DOI:
10.1039/C7RA13086D
(Paper)
RSC Adv., 2018,
8, 3910-3918
Controllable synthesis of raspberry-like PS–SiO2 nanocomposite particles via Pickering emulsion polymerization†
Received
6th December 2017
, Accepted 15th January 2018
First published on 22nd January 2018
Abstract
This paper presents a simple and controllable method for the synthesis of monodisperse nanometer-sized organic–inorganic raspberry-like polystyrene (PS)–SiO2 nanocomposite particles (NCPs) via Pickering emulsion polymerization, by simply using a silane coupling agent, 3-(trimethoxysilyl)propyl methacrylate (MPS), as an auxiliary monomer and controlling its hydrolysis/condensation processes and amount. In this method, when MPS was stirred in acidic water with styrene (St) for a period of time, and then a basic silica solution added, raspberry-like PS–SiO2 NCPs were directly obtained after the polymerization. The whole process needs neither surface treatment for the silica particles nor additional surfactants or stabilizers. We propose that a silica-stabilized Pickering emulsion is formed through Si–OH reaction between the hydrolysis/condensation products of MPS distributed on the St droplets surface and the silica particles.
Introduction
Over the past few years, raspberry-like organic–inorganic hierarchical structured nanocomposite particles (NCPs), which consist of smaller corona particles placed over larger core particles with fascinating characteristics, such as unique morphology, higher surface roughness, large specific surface areas, and light scattering, have attracted substantial research interests. Principally, such particles can utilize the characteristic nanostructure to exhibit some new exciting physical–chemical properties, giving them good potential in applications such as catalysis, self-assembly, and construction of superhydrophobic, superhydrophilic coatings or other materials with specific UV-shielding, optical, and electrical properties.1–18
Generally, two strategies have been developed to fabricate the raspberry-like organic–inorganic NCPs: (i) larger core particles are synthesized in advance, and then small corona particles are anchored or formed in situ onto the core particles by covalent bonding,19 hydrogen bonding,20,21 acid–base,22 and electrostatic interactions,23,24 or through seeded emulsion polymerization.25,26 (ii) Larger core particles are monomer droplets with small corona particles adsorbed at fluid interface to form Pickering emulsion, followed by polymerization.27–30
Among the above-mentioned methods, the Pickering emulsion polymerization has drawn particular attention due to their excellent droplet stability, less foam and promising in practical applications.31 Typically, the single-particle wetting properties at the fluid interfaces hold the key to forming particle-stabilized Pickering emulsion. The contact angle of the interface with the solid particles at 90° is the optimum stabilization condition of the Pickering emulsion system.32–34 Generally, commonly-used inorganic particles are hydrophilic, while monomers are usually hydrophobic. Although some hydrophilic inorganic particles could be directly used to construct Pickering emulsion, the obtained raspberry-like NCPs are usually polydispersed in the range of several to several tens of micrometers.35,36 Some inorganic particles could be pre-modified to tune the wettability to form uniform Pickering emulsion.37,38 However, this pre-modification is very tedious and energy-consuming, and the particle modification degree, that reflects the wettability is difficult to be precisely controlled.39,40 Another efficient strategy to prepare raspberry-like NCPs is adding some auxiliary comonomers to improve the compatibility of organic and inorganic phases by directly link the two phase together. For instance, Armes et al. used basic 4-vinylpyridine (4-VP) as the auxiliary monomer to enhance the interaction between silica and polymer to produce raspberry-like NCPs.41,42 Wu et al. used a basic comonomer 1-vinylimidazole (1-VID)22 and a cationic comonomer 2-(methacryloyl)ethyltrimethylammonium chloride (MTC)24 as the auxiliary monomers, and successfully synthesized a series of long-stand stable raspberry-like NCPs. They both used the acid–base interaction between the silanol groups (acidic) of silica surfaces and amino groups (basic) of 4-VP or 1-VID and the strong electrostatic interaction between positively charged MTC and negatively charged silica to connect the two phase together. Nevertheless, the choosing of these auxiliary comonomers are very rigorous, as no such NCPs could be formed by using another related basic (co)monomer 2-vinylpyridine (2-VP).42 Thus, it is important to develop some new and general comonomers and convenient means to effectively bind the particle stabilizers to the monomer droplets for making stable, narrowly size-distributed Pickering emulsions.
As 3-(trimethoxysilyl)propyl methacrylate (MPS) is a very cheap silane coupling agent and can effectively modify various types of hydrophilic inorganic particles, it has become one of the most commonly used auxiliary monomers to improve the compatibility of organic and inorganic phases in the synthesis of organic–inorganic NCPs.43 However, it is seldom used directly as an auxiliary comonomer to construct Pickering emulsion for synthesis of raspberry-like NCPs because the wetting properties of the inorganic particles are difficult to be controlled by the directly MPS modification for satisfying the wetting conditions to form a stable particle-stabilized Pickering emulsion.
