au@Ag nanoparticle surface observed under dark-field microscopy (DFM).
Introduction
Hydrogen sulfide (H2S), known for its toxicity, is a colorless acidic gas with a “rotten egg” smell. Recently, it was found that hydrogen sulfide is the third gaseous mediator in mammals and has an impact on human physiology and pathology, including neurotransmission,1,2 vasorelaxation,3,4 inhibition of insulin signaling,5,6 and anti-inflammation.7 Many diseases are also associated with the abnormal concentrations of H2S, such as Alzheimer's disease,8 ischaemia,9 and Down's syndrome.10,11 As a new gasotransmitter similar to nitric oxide (NO) and carbon monoxide (CO), H2S is gradually attracting more and more attention of researchers for its understanding and exploration. According to the reported results, sulfide ions could be detected now by various methods, such as the color analysis,12–14 electrochemical analysis,15 and gas chromatography analysis.16 For example, some fluorescent H2S probes have been designed by utilizing several chemical characteristics of H2S, such as the reduction of azide groups,11,12,17 nucleophilic addition,18 the S–Cu bonding,19,20 and nucleophilic substitution.13,21 Although the limit of detection (LOD) of these methods usually only reaches μM range (which is suggested as the average endogenous H2S level in most publications), the sulfide ion concentrations in the cellular anabolic and catabolic reactions can be as low as nM to sub-mM.22 Therefore, it is important to develop a novel nanosensor to map the distributions of sulfide ions and to monitor the dynamics of sulfide ion generations and eliminations inside the cell. A sensitive method with a large dynamic range means that it will be capable of following the gaseous transmitter for in-depth studies.
During the past decade, noble metal nanoparticles (NPs) have received extensive attentions for a wide variety of applications in biological sensing and imaging,23–25 catalysis,26 photothermal therapy,27 and surface-enhanced Raman scattering (SERS),28,29 because of their unique LSPR effect. When excited by a particular frequency of incident radiation, the free electrons in the conduction band of metal nanoparticles undergo coherent oscillations, which results in their unique scattering and absorption optical phenomenon.25 LSPR characters strongly depend on the shape, size and compositions of nanoparticles and the surrounding dielectric environment.30,31 Compared to those used in fluorescent sensing, plasmonic nanomaterials used in LSPR biosensing are more sensitive, non-toxic, and do not suffer from background suppression. The LSPR scattering spectroscopy of a single plasmonic nanoparticle (PNP) could be easily performed by dark-field microscopy and a microspectral analyzer, and the notable spectral shifts indicate the refractive index of the microenvironment surrounding an individual nanoparticle.32,33
NaHS is a weak acid salt and has a dynamic equilibrium with two forms (HS− and S2−) in aqueous solutions. The presence of S2− ions and Ag crystals could produce a chemical reaction to form an Ag2S precipitate. Several Au–Ag core–shell NPs based on LSPR sensing, have been developed to detect H2S based on this principle.22,34 He et al. have synthesized Au–Ag core–shell nanospheres34 and used them as colorimetric probes for the recognition of H2S through color RGB (red/green/blue) analysis of the color of PNPs. According to our group's previous results, when target miRNA-21 hybridized with probe ssDNA is immobilized on Ag nanocube surface, the cubic Ag nanoparticles exhibit more sensitivity to the tiny changes in the refractive index around the nanoparticle.35,36 Therefore, the Au@Ag core–shell nanocubes (NCs) exhibit a sufficient signal shift in LSPR peak and notable color change, which could be used as an optical plasmonic nanosensor. In this paper, a single Au@Ag NC as the sensor was prepared for the detection of trace sulfide ions by LSPR technology. We successfully monitored the process of Ag2S formation by LSPR spectra in real time at single particle level. In the presence of different concentrations of NaHS, the peak positions in LSPR scattering spectra of individual Au@Ag sensors red shift to longer wavelengths. Moreover, there is a linear relationship between the Δλmax and the sulfide ion concentrations over a large range from nM to μM, with a lower LOD up to 0.04 nM. The high sensitivity of this probe will achieve success in broad applications in detection and real-time monitoring of trace sulfide ions in real samples.
Results and discussion
The Au@Ag NCs had better dispersions in aqueous suspensions than Ag cubes.35,37 More importantly, they had a “silver cubic sensitivity” which means that tiny changes in the surrounding environment of the nanoparticle surface will lead to more shifts in the signals of LSPR scattering spectra. As shown in Scheme 1, in the presence of sulfide ions, the activated Ag atoms on nanoparticle surface reacted with S2− to generate Ag2S. There was a great difference between the refractive index of Ag (∼0.06) and Ag2S (∼3.08) at 509 nm,38,39 which resulted in a notable red-shift of the Au@Ag NC nanoparticle's scattering to a longer wavelength and a color change from cyan to dark red. On the basis of these results, Au@Ag NCs can be used as a new nanosensor to detect H2S.
