DOI:
10.1039/C8RA01088A
(Paper)
RSC Adv., 2018,
8, 9822-9832
Linear-type carbazoledioxazine-based organic semiconductors: the effect of backbone planarity on the molecular orientation and charge transport properties†
Received
3rd February 2018
, Accepted 27th February 2018
First published on 13th March 2018
Abstract
We report the synthesis of a linear-type dibromocarbazoledioxazine (CZ) derivative as a new precursor for semiconducting polymers. The chemical structures of the CZ unit and its polymers with thiophene or thienothiophene spacers (namely, PCZT and PCZTT) were fully characterized. PCZT and PCZTT possessed similar medium optical band gap (Eoptg) and electrochemical band gap (Ecvg) of around 1.70 eV estimated from the onset absorption and electrochemical redox potentials of the thin films, respectively. Computational density functional theory (DFT) calculations suggested that the backbone of the PCZT might be highly twisted, while that of PCZTT could be very planar. The effect of different backbone geometries on the charge–transport properties was studied by using thin film transistors (TFTs). The TFT device based on PCZTT showed a four times higher hole mobility as compared to that based on PCZT. The superior TFT performances of PCZTT were reasonably attributed to its edge-on backbone packing orientations toward the Si substrate revealed by the grazing-incidence wide-angle X-ray scattering (GIWAXS), which was favorable for in-plane charge transport in the TFT devices.
Introduction
With their interesting optical and electrical properties, organic semiconductors are increasingly prominent in various electronic devices such as organic photovoltaics (OPVs)1–9 and organic thin film transistors (TFTs).10–18 In particular, due to their solution processability and mechanical flexibility, organic semiconductors would become fundamental components for novel mobile electronic devices, such as flexible displays, biocompatible sensors, and radio frequency identification (RFID) tags.19–21 In order to realize such next-generation devices, significant efforts have been devoted to the development of new organic semiconducting materials, some of which already demonstrated charge carrier mobilities beyond the benchmark of industry standard inorganic materials, such as amorphous silicon (0.1–1 cm2 V−1 s−1).22–25 One important strategy of designing the organic/polymeric semiconducting materials is to envision more promising building blocks.26–28 While the electron-donating building blocks have been intensively explored,29–31 the development of acceptor units lags behind because of the limited species and synthetic difficulties.32 Among the reported acceptor building blocks, dye/pigment molecules have received enormous interests. Diketopyrrolopyrrole (DPP),33 isoindigo (IID),34 and rylene diimide (such as naphthalenediimide (NDI) and perylenediimide (PDI))35–37 (Chart 1 top) are successful examples for the construction of high-performance semiconducting polymers with mobilities (μ) > 1 cm2 V−1 s−1 in organic TFTs and power conversion efficiencies (PCE) > 10% in OPVs.38
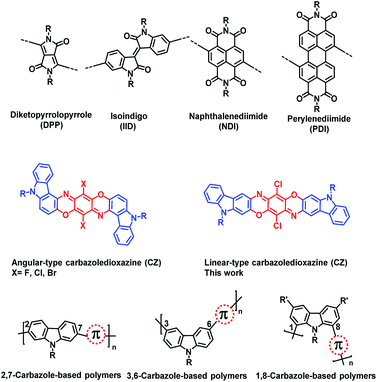 |
| Chart 1 Chemical structures of (top) well-known dye/pigment units used for high-performance semiconducting polymers; (middle) angular and linear-type carbazoledioxazines; (bottom) 2,7-carbazole-, 3,6-carbazole-, and 1,8-carbazole-based copolymers. | |
Among these dye/pigment molecules, the angular-type carbazoledioxazine (CZ, X = Cl, Chart 1 middle), also called pigment violet 23, is a well-known commercial pigment, but it has been less explored in the field of organic electronics.39–42 Note that this pigment is not only a member of the electron-accepting dioxazine family, but also originates from the electron-donating carbazole.43,44 Thus, the violet color is derived from the highly-efficient intramolecular charge-transfer (CT) band. The general synthesis of this pigment is based on the condensation of a 3-aminocarbazole derivative with p-chloranil followed by two-fold cyclization reactions. In the final cyclization process, simple heating selectively affords the angular-type CZ (Chart 1 middle). On the other hand, the introduction of the methoxy-substituent into the 2-position of the carbazole unit produces the linear-type CZ under the same conditions (Chart 1 middle).45,46 The electrochemical and optical properties of some CZ derivatives were investigated in the 1990s.47,48 However, there have been, to the best of our knowledge, no reports about their applications in organic electronic devices until our recent publication of the first TFTs based on newly-designed angular-type CZs with different halogen atoms (Chart 1 middle, X = F, Cl, Br).49 The TFT studies suggested that the CZ derivatives are relatively good p-type semiconductors with hole mobilities as high as 4.9 × 10−3 cm2 V−1 s−1. However, to the best of our knowledge, no studies have been reported so far for the synthesis of carbazoledioxazine-based polymers. Therefore, we became interested in incorporating the CZ unit into semiconducting polymers to further explore intriguing optical and electronic properties.