Herein, we report a simple synthesis of raspberry-like organic–inorganic PS–SiO2 NCPs based on silica-stabilized Pickering emulsion directly using MPS as the auxiliary comonomer through introducing pre-hydrolysis and subsequent promoted condensation processes to MPS. In this method, when MPS was stirred with acidic water and styrene (St) for a period of time, and added basic silica solution, monodisperse raspberry-like PS–SiO2 NCPs with nanometer-distributed size are directly obtained after polymerization. The effects of hydrolysis/condensation processes and amount of MPS, the sonication process, and the size of SiO2 on the morphology of the NCPs were investigated. Compared to the previously reported strategies, our proposed method is rather mild. Accordingly, we would believe that the advanced pre-hydrolysis and subsequent promoted condensation processes of MPS to tune the wettability of MPS products to bind the inorganic particles on the surface of organic droplets to form Pickering emulsion could open a new pathway and possibility for the rational design and synthesis of other raspberry-like NCPs.
Experimental section
Materials
Styrene (St, 99.0%), MPS (97.0%), acetic acid (HAc, 99.7%), ammonia solution (NH3·H2O, 25 wt%), triethylamine (TEA, 99.0%) and 2,2-azobis(isobutyronitrile) (AIBN, 98.0%) were purchased from Aladdin Chemical Reagent Corp. and used as received. LS50C40 (50 nm silica sol, 40 wt% aqueous dispersion) and LS20C30 (20 nm silica sol, 30 wt% aqueous dispersion) were supplied by Shandong Peak-tech New Material Co. (China), and diluted in water to 1 wt%. Deionized water was used for all polymerization and treatment processes.
Synthesis of raspberry-like PS–SiO2 NCPs
The raspberry-like PS–SiO2 NCPs were synthesized via Pickering emulsion polymerization. Typically, MPS was first added to a 250 mL four-neck round-bottom flask containing 80 mL HAc solution (pH ≈ 4) and stirred in an ice bath for 5 min for the pre-hydrolysis of MPS. Then, St (2 g) and AIBN (0.04 g) were added, followed by gently stirring at room temperature for 2 h or by sonication for 30–90 min. Subsequently, 20 mL of 50 nm silica sol dispersion was mixed with 0.3 mL TEA, and then was injected into the flask under stirring with a syringe pump (120 mL h−1). The mixed solution was degassed with nitrogen for 30 min under stirring, and then heated to 75 °C to initiate the polymerization. The reaction lasted for 10 h to obtain raspberry-like PS–SiO2 NCPs. The detailed experimental conditions and results are summarized in Table 1.
Table 1 Summary of experimental conditions and results
Runs |
MPS (mL) |
Silica size (nm) |
TEA (mL) |
Stirring time (min) |
Particle sizea/nm (DLS) |
The particle concentration for the measurement of DLS is 0.2 wt%. |
1 |
0.1 |
50 |
0.3 |
120 |
Aggregation |
2 |
0.2 |
50 |
0.3 |
120 |
Aggregation |
3 |
0.4 |
50 |
0.3 |
120 |
971 |
4 |
0.6 |
50 |
0.3 |
120 |
712 |
5 |
0.8 |
50 |
0.3 |
120 |
607 |
6 |
0.4 |
50 |
0.1 |
120 |
Aggregation |
7 |
0.4 |
50 |
0.5 |
120 |
Aggregation |
|
|
|
|
Sonication time (min) |
|
8 |
0.4 |
50 |
0.3 |
30 |
520 |
9 |
0.4 |
50 |
0.3 |
60 |
426 |
10 |
0.4 |
50 |
0.3 |
90 |
335 |
11 |
0.4 |
20 |
0.3 |
60 |
Aggregation |
Characterization
Scanning electron microscope (SEM) images were obtained with a Philips XL 30 field emission microscope at an accelerating voltage of 20 kV. All SEM samples were sputter coated with gold before observation. Transmission electron microscope (TEM) images were taken with a Hitachi H-800 transmission electron microscope (Japan) operated at 100 kV. The dispersions were diluted with deionized water and then dried onto carbon-coated copper grids before examination. Fourier transformation infrared spectroscopy (FTIR) spectra were scanned on a Nicolet Nexus 470 FTIR spectrometer with powder-pressed KBr pellets. The morphology of Pickering emulsion was characterized using HIROX KH-7700 digital microscope. Dynamic light scattering (DLS, Beckman Coulter Co.) measurements were carried out on the diluted reaction solutions to obtain the average diameter of the particles.
Results and discussion
Pickering emulsion stabilized by silica particles with the assistance of pre-hydrolyzed MPS
In the present work, firstly, MPS was pre-hydrolyzed for 5 min under acidic solutions in an ice bath; secondly, the hydrolyzed MPS molecules were stirred with St for 120 min to form a stable monomer dispersion; thirdly, the resulting monomer dispersion was mixed with a basic dispersion of silica particles to form a Pickering emulsion; finally, well-defined raspberry-like PS–SiO2 NCPs were efficiently produced through Pickering emulsion polymerization.