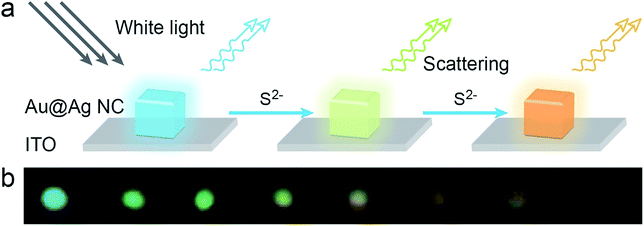 |
| Scheme 1 (a) The scheme of sulfide ions induced color change on a single Au@Ag NC. (b) The color changes of Au@Ag NC after reacting with different concentrations of NaHS. | |
Au@Ag NCs were prepared by the seed growth method (see ESI† for more details). Fig. S1a and S1b† are the TEM images of the Au nanospheres and Au@Ag NCs, respectively. The average size of the prepared Au@Ag NCs containing a 30 nm Au nanosphere core was 50.2 ± 1.8 nm (see Fig. S1a, S1b and S2†). The extinction spectra of 30 nm Au nanospheres, Au@Ag NCs, and Au@Ag@Ag2S NCs solutions are shown in Fig. S1c.† After being coated by the Ag cube, there was a blue-shift in the extinction spectrum, which exhibited the unique optical property of Ag NCs.40 Then, the extinction spectra red-shifted to longer wavelengths upon the formation of Ag2S on the nanocube surface in sulfide ion solution with a certain concentration. Fig. S1d† illustrates the SEM image of the Au@Ag@Ag2S NCs which were mixed with 1 μM NaHS for a certain time; sulfurization occurred at the sharp vertices and edges of NCs, which agrees with the reported results by Wang41 et al.
With the help of DFM system, the single Au@Ag NC could be employed to monitor the sulfurization process in the presence of NaHS in real time. As shown in Fig. 1a, four random Au@Ag nanosensors were selected to monitor the sulfurization process. Because of the high activity edges of NC, the peak positions of scattering spectrum red-shifted rapidly at the preliminary stage, and there was a remarkable slowdown of the reaction speed after 10 minutes. Meanwhile, the scattering peak red-shifted to a different wavelength (Δλmax), which was related to the concentration of NaHS at different times (Fig. 1b). There was a good linear dependence between the peak shift and the sulfide ions concentration, which indicated that the Au@Ag NC could be used as a plasmonic nanosensor for the detection of sulfide ions.
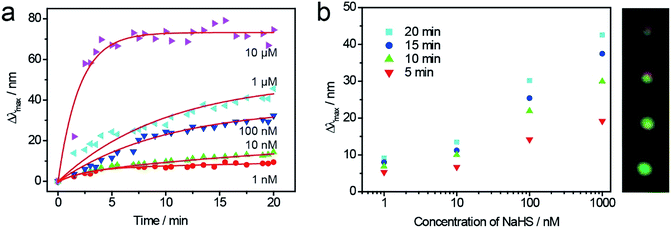 |
| Fig. 1 (a) Measured and calculated (red lines) time-dependent spectral shifts of single Au@Ag nanoparticles exposed to concentrations of 1 nM to 10 μM NaHS. (b) Calculated Δλmax values at various time points as a function of applied NaHS concentration. The illustration is the color of the particles after the reaction. | |
Fig. 2 shows the XRD spectra of the different samples. The sharp peaks at 38–65° can be assigned to (111), (200) and (220), which correspond to cubic Ag (JCPDS 89-3722). The distinctive peaks at 30–35° can be assigned to (
12), (
21) and (022) reflections of monoclinic Ag2S (JCPDS no. 14-0072), and they also appear in the XRD spectrum of Au@Ag@Ag2S NCs. These results indicate that the sulfurization process on Au@Ag NCs surface induced the peak shift of LSPR scattering spectra and color change.