In view of the historical development of carbazole-based semiconducting polymers, the CZ molecular backbone shape was carefully designed. The linear-type 2,7-carbazole was previously shown to be a backbone component of semiconducting polymers superior to the angular-type 3,6-carbazole and 1,8-carbazole connectivities (Chart 1 bottom),50–57 although 1,8-carbazole-based copolymers recently found a promising application possibility as fluorescent polymer nanoparticles (Pdots).58,59 For instance, Leclerc et al. reported a 2,7-carbazole-based copolymer, namely poly(N-9-heptadecanyl)-2,7-carbazole-alt-5,5-(4,7-di-2-thienyl-2,1,3-benzothiadiazole) (PCDTBT), which demonstrated an excellent TFT mobility and OPV performances.51–53 We anticipated that the angular- and linear-type CZs are analogous to these carbazole connectivities. Therefore, we decided to adopt the linear-type CZ structure in this study, rather than the recently reported angular-type CZ unit. The linear-type dibromo-CZ was thus newly designed and synthesized via a multistep synthesis (Scheme 1). This bifunctional monomer was then reacted with ditin–thiophene or ditin–thienothiophene units by Stille polycondensation, producing two promising semiconductors, namely PCZT and PCZTT, respectively (Scheme 1). The frontier energy levels (highest occupied molecular orbital: HOMO; lowest unoccupied molecular orbital: LUMO) and band gaps of CZ and its polymers were determined by their optical and electrochemical properties. Most importantly, the backbone planarity was estimated from the computational calculations. There was a clear correlation between these physical properties and TFT performances. The TFTs based on PCZTT displayed a hole mobility as high as 1.8 × 10−3 cm2 V−1 s−1, which was four times higher than that based on PCZT. This difference in the TFT performances was mainly attributed to the different backbone planarity, which was reasonably supported by their different solid-state packing structures and thin-film morphologies revealed by their X-ray diffraction measurements and atomic force microscopy images.
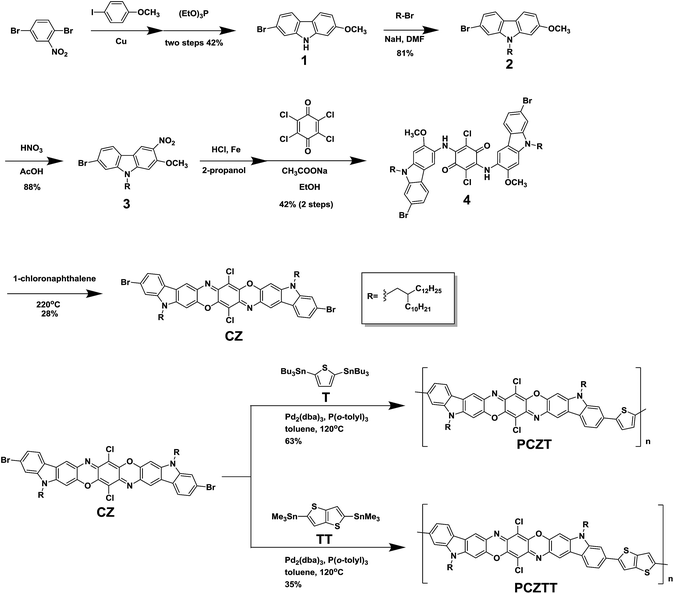 |
| Scheme 1 Synthesis of the linear-type dibromocarbazoledioxazine monomer and its Stille polycondensation to give the corresponding semiconducting oligomers. | |
Experimental
General measurements
Nuclear magnetic resonance (NMR) spectra were recorded using a JEOL model AL300 (300 MHz) at room temperature. Deuterated chloroform was used as the solvent. Chemical shifts of NMR were reported in ppm (parts per million) relative to the residual solvent peak at 7.26 ppm for 1H NMR spectroscopy and 77.6 ppm for 13C NMR spectroscopy. Coupling constants (J) were given in Hz. The resonance multiplicity was described as s (singlet), d (doublet), t (triplet), and m (multiplet). Fourier transform infrared (FT-IR) spectra were recorded on a JASCO FT/IR-4100 spectrometer in the range from 4000 to 600 cm−1. MALDI-TOF mass spectra of small molecules were measured on a Shimadzu/Kratos AXIMACFR mass spectrometer equipped with a nitrogen laser (λ = 337 nm) and pulsed ion extraction, which was operated in a linear-positive ion mode at an accelerating potential of 20 kV. Tetrahydrofuran (THF) solutions containing 1 g L−1 of a sample, 20 g L−1 of dithranol, and 1 g L−1 of sodium trifluoroacetate were mixed at a ratio of 1
:
1
:
1; and then 1 μL aliquot of this mixture was deposited onto a sample target plate. MALDI-TOF mass spectra of polymers were measured on a Bruker UltrafleXtreme mass spectrometer, which was operated in a linear-positive ion mode. Dithranol was used as a matrix. Elemental analysis was conducted at the Center for Advanced Materials Analysis, Tokyo Institute of Technology. The elements of C, H, and N were measured on a J-Science JM10, the elements of Cl, Br, and S on a Yanako HSU-20+ICS-1100, and the element of O on an Elementar Vario micro cube. UV-vis-NIR spectra were recorded on a JASCO V-670 spectrophotometer. Thermogravimetric analysis (TGA) and differential scanning calorimetry (DSC) measurements were carried out on a Rigaku TG8120 and a Rigaku DSC8230, respectively, under nitrogen flow at the scan rate of 10 °C min−1. Electrochemistry measurements were carried out on a BAS electrochemical analyzer model 612C at 20 °C in a classical three-electrode cell. The working, reference, and auxiliary electrodes were a glassy carbon electrode, Ag/AgNO3/CH3CN/(nC4H9)4NPF6, and a Pt wire, respectively. For calibration, the redox potential of ferrocene/ferrocenium (Fc/Fc+) was measured. It was assumed that the redox potential of Fc/Fc+ has an absolute energy level of −4.80 eV to vacuum. The HOMO and LUMO energy levels were then calculated according to the following equations: |
EHOMO = −(φox + φFc/Fc+ + 4.80) (eV)
| (1) |
|
ELUMO = −(φre + φFc/Fc+ + 4.80) (eV)
| (2) |
where φox is the onset oxidation potential vs. Ag/AgNO3, φre is the onset reduction potential vs. Ag/AgNO3, and φFc/Fc+ is the redox potential of ferrocene/ferrocenium vs. Ag/AgNO3 and it is −0.05 V in this study.
Fabrication and characterization of thin film transistors
Top-contact/bottom-gate TFT devices were fabricated using n+-Si/SiO2 substrates where n+-Si and SiO2 were used as the gate electrode and gate dielectric, respectively. The substrates were subjected to cleaning with deionized water, acetone, and 2-propanol. Then, the substrates were cleaned with O3 for 20 min using a Technovision model 208 UV-O3 cleaning system. The cleaned substrates were modified with octadecyltrimethoxysilane (OTMS) to form a self-assembled monolayer (SAM). Thin films of the CZ-based semiconductors were deposited on the treated substrate by spin-coating a chloroform solution (5 g L−1), followed by thermal annealing at 150, 200, 250, or 300 °C for 10 min in an argon-filled glove box. After the thin film deposition, ∼50 nm thick gold was deposited as source and drain contacts using a shadow mask. The TFT devices had a channel length (L) of 100 μm and a channel width (W) of 1 mm. The TFT performances were measured under ambient conditions using a Keithley 4200 parameter analyzer on a probe stage. The carrier mobilities, μ, were calculated from the data in the saturated regime according to the following equation: |
ISD = (W/2L)Ciμ(VGS − Vth)2
| (3) |
where ISD is the drain current in the saturated regime, W and L are the semiconductor channel width and length, respectively, Ci (Ci = 13.3 nF cm−2) is the capacitance per unit area of the gate dielectric layer, and VGS and Vth are the gate voltage and threshold voltage, respectively. VGS − Vth of the devices was determined from the square root values of ISD at the saturated regime. Current on/off ratios (Ion/Ioff) were determined from the minimum current at around VGS = +20 to −20 V (Ioff) and the current at VGS = −80 V (Ion). The transfer characteristics were obtained by sweeping the VGS from +20 to −80 V.