The acidic catalyzed pre-hydrolysis and basic catalyzed condensation processes of MPS play a vital role in the efficient formation of raspberry-like NCPs. As a control experiment, MPS was directly mixed with silica particles without pre-hydrolysis process. As shown in Fig. S1,† many plain polymer particles were produced, irrespective of the amounts of silica particles, St, and MPS, as well as the emulsification time. Under acidic conditions, the (CH3O)3–Si– groups of MPS molecules are inclined to hydrolyze into metastable Si–OH groups and further self-condense into low-molecular-weight linear and cyclic oligomers.44–47 Normally, the condensation extent is relatively low under acidic conditions, and therefore, many silanol groups are remained in the silica oligomers.48 Due to the coexistence of hydrophobic methacrylate groups and hydrophilic silanol ones, the silica oligomers display an amphiphilic property and are prone to be distributed on the surface of St monomer droplets,49–54 functioning as emulsifiers to form a stable milky white emulsion (Fig. 1a). According to the microscopic observation, the monomer droplets with a number-average particle size of 3.25 ± 3.14 μm were stably dispersed in the resulting emulsion (Fig. 1b).
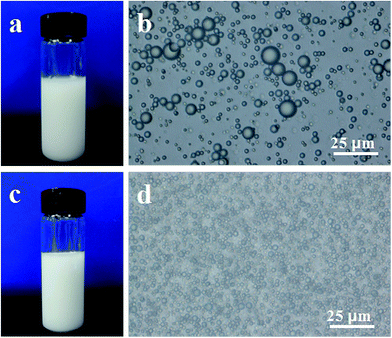 |
| Fig. 1 (a) The emulsion obtained after the pre-hydrolysis process of MPS and stirring with monomer St for 2 h, and (b) its optical image, (c) the emulsion obtained after adding basic silica sol, and (d) its optical image (run 3 in Table 1). | |
Addition of a basic dispersion of silica particles did not obviously influence the colloidal stability of the milk emulsion (Fig. 1c). Furthermore, a close microscopic observation showed that the diameter of the emulsion droplets significantly decreased to about 2.09 ± 0.45 μm (Fig. 1d). The size decrement may be attributed to the adsorption of silica particles onto the surface of monomer droplets that acted as colloidal stabilizer to stabilize the monomer droplets, the more the silica amount, the smaller the mean diameters of the monomer droplets.55–57
The detailed mechanism for the adsorption of silica particles onto the monomer droplets will be discussed in the following part of the article.
Preparation of raspberry-like PS–SiO2 NCPs through Pickering emulsion polymerization
Raspberry-like NCPs were prepared through polymerization in the Pickering emulsion (Fig. 2). According to the SEM images in Fig. 2a and b, the as-synthesized NCPs were covered with a dense layer of small silica nanoparticles, displaying a rugged surface morphology. The TEM image further confirmed the formation of the raspberry-like structure (Fig. 2c). DLS result showed in Fig. 2d indicated that the raspberry-like NCPs had an average diameter of 426 nm and a narrow size distribution with the polydisperse index of 0.108, indicating that the particles are relatively monodisperse without aggregation.
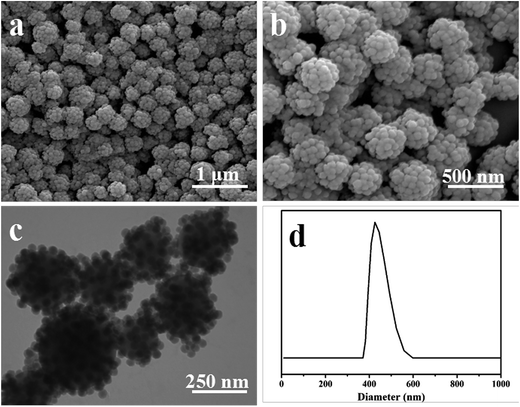 |
| Fig. 2 Typical (a and b) SEM and (c) TEM images, and (d) intensity size distribution of the as-obtained raspberry-like PS–SiO2 NCPs (run 9 in Table 1). | |
Variables influencing the particle properties of PS–SiO2 NCPs
(a) Effect of the amount of MPS. Fig. 3 and S2† showed the SEM images of the as-obtained NCPs as a function of the amount of MPS. When 0.1 mL of MPS was used, many free silica particles aggregated together and most of the PS particles were not covered by silica as shown in Fig. S2a and b.