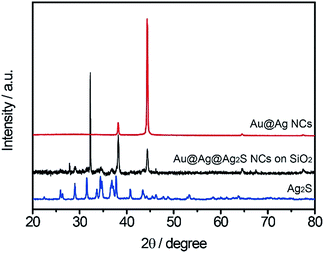 |
| Fig. 2 XRD spectra of the Au@Ag NCs (red), Au@Ag@Ag2S (black), and Ag2S (blue). | |
To investigate the stability of the Au@Ag NCs nanosensors for detection of sulfide ions at single particle level, a large quantity of LSPR scattering experiments were repeated at the same concentration of S2− for statistical analyses. Au@Ag NCs were immobilized on an ITO glass substrate with different concentrations of NaHS solutions for 25 min, and their spectra and color images were obtained. Fig. 3a and b show the color change of Au@Ag NCs before and after the treatment with 1 μM NaHS. During the sulfurization process, the NCs' color changed from cyan to dark red under dark-field microscopy. At the same concentration of NaHS, several LSPR nanosensors were randomly selected to observe the changes in their LSPR scattering spectra (Fig. 3c). The Δλmax values were apparently linear within a large range of NaHS concentrations from 0.1 nM to 1 μM (Fig. 3d). The limit of detection was calculated to be 0.04 nM when the ratio of S/N was 3. Meanwhile, the probes appeared in different colors, as shown in Scheme 1b, in the corresponding concentrations of NaHS; thus, it could be used for colorimetric detection.
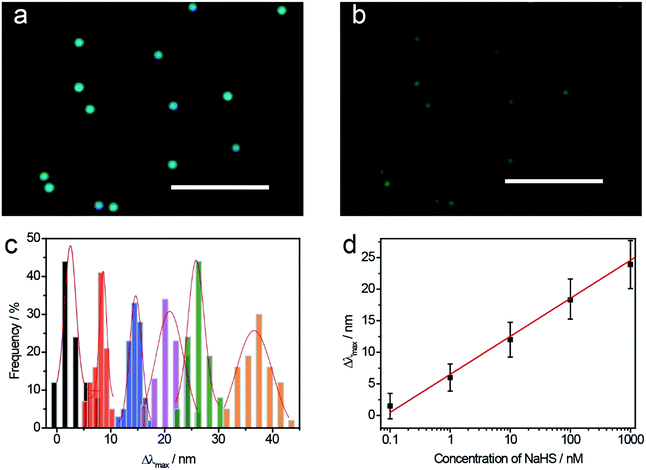 |
| Fig. 3 Dark-field images of Au@Ag nanosensors before (a) and after (b) the treatment with 1 μM NaHS. The scale bar is 10 μM. (c) Distributions of Au@Ag nanosensor LSPR peak redshift with the addition of 0.1 nM (black); 1 nM (red); 10 nM (blue); 100 nM (magenta); 1000 nM (green); and 10 μM (orange) NaHS. Over 60 particles were counted in each case. The red lines are Gaussian distributions of maximum peak (Δλmax) red-shift values. (d) The line fitting curve of the average of the Δλmax versus NaHS concentration. | |
To verify the selectivity of Au@Ag nanosensor for detection of sulfide ions, different interfering ions (Fig. 4) were selected to perform the same experiment at the same condition. Fig. 4 shows the selectivity test results of LSPR scattering spectra shift in the presence of NaCl, Na2CO3, NaNO3, L-cysteine and NaHS, respectively. There was no remarkable color change with presents of Cl−, CO32− and NO3−, which indicated that the nanosensors could be used for trace sulfide ion detection with high selectivity. LSPR scattering spectra peak position exhibited a slight blue shift. This result might be attributed to the slight RI change around the nanosensors, caused by the damage of the bilayer of CTAB on Au@Ag NC surface by charged ions. Moreover, there was only 5–8 nm red-shift in the presence of a 10-fold concentration of HS− because of the L-cysteine absorption on the Au@Ag NC plasmonic nanosensor surface. The results indicated that this LSPR nanosensor can be used for the detection of sulfide ions with high selectivity.
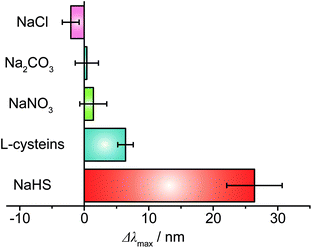 |
| Fig. 4 Selectivity tests for sulfide ions and other anions. Legend: NaHS (5 μM); L-cysteine (50 μM); NaNO3 (50 μM); Na2CO3 (50 μM); and NaCl (50 μM). | |
Conclusions
This study introduces a new method for real-time monitoring of the sulfurization process, occurring on an individual Au@Ag NC surface, and the LSPR scattering spectra red-shifts to longer wavelengths in the presence of different concentrations of sulfide ions. In addition, this process can be easily observed by the color change from the DFM images. These results can be used to design novel plasmonic nanosensors for detection of trace sulfide ions. There is a linear dependence between the LSPR scattering spectra red-shift and different concentrations of sulfide ions ranging from 0.1 nM to 1 μM. Moreover, this plasmonic method demonstrates more sensitivity (LOD: 0.04 nM) and selectivity. With the developments in LSPR, nanosensors at nano scale can be expected to detect other molecules for chemical and biological applications.