Grazing-incidence wide angle X-ray scattering (GIWAXS) measurements
The GIWAXS profiles were obtained by using a Phillips X'Pert-Pro-MRD with monochromated CuKα radiation (wavelength = 0.154 nm) operated at 45 kV and 40 mA in air. The samples were exposed to the X-ray beam for 1 h with an incident beam radius of 320 mm. The thin films for GIWAXS were prepared by spin-coating the polymer solutions on OTMS-treated Si/SiO2 substrates followed by thermal annealing at the optimized temperature for 10 min under vacuum.
Atomic force microscopy (AFM) measurements
AFM samples were prepared by spin-coating the polymer solutions on OTMS-treated Si/SiO2 substrates. Both pristine and thermally-treated films were examined by a Seiko Instruments SPA-400 with a stiff cantilever of Seiko Instruments DF-20.
Materials and synthetic details
All chemicals were purchased from Tokyo Chemical Industry (TCI), Kanto Chemical, and Sigma Aldrich and used as received unless otherwise stated. 2-Bromo-7-methoxy-9H-carbazole 1 and 11-(bromomethyl)tricosane were prepared according to a literature method.49,60
Synthesis of 2-bromo-9-(2-decyltetradecyl)-7-methoxy-9H-carbazole (2)
NaH (2.50 g, 60% dispersion in paraffin liquid) in dry DMF (80.0 mL) was slowly added to 2-bromo-7-methoxy-9H-carbazole (4.34 g, 15.7 mmol) under nitrogen. After stirring for 30 min, 11-(bromomethyl)tricosane (7.83 g, 20.8 mmol) was added and the mixture was stirred at room temperature for 1 day. The reaction mixture was quenched with H2O and extracted with CH2Cl2. Evaporation followed by column chromatography (SiO2, hexane/CH2Cl2 4
:
1) afforded the desired compound as colorless oil (7.83 g, 81%).
1H NMR (300 MHz, CDCl3): δ = 7.92 (d, J = 8.4 Hz, 1H), 7.84 (d, J = 8.4 Hz, 1H), 7.47 (d, J = 1.5 Hz, 1H), 7.30 (dd, J = 8.6, 1.3 Hz, 1H), 6.88 (dd, J = 8.6, 2.0 Hz, 1H), 6.82 (d, J = 2.2 Hz, 1H), 4.06 (d, J = 7.7 Hz, 2H), 3.94 (s, 3H), 2.10 (br, 1H), 1.23 (m, 40H), 0.90 (t, J = 6.6 Hz, 6H) ppm; 13C NMR (75 MHz, CDCl3): δ = 159.18, 142.38, 141.90, 121.89, 121.86, 120.94, 120.43, 117.75, 116.18, 111.75, 107.49, 93.63, 55.56, 47.66, 37.54, 31.92, 31.90, 31.73, 29.92, 29.66, 29.65, 29.60, 29.56, 29.37, 29.32, 29.49, 22.68, 14.12 ppm; IR (neat): ν = 2922, 2852, 1634, 1597, 1519, 1457, 1334, 1309, 1247, 1063, 825, 811, 718 cm−1; MALDI-TOF MS (Mw = 612.8): m/z = 612.6 [M+].