† When the amount of MPS was increased to 0.2 mL, most of the PS cores were covered by silica particles, displaying a raspberry-like structure (Fig. S2c and d†). However, the colloidal stability of this dispersion was still poor, and many aggregates of the NCPs and silica particles appeared in the SEM images. When the amount of MPS exceeded 0.4 mL, stable colloidal dispersion solution of raspberry-like NCPs could be prepared. With the increase of the MPS amount from 0.4 mL, 0.6 mL, to 0.8 mL, the size of NCPs decreased from 970 nm, 712 nm, to 607 nm (by DLS) (Fig. 3a–f). These results indicated that the MPS amount play a vital role in improving colloidal stability of raspberry-like NCPs, and controlling the particle size of NCPs. This could be possibly explained as follows: the hydrolyzed products of MPS are mainly distributed on the surface of St droplets, acting as emulsifiers to stabilize the St droplets. Small amount of MPS is insufficient to provide enough silanol groups on the surface of the St droplets to adsorb more silica particles to stabilize Pickering emulsions. With the increase of MPS, more hydrolyzed products of MPS would be produced. They can interact with more SiO2 particles, and consequently, with the adsorption of more SiO2 NPs, the colloidal stability of the dispersion could be improved. Furthermore, the particle size of the raspberry-like NCPs also decreased with the increase of the MPS amount. This could be again ascribed to the improved protection capability with more hydrolyzed products of MPS.58–61 As the amount of pre-hydrolyzed MPS products that acted as the emulsifiers stabilizing the monomer droplets increases, the diameter of the particles would decrease.55
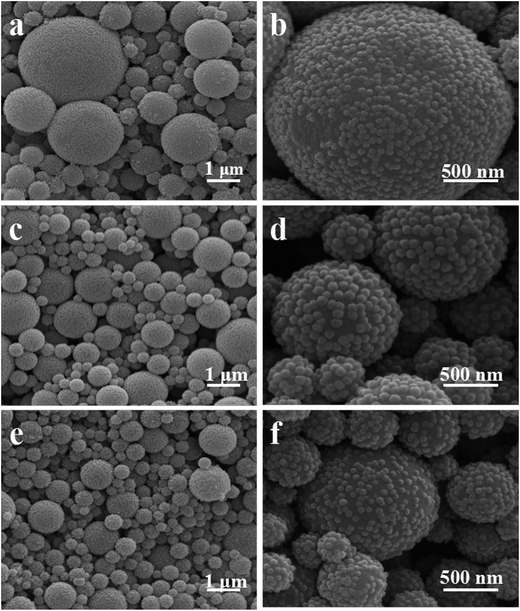 |
| Fig. 3 SEM images of the as-obtained PS–SiO2 NCPs with pre-hydrolysis of different amounts of MPS: (a and b) 0.4 mL, (c and d) 0.6 mL, (e and f) 0.8 mL. | |
The FTIR spectra of the pure silica particles and silica particles obtained by centrifugation, washing and then drying when the Pickering emulsion polymerization reaction was not carried out, are indicated in Fig. 4. The FTIR spectrum of the pure silica particles displays absorbance peaks at 1096 and 955 cm−1, respectively indicative of the stretching vibration of Si–O–Si bonds and Si–OH vibration. The presence of the new absorption bands at 1730 cm−1, which was attributed to the stretching absorption of C
O bonds, indicating MPS has been indeed grafted on the surface of silica particles. As the surface wettability of the nanoparticle is though to be the key controlling factor for particle-based emulsifiers in stabilizing oil-in-water Pickering emulsions, the hydrolyzed MPS molecules connected the hydrophilic silica particles to the interface of hydrophobic monomer St droplets.
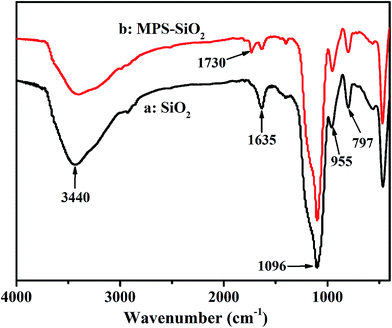 |
| Fig. 4 FTIR spectra of the (a) pure silica sol particles, (b) silica sol particles obtained after centrifuging the Pickering emulsion droplets. | |
(b) Amount of TEA. The amount of TEA displayed an obvious influence on the particle morphology and colloidal stability of the final products (Fig. 5). Insufficient (0.1 mL) or excessive (0.5 mL) TEA led to serious aggregation of NCPs. Moreover, the silica particles only sparsely attached to the surface of PS spheres when 0.1 mL of TEA was used (Fig. 5b), most probably due to the insufficient interaction between the silica particles and PS spheres.
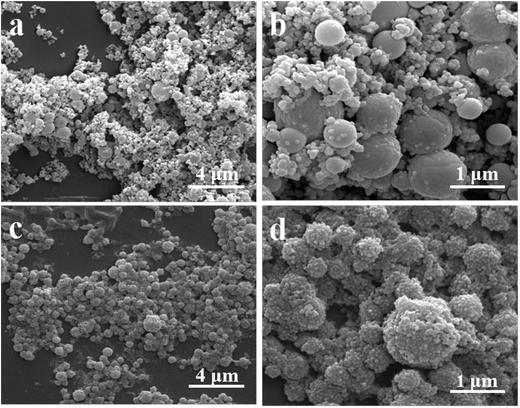 |
| Fig. 5 SEM images of the as-obtained PS–SiO2 NCPs with different amounts of TEA added to the silica solution: (a and b) 0.1 mL, (c and d) 0.5 mL. | |
This may be ascribed to the basic catalyzed process, which would affect the condensation reaction between the Si–OH groups on the silica surface and the pre-hydrolyzed MPS on the surface of St droplets. The condensation reaction highly depended on the added amount of TEA, too little (0.1 mL) or too much (0.5 mL) TEA would lead to aggregation of the NCPs, only 0.3 mL TEA is appropriate, under which the pH is 9.3. Normally, the condensation reaction extent is highly promoted under basic condition, the Si–OH condensation reaction are slow under low pH to connect enough silica particles to bind to the surface of St droplets, and very fast under high pH to adsorb too much silica particles, both of them could lead to aggregation of the NCPs.