Conflicts of interest
There are no conflicts to declare.
Acknowledgements
This work was supported by the National Natural Science Foundation of China (61571239, 21475064, 21674048), the Sci-tech Support Plan of Jiangsu Province (BE2014719), the Program for Changjiang Scholars and Innovative Research Team in University (IRT_15R37), the Scientific Research Foundation of Nanjing University of Posts and Telecommunications (NY215173), and the Priority Academic Program Development of Jiangsu Higher Education Institutions (YX03001).
Notes and references
- A. Salvi, P. Bankhele, J. M. Jamil, M. Kulkarni-Chitnis, Y. F. Njie-Mbye, S. E. Ohia and C. A. Opere, Neurochem. Res., 2016, 41, 1020–1028 CrossRef CAS PubMed.
- V. S. Fernandes, A. S. Ribeiro, P. Martinez, M. E. Lopez-Oliva, M. V. Barahona, L. M. Orensanz, A. Martinez-Saenz, P. Recio, S. Benedito, S. Bustamante, A. Garcia-Sacristan, D. Prieto and M. Hernandez, PLoS One, 2014, 9, e113580 Search PubMed.
- Y. Sun, Y. Huang, W. Yu, S. Chen, Q. Yao, C. Zhang, D. Bu, C. Tang, J. Du and H. Jin, Oncotarget, 2017, 8, 31888–31900 Search PubMed.
- Q. Chai, T. Lu, X. L. Wang and H. C. Lee, Pflügers Archiv, 2015, 467, 329–340 CrossRef CAS PubMed.
- N. Hu, M. Dong and J. Ren, Am. J. Physiol. Regul. Integr. Comp. Physiol., 2014, 306, R761–R771 CrossRef CAS PubMed.
- G. Tang, L. Zhang, G. Yang, L. Wu and R. Wang, Diabetologia, 2013, 56, 533–541 CrossRef CAS PubMed.
- J. L. Wallace, R. W. Blackler, M. V. Chan, G. J. Da Silva, W. Elsheikh, K. L. Flannigan, I. Gamaniek, A. Manko, L. Wang, J. P. Motta and A. G. Buret, Antioxid. Redox Signaling, 2015, 22, 398–410 CrossRef CAS PubMed.
- A. Xuan, D. Long, J. Li, W. Ji, M. Zhang, L. Hong and J. Liu, J. Neuroinflammation, 2012, 9, 202–213 CAS.
- I. Andreadou, E. K. Iliodromitis, T. Rassaf, R. Schulz, A. Papapetropoulos and P. Ferdinandy, Br. J. Pharmacol., 2015, 172, 1587–1606 CrossRef CAS PubMed.
- H. Yang, Y. Zhang, L. Li, G. Sun, L. Zhang, S. Ge and J. Yu, Biosens. Bioelectron., 2017, 87, 53–58 CrossRef CAS PubMed.
- K. Wang, H. Peng, N. Ni, C. Dai and B. Wang, J. Fluoresc., 2014, 24, 1–5 CrossRef CAS PubMed.
- N. Adarsh, M. S. Krishnan and D. Ramaiah, Anal. Chem., 2014, 86, 9335–9342 CrossRef CAS PubMed.
- L. A. Montoya, T. F. Pearce, R. J. Hansen, L. N. Zakharov and M. D. Pluth, J. Org. Chem., 2013, 78, 6550–6557 CrossRef CAS PubMed.
- Y. Zhao, X. Zhu, H. Kan, W. Wang, B. Zhu, B. Du and X. Zhang, Analyst, 2012, 137, 5576–5580 RSC.
- E. Bitziou, M. B. Joseph, T. L. Read, N. Palmer, T. Mollart, M. E. Newton and J. V. Macpherson, Anal. Chem., 2014, 86, 10834–10840 CrossRef CAS PubMed.
- M. Deng, M. Zhang, X. Huang, J. Ma, L. Hu, G. Lin and X. Wang, Journal of Forensic and Legal Medicine, 2015, 32, 59–63 CrossRef PubMed.
- S. Chen, Z. J. Chen, W. Ren and H. W. Ai, J. Am. Chem. Soc., 2012, 134, 9589–9592 CrossRef CAS PubMed.
- C. Liu, B. Peng, S. Li, C. M. Park, A. R. Whorton and M. Xian, Org. Lett., 2012, 14, 2184–2187 CrossRef CAS PubMed.