Synthesis of 7-bromo-9-(2-decyltetradecyl)-2-methoxy-3-nitro-9H-carbazole (3)
To a solution of 2 (5.34 g, 8.71 mmol) in acetic acid (80 mL), a mixture of fuming nitric acid (2.0 mL, 48.2 mmol) and acetic acid (100 mL) was dropwise added at room temperature for over 30 min. After saturated aqueous solution of sodium bicarbonate was added, the organic phase was extracted with CH2Cl2. Evaporation followed by column chromatography (SiO2, hexane/CH2Cl2 1
:
1) afforded the desired compound as yellow oil (5.02 g, 88%).
1H NMR (300 MHz, CDCl3): δ = 8.54 (s, 2H), 7.80 (d, J = 8.1 Hz, 2H), 7.71 (s, 2H), 7.48 (d, J = 1.5 Hz, 2H), 7.32 (dd, J = 8.2, 1.6 Hz, 2H), 6.80 (s, 2H), 4.08 (d, J = 7.3 Hz, 4H), 3.98 (s, 6H), 2.10 (br, 2H), 1.22 (m, 80H), 0.87 (t, J = 6.6 Hz, 12H) ppm; 13C NMR (75 MHz, CDCl3): δ = 153.71, 144.50, 142.80, 133.61, 123.88, 121.72, 121.35, 119.84, 119.60, 114.97, 112.82, 92.77, 56.84, 48.09, 37.71, 32.04, 32.01, 31.87, 30.08, 29.98, 29.82, 29.78, 29.75, 29.71, 29.66, 29.59, 29.54, 29.47, 29.43, 26.74, 26.62, 22.92, 22.86, 22.82, 14.36, 14.24 ppm; IR (neat): ν = 2922, 2852, 1634, 1597, 1519, 1457, 1334, 1308, 1247, 1063, 1028, 825, 811, 720 cm−1; MALDI-TOF MS (Mw = 657.8): m/z = 657.9 [M+].
Synthesis of 2,5-bis((7-bromo-9-(2-decyltetradecyl)-2-methoxy-9H-carbazol-3-yl)amino)-3,6-dichlorocyclohexa-2,5-diene-1,4-dione (4)
Iron dust (22.5 g, 0.403 mol) was added to water (30 mL) and aqueous HCl (10 N, 5 mL), and the mixture was stirred at 90 °C for 30 min under nitrogen. After a solution of 3 (8.64 g, 13.1 mmol) in 2-propanol (150 mL) was added, the mixture was refluxed for 5 h. After cooling to room temperature, water was added and the organic phase was extracted with CH2Cl2. Evaporation yielded 7-bromo-9-(2-decyltetradecyl)-2-methoxy-9H-carbazol-3-amine, and this compound was subjected to the next reaction without further purification. p-Chloranil (1.64 g, 6.67 mmol), sodium acetate (0.542 g, 6.61 mmol), and ethanol (200 mL) were added, and the mixture was refluxed under nitrogen for 5 h. After cooling to room temperature, water was added and the organic phase was extracted with CH2Cl2. Evaporation followed by column chromatography (SiO2, hexane/CH2Cl2 5
:
4) afforded the desired compound as a reddish-purple solid (3.95 g, two-step yield of 42%).
1H NMR (300 MHz, CDCl3): δ = 8.07 (s, 2H), 7.77 (d, J = 8.0 Hz, 2H), 7.32 (dd, J = 8.1, 1.6 Hz, 2H), 7.48 (d, J = 1.5 Hz, 2H), 7.32 (dd, J = 8.2, 1.6 Hz, 2H), 6.80 (s, 2H), 4.08 (d, J = 7.3 Hz, 4H), 3.98 (s, 6H), 2.10 (br, 2H), 1.22 (m, 80H), 0.87 (t, J = 6.6 Hz, 12H) ppm; 13C NMR (75 MHz, CDCl3): δ = 173.74, 153.56, 142.98, 141.99, 140.78, 122.59, 121.84, 120.90, 119.24, 118.46, 118.35, 114.42, 112.24, 102.71, 91.37, 56.09, 48.02, 37.84, 32.06, 32.02, 31.95, 30.50, 29.82, 29.79, 29.77, 29.73, 29.71, 29.49, 29.45, 26.66, 22.83, 14.25 ppm; IR (neat): ν = 3226, 2925, 2853, 1738, 1714, 1574, 1465, 1363, 1219, 755, 745 cm−1; MALDI-TOF MS (Mw = 1428.5): m/z = 1429.2 [M+].