(c) Effect of the size of silica. As discussed in the previous sections, silica particles, as colloidal stabilizer, were adsorbed onto the surface of St monomer droplets through the condensation reaction between the silanol groups of silica particles and the hydrolyzed products of MPS on the monomer droplets. In order to understand the influence of the particle size of silica particles on the formation of the NCPs, silica particles with two different particle sizes were used to prepare raspberry-like NCPs. When the silica particles with 50 nm in diameter were used, TEM (Fig. 6a and b) indicated clearly raspberry-like structure with silica particles distributed dispersedly on the surface of the polymer particles with narrow size distribution and kept stable. In contrast, when the silica particles with 20 nm in diameter were used, large amount of coagulum were observed (Fig. 6c and d), indicating their poor stabilization power. This phenomenon can be explained as follows: for one individual silica particles, a 50 nm silica particle has 15.6 times the weight as a 20 nm silica particle, thus when the same weight of silica particles were used, the total number of 20 nm silica is 15.6 times as the 50 nm silica. Since silica particles were adsorbed via condensation reaction with Si–OH of MPS on the surface of the emulsion droplets, the amount of adsorbed silica particles are nearly the same at a given amount of MPS. Thus, too many 20 nm silica particles are available compared to 50 nm silica, which cause the instability of the reaction system, leading to the aggregation of the raspberry-like NCPs.
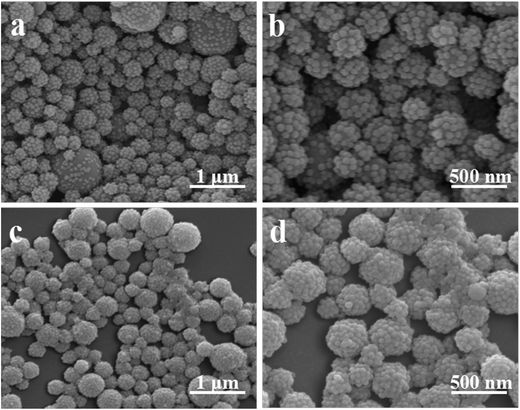 |
| Fig. 6 SEM images of the as-obtained PS–SiO2 NCPs with varied silica size (a and b) 50 nm, (c and d) 20 nm. | |
(d) Sonication conditions. When the MPS was pre-hydrolyzed in the acidic water for 5 min and stirred with St for 120 min, the raspberry-like NCPs could be successfully obtained. However, the particle size distribution of NCPs was relatively broad. Instead of stirring for 120 min, the dispersion was sonicated for various time durations. Promisingly, the raspberry-like NCPs with a smaller particle sizes and a more homogenous particle size distribution were prepared (Fig. 7). Regarding the particle size, with the increase of the sonication time from 30, 60, to 90 min, the Z-average particle size of NCPs decreased from 520, 426, to 335 nm.
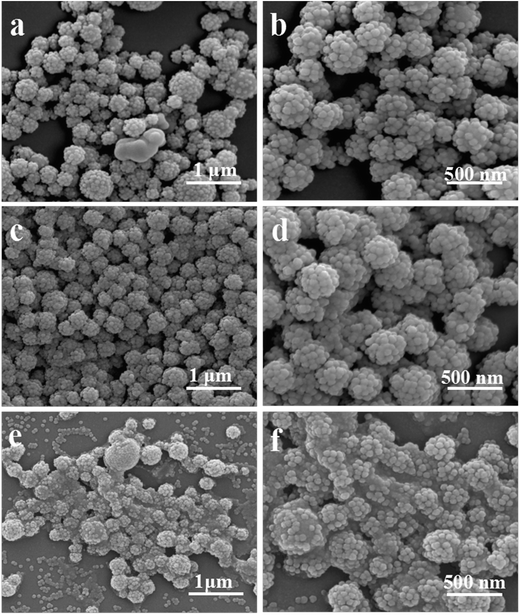 |
| Fig. 7 SEM images of the as-obtained PS–SiO2 NCPs with different sonication time: (a and b) 30 min, (c and d) 60 min, (e and f) 90 min. | |
As shown in Fig. 7a and b, the particle size of most of the raspberry-like NCPs was in the range of 320–350 nm when the sonication time was 30 min. However, a small amount of NCPs with 250 nm could also be observed. With increasing the sonication time to 60 min, the raspberry-like NCPs displayed a very good dispersion nature and much more uniform in size (Fig. 7c and d). Surprisingly, with further to 90 min (Fig. 7e and f), many free silica particles were observed. As the assistant of sonication can provide high energy densities to the reaction system and cause the big droplets deformation and disruption into smaller droplets.61 The longer the sonication, the more energy could be transferred to the emulsion droplets and the smaller the size of the droplets, thus decreasing the mean diameter of the obtained raspberry-like NCPs. However, if the sonication time was too long to produce much smaller droplets, the amount of MPS on each individual surface of monomer droplets would be not enough to adsorb the given amount of MPS.