- L. E. Santos-Figueroa, C. de la Torre, S. El Sayed, F. Sancenón, R. Martínez-Máñez, A. M. Costero, S. Gil and M. Parra, Eur. J. Inorg. Chem., 2014, 2014, 41–45 CrossRef CAS.
- K. Sasakura, K. Hanaoka, N. Shibuya, Y. Mikami, Y. Kimura, T. Komatsu, T. Ueno, T. Terai, H. Kimura and T. Nagano, J. Am. Chem. Soc., 2011, 133, 18003–18005 CrossRef CAS PubMed.
- T. Liu, Z. Xu, D. R. Spring and J. Cui, Org. Lett., 2013, 15, 2310–2313 CrossRef CAS PubMed.
- B. Xiong, R. Zhou, J. Hao, Y. Jia, Y. He and E. S. Yeung, Nat. Commun., 2013, 4, 1708–1717 CrossRef PubMed.
- J. Li, J. Liu and C. Chen, ACS Nano, 2017, 11, 2403–2409 CrossRef CAS PubMed.
- G. Doria, J. Conde, B. Veigas, L. Giestas, C. Almeida, M. Assuncao, J. Rosa and P. V. Baptista, Sensors, 2012, 12, 1657–1687 CrossRef CAS PubMed.
- P. K. Jain, X. Huang, I. H. El-Sayed and M. A. El-Sayed, Acc. Chem. Res., 2008, 41, 1578–1586 CrossRef CAS PubMed.
- K. K. Haldar, S. Kundu and A. Patra, ACS Appl. Mater. Interfaces, 2014, 6, 21946–21953 CAS.
- B. Jang, J. Y. Park, C. H. Tung, I. H. Kim and Y. Choi, ACS Nano, 2011, 5, 1086–1094 CrossRef CAS PubMed.
- L. Pei, Y. Ou, W. Yu, Y. Fan, Y. Huang and K. Lai, J. Nanomater., 2015, 16, 1–8 Search PubMed.
- L. Guerrini and D. Graham, Chem. Soc. Rev., 2012, 41, 7085–7107 RSC.
- J. Wirth, F. Garwe, J. Bergmann, W. Paa, A. Csaki, O. Stranik and W. Fritzsche, Nano Lett., 2014, 14, 570–577 CrossRef CAS PubMed.
- Y. Li, C. Jing, L. Zhang and Y. T. Long, Chem. Soc. Rev., 2012, 41, 632–642 RSC.
- L. Shi, C. Jing, W. Ma, D.-W. Li, J. E. Halls, F. Marken and Y.-T. Long, Angew. Chem., Int. Ed., 2013, 52, 6011–6014 CrossRef CAS PubMed.
- R. Qian, Y. Cao and Y.-T. Long, Angew. Chem., Int. Ed., 2016, 55, 719–723 CrossRef CAS PubMed.
- J. Hao, B. Xiong, X. Cheng, Y. He and E. S. Yeung, Anal. Chem., 2014, 86, 4663–4667 CrossRef CAS PubMed.
- L. Zhang, Y. Zhang, Y. Hu, Q. Fan, W. Yang, A. Li, S. Li, W. Huang and L. Wang, Chem. Commun., 2015, 51, 294–297 RSC.
- Y. Hu, L. Zhang, Y. Zhang, B. Wang, Y. Wang, Q. Fan, W. Huang and L. Wang, ACS Appl. Mater. Interfaces, 2015, 7, 2459–2466 CAS.
- Q. Zhang, W. Li, C. Moran, J. Zeng, J. Chen, L. P. Wen and Y. Xia, J. Am. Chem. Soc., 2010, 132, 11372–11378 CrossRef CAS PubMed.
- P. B. Johnson and R. W. Christy, Phys. Rev. B, 1972, 6, 4370–4379 CrossRef CAS.
- J. M. Bennett, J. L. Stanford and E. J. Ashley, J. Opt. Soc. Am., 1970, 60, 224–231 CrossRef CAS.
- G. Park, D. Seo, J. Jung, S. Ryu and H. Song, J. Phys. Chem. C, 2011, 115, 9417–9423 CAS.
- C. Fang, Y. H. Lee, L. Shao, R. Jiang, J. Wang and Q. H. Xu, ACS Nano, 2013, 7, 9354–9365 CrossRef CAS PubMed.
Footnote |
† Electronic supplementary information (ESI) available: Experimental details, Au@Ag NCs synthesis, immobilization of the PNPs. See DOI: 10.1039/c7ra12779k |
|
This journal is © The Royal Society of Chemistry 2018 |
Click here to see how this site uses Cookies. View our privacy policy here.