Formation of the raspberry-like PS–SiO2 NCPs
To understand the mechanism by which these raspberry-like PS–SiO2 NCPs form, a control experiment in the absence of MPS was carried out. A metastable and flocculated milky-like dispersion was obtained. The SEM image shown in Fig. 8a indicated that only some bare PS particles and free silica beads coexisted, no PS–SiO2 raspberry-like or other NCPs were observed. However, when MPS was pre-hydrolyzed and introduced to the reaction system, raspberry-like PS–SiO2 NCPs were obtained as seen in Fig. 8b. In the meantime, another experiment was carried out without the pre-hydrolysis of the MPS, as illustrated in Fig. S1,† only bare polymer particles and free silica particles existed. This suggests that the pre-hydrolysis process of MPS is playing the key role for the formation of raspberry-like NCPs, it can improve the connection between the organic St phase with inorganic silica particles.
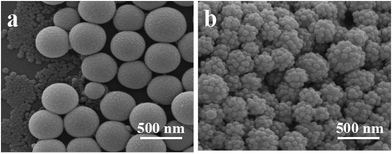 |
| Fig. 8 SEM images of as-obtained particles (a) without MPS, (b) with MPS. | |
Based on all the experimental results and discussion, we could propose a possible formation mechanism of the raspberry-like PS–SiO2 NCPs, as shown in Scheme 1. Under acidic condition, MPS molecules underwent hydrolysis reactions, producing hydrophilic or amphiphilic Si–OH groups enriched low-molecular-weight linear and even cyclical oligomers.44 These amphiphilic Si–OH enriched oligomers are prone to distribute on the surfaces of the St monomer emulsion droplets,62 which are easily to react with the ultrafine silica particles with many Si–OH groups on their surfaces under basic promoted condensation reaction. Thus, without any added surfactants or polymeric stabilizers, the silica particles herein acted as emulsifiers to stabilize the monomer droplets with the assistant of pre-hydrolyzed MPS. After the temperature was risen, the oil-soluble initiators AIBN dissolved in monomer droplets would decompose and generated free radicals, which initiated a “bulk” polymerization of monomer and MPS molecules in the silica-stabilized emulsion droplets, the MPS molecules connect the silica particles and organic phase and finally formed raspberry-like NCPs.
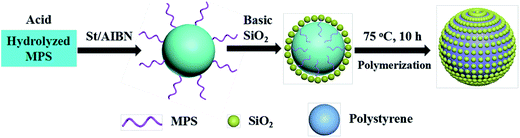 |
| Scheme 1 Schematic illustration of the formation of the raspberry-like PS–SiO2 NCPs. | |
Conclusion
In the present study, a monodisperse nanometer-sized raspberry-like PS–SiO2 NCPs were successfully synthesized via a Pickering emulsion polymerization by using MPS as the auxiliary monomer. The average particle sizes, size distribution, and morphology of the NCPs could be easily controlled by adjusting the pre-hydrolysis and condensation processes of MPS, the amount of MPS, silica size, and sonication time. The mechanism of the formed raspberry-like structures could be elucidated by the acid-catalyzed pre-hydrolyzed amphiphilic products of MPS molecules which distributed on the surface of St monomer droplets and basic catalyzed condensation reaction to adsorb the silica to the surface of the St monomer droplets. As many nanoparticles are easily to be modified with MPS, this method we present here would be readily extended for synthesis of versatile raspberry-like NCPs with various components, such as TiO2, Fe3O4, Al2O3, ZrO2, to exhibit some specific properties and applications. It is believed that these raspberry-like structures NCPs obtained by Pickering emulsion polymerization, are good candidates for the construction of functional colloidal coatings and complex colloidal architectures in large-scale.
Conflicts of interest
There are no conflicts to declare.
Acknowledgements
Financial supports of this research from the National Natural Science Foundation of China (Grants 51703203), the Zhejiang Provincial Natural Science Foundation of China (LQ17E030004), Science Foundation of Zhejiang Sci-Tech University (ZSTU) under Grant No. 16012172-Y, Zhejiang Provincial Top Key Academic Discipline of Chemical Engineering and Technology of Zhejiang Sci-Tech University (CETT2016004), The Young Researchers Foundation of Zhejiang Provincial Key Laboratory of Advanced Textile Materials and Manufacturing Technology, Zhejiang Sci-Tech University (2016QN09) are appreciated.
Notes and references
- J. Hu, M. Chen and L. M. Wu, Polym. Chem., 2011, 2, 760–772 RSC.
- S. Reculusa, C. Mingotaud, E. Bourgeat-Lami, E. Duguet and S. Ravaine, Nano Lett., 2004, 4, 1677–1682 CrossRef CAS.
- S. Reculusa, C. Poncet-Legrand, S. Ravaine, C. Mingotaud, E. Duguet and E. Bourgeat-Lami, Chem. Mater., 2002, 14, 2354–2359 CrossRef CAS.
- X. G. Qiao, M. Chen, J. Zhou and L. M. Wu, J. Polym. Sci., Part A: Polym. Chem., 2007, 45, 1028–1037 CrossRef CAS.
- Z. Li, V. Ravaine, P. Garrigue and A. Kuhn, Adv. Funct. Mater., 2007, 17, 618–622 CrossRef CAS.
- T. J. Yao, C. X. Wang, J. Wu, Q. Lin, H. Lv, K. Zhang, K. Yu and B. Yang, J. Colloid Interface Sci., 2009, 338, 573–577 CrossRef CAS PubMed.
- Y. C. Liu, M. L. Li and G. F. Chen, J. Mater. Chem. A, 2013, 1, 930–937 CAS.
- D. Z. Xu, M. Z. Wang, X. W. Ge, M. H. W. Lam and X. P. Ge, J. Mater. Chem., 2012, 22, 5784–5791 RSC.
- W. Ming, D. Wu, R. Van Benthem and G. De With, Nano Lett., 2005, 5, 2298–2301 CrossRef CAS PubMed.
- Z. Qian, Z. C. Zhang, L. Y. Song and H. R. Liu, J. Mater. Chem., 2009, 19, 1297–1304 RSC.
- R. P. M. Höller, M. Dulle, S. Thoma, M. Mayer, A. M. Steiner, S. Forster, A. Fery, C. Kuttner and M. Chanana, ACS Nano, 2016, 10, 5740–5750 CrossRef PubMed.
- Z. H. Cao, H. N. Chen, S. D. Zhu, Z. J. Chen, C. Xu, D. M. Qi and U. Ziener, Colloids Surf., A, 2016, 489, 223–233 CrossRef CAS.
- X. Y. Li and J. H. He, ACS Appl. Mater. Interfaces, 2013, 5, 5282–5290 CAS.
- W. J. Jiang, C. M. Grozea, Z. Q. Shi and G. J. Liu, ACS Appl. Mater. Interfaces, 2014, 6, 2629–2638 CAS.
- F. P. Dong, H. B. Xie, Q. Zheng and C. S. Ha, RSC Adv., 2017, 7, 6685–6690 RSC.
- E. Bourgeat-Lami and M. Lansalot, Adv. Polym. Sci., 2010, 233, 53–123 CrossRef CAS.
- Z. Li, V. Ravaine, S. Ravaine, P. Garrigue and A. Kuhn, Adv. Funct. Mater., 2007, 17, 618–622 CrossRef CAS.
- X. Y. Li and J. H. He, ACS Appl. Mater. Interfaces, 2013, 5, 5282–5290 CAS.
- J. Y. Wang and X. F. Yang, Langmuir, 2008, 24, 3358–3364 CrossRef CAS PubMed.
- J. Y. Wang and X. L. Yang, Colloid Polym. Sci., 2008, 286, 283–291 CAS.
- H. Minami, Y. Mizuta and T. Suzuki, Langmuir, 2013, 29, 554–560 CrossRef CAS PubMed.
- M. Chen, L. M. Wu, S. X. Zhou and B. You, Macromolecules, 2004, 37, 9613–9619 CrossRef CAS.
- W. S. Choi, H. Y. Koo and W. T. S. Huck, J. Mater. Chem., 2007, 17, 4943–4946 RSC.
- M. Chen, S. X. Zhou, B. You and L. M. Wu, Macromolecules, 2005, 38, 6411–6417 CrossRef CAS.
- R. K. Wang, H. R. Liu and F. W. Wang, Langmuir, 2013, 29, 11440–11448 CrossRef CAS PubMed.
- E. Bourgeat-Lami and J. Lang, J. Colloid Interface Sci., 1998, 197, 293–308 CrossRef CAS PubMed.
- T. Chen, P. J. Colver and S. A. F. Bon, Adv. Mater., 2007, 19, 2286–2289 CrossRef CAS.
- Z. H. Cao, A. Schrade, K. Landfester and U. Ziener, J. Polym. Sci., Part A: Polym. Chem., 2011, 49, 2382–2394 CrossRef CAS.
- A. Schrade, Z. H. Cao, K. Landfester and U. Ziener, Langmuir, 2011, 27, 6689–6700 CrossRef CAS PubMed.
- K. L. Chen, S. X. Zhou, S. Yang and L. M. Wu, Adv. Funct. Mater., 2015, 25, 1035–1041 CrossRef CAS.
- A. Schrade, K. Landfester and U. Ziener, Chem. Soc. Rev., 2013, 42, 6823–6839 RSC.
- T. Young, An essay on the cohesion of fluids, Philos. Trans. R. Soc. London, 1805, 95, 65–87 CrossRef.
- P. Pieranski, Phys. Rev. Lett., 1980, 45, 569–572 CrossRef CAS.
- M. Zanini, C. Marschelke, S. E. Anachkoy, E. Marini, A. Synytska and L. Isa, Nat. Commun., 2017, 8, 15701 CrossRef CAS PubMed.
- T. Chen, P. J. Colver and S. A. F. Bon, Adv. Mater., 2007, 19, 2286–2289 CrossRef CAS.
- M. J. Percy and S. P. Armes, Langmuir, 2002, 18, 4562–4565 CrossRef CAS.
- D. J. Voorn, W. Ming and A. M. Van Herk, Macromolecules, 2006, 39, 2137–2143 CrossRef CAS.
- J. N. Zhang, X. W. Ge, M. Z. Wang, J. J. Yang, Q. Y. Wu, M. Y. Wu, N. N. Liu and Z. T. Jin, Chem. Commun., 2010, 46, 4318–4320 RSC.
- B. P. Binks and S. O. Lumsdon, Langmuir, 2000, 16, 8622–8631 CrossRef CAS.
- G. E. Yakubov, O. I. Vinogradova and H. J. Butt, J. Adhes. Sci. Technol., 2000, 14, 1783–1799 CrossRef CAS.
- C. Barthet, A. J. Hickey, D. B. Cairns and S. P. Armes, Adv. Mater., 1999, 11, 408–410 CrossRef CAS.
- M. J. Percy, C. Barthet, J. C. Lobb, M. A. Khan, S. F. Lascelles, M. Vamvakaki and S. P. Armes, Langmuir, 2000, 16, 6913–6920 CrossRef CAS.
- S. Kongo, S. Kalia, A. Celli, J. Njuguna, Y. Habibi and R. Kumar, Prog. Polym. Sci., 2013, 38, 1232–1261 CrossRef.
- S. Savard, L. P. Blanchard, J. Leonard and R. E. Prudhomme, Polym. Compos., 1984, 5, 242–249 CrossRef CAS.
- F. D. Osterholtz and E. R. Pohl, J. Adhes. Sci. Technol., 1992, 6, 127–149 CrossRef CAS.
- J. Sefcik and A. V. McCormick, Catal. Today, 1997, 35, 205–223 CrossRef CAS.
- E. Bourgeat-Lami, I. Tissot and F. Lefebvre, Macromolecules, 2002, 35, 6185–6191 CrossRef CAS.
- C. J. Brinker and G. W. Scherer, Sol–Gel Science: The Physics and Chemistry of Sol–Gel Processing, Academic, New York, 1990 Search PubMed.
- K. F. Ni, N. Sheibat-Othman, G. R. Shan, G. Fevotte and E. Bourgeat-Lami, Macromolecules, 2005, 38, 9100–9109 CrossRef CAS.
- K. F. Ni, G. R. Shan, Z. X. Weng, N. Sheibat-Othman, G. Fevotte, F. Lefebvre and E. Bourgeat-Lami, Macromolecules, 2005, 38, 7321–7329 CrossRef CAS.
- Z. H. Cao, G. R. Shan, G. Fevotte, N. Sheibat-Othman and E. Bourgeat-Lami, Macromolecules, 2008, 41, 5166–5173 CrossRef CAS.
- Y. X. Li, Z. Q. Wang, Z. Huang, Y. F. Pan and G. Xue, J. Mater. Chem., 2010, 20, 5516–5520 RSC.
- Z. D. Lu, L. Sun, K. Nguyen, C. B. Gao and Y. D. Yin, Langmuir, 2011, 27, 3372–3380 CrossRef CAS PubMed.
- Y. Y. Sun, M. Chen, S. X. Zhou, J. Hu and L. M. Wu, ACS Nano, 2015, 9, 12513–12520 CrossRef CAS PubMed.
- T. Yamamoto, M. Nakayama, Y. Kanda and K. Higashitani, J. Colloid Interface Sci., 2006, 297, 112–121 CrossRef CAS PubMed.
- Y. Cong, K. L. Chen, S. X. Zhou and L. M. Wu, J. Mater. Chem. A, 2015, 3, 19093–19099 CAS.
- Y. Cong, Q. J. Li, M. Chen and L. M. Wu, Angew. Chem., 2017, 129, 3606–3610 CrossRef.
- T. Yamamoto, M. Nakayama, Y. Kanda and K. Higashitani, J. Colloid Interface Sci., 2006, 297, 112–121 CrossRef CAS PubMed.
- A. R. Mahdavian and M. Abdollahi, Polymer, 2004, 45, 3233–3239 CrossRef CAS.
- Y. Y. Sun, Y. Y. Yin, M. Chen, S. X. Zhou and L. M. Wu, Polym. Chem., 2013, 4, 3020–3027 RSC.
- L. G. Gómez-Mascaraque and A. López-Rubio, J. Colloid Interface Sci., 2016, 465, 259–270 CrossRef PubMed.
- C. L. Winzor and D. C. Sundberg, Polymer, 1992, 33, 3797–3810 CrossRef CAS.
Footnote |
† Electronic supplementary information (ESI) available. See DOI: 10.1039/c7ra13086d |
|
This journal is © The Royal Society of Chemistry 2018 |
Click here to see how this site uses Cookies. View our privacy policy here.