Synthesis of 3,13-dibromo-8,18-dichloro-5,15-bis(2-decyltetradecyl)-5,15-dihydrocarbazolo[3′,2′:5,6][1,4]oxazino[2,3-b]indolo[2,3-i]phenoxazine (CZ)
A solution of 4 (0.442 g, 0.309 mmol) in 1-chloronaphthalene (8.0 mL) was heated to 220 °C for 26 h under nitrogen. After cooling to room temperature, the mixture was poured into methanol. The precipitate was collected by filtration and further purified by Soxhlet extraction with acetone for 24 h and the residue was finally extracted with chloroform. Further purification was performed by recycling preparative HPLC to yield the desired product as a violet solid (0.124 g, 28%).
1H NMR (300 MHz, CDCl3): δ = 8.07 (s, 2H), 7.77 (d, J = 8.0 Hz, 2H), 7.32 (dd, J = 8.1, 1.6 Hz, 2H), 7.20 (d, J = 1.4 Hz, 2H), 6.82 (s, 2H), 3.75 (d, J = 7.3 Hz, 4H), 1.95 (br, 2H), 1.24 (m, 80H), 0.88 (t, J = 6.6 Hz, 12H) ppm; 13C NMR (75 MHz, CDCl3): δ = 143.19, 142.82, 142.24, 142.18, 141.65, 128.82, 122.98, 121.39, 121.13, 121.12, 120.40, 119.66, 112.52, 109.89, 94.90, 48.23, 37.63, 32.08, 31.83, 30.14, 29.86, 29.84, 29.81, 29.54, 29.50, 26.62, 22.84, 14.27 ppm; MALDI-TOF MS (Mw = 1360.4): m/z = 1361.4 [M + H]+.
Synthesis of PCZT
CZ (204 mg, 0.150 mmol), 2,5-bis(tributylstannyl)thiophene (99.1 mg, 0.150 mmol), Pd2(dba)3 (6.0 mg, 6.6 μmol), and P(o-tolyl)3 (4.9 mg, 16 μmol) in toluene (15 mL) were refluxed for 2 days under nitrogen. After cooling to room temperature, the reaction mixture was poured into methanol (400 mL). The precipitate was collected by filtration and purified by Soxhlet extraction using methanol, acetone, hexane, and chloroform in this order. The chloroform soluble fraction was concentrated and reprecipitated into methanol, yielding a dark blue solid (193 mg, 63%).
GPC (eluent: o-dichlorobenzene): Mn = 6.2 kg mol−1, PDI = 2.5; 1H NMR (CDCl3, 300 MHz): δ = 8.01–5.50 (br, 12H), 5.02–3.10 (br, 4H), 2.00–0.60 (br, 47H) ppm; IR (neat): ν = 3068, 3018, 1574, 1457, 1434, 1219, 1127, 1036, 942, 772, 748, 660 cm−1; anal. calcd for (C82H110Cl2N4O2S)n: C, 76.54; H, 8.62; Cl, 5.51; N, 4.35; O, 2.49; S, 2.49; found: C, 73.41; H, 7.92; Br, 1.74; Cl, 5.32; N, 3.98; O, 4.35; S, 2.62.
Synthesis of PCZTT
CZ (244 mg, 0.179 mmol), 2,5-bis(trimethylstannyl)thieno[3,2-b]thiophene (81.9 mg, 0.179 mmol), Pd2(dba)3 (8.9 mg, 9.7 μmol), and P(o-tolyl)3 (5.3 mg, 17 μmol) in toluene (20 mL) was refluxed for 2 days under nitrogen. After cooling to room temperature, the reaction mixture was poured into methanol (400 mL). The precipitate was collected by filtration and purified with Soxhlet extraction using methanol, acetone, hexane, and chloroform in this order. The chloroform soluble fraction was concentrated and reprecipitated into methanol, yielding a dark blue solid (85.0 mg, 35%).
GPC (eluent: o-dichlorobenzene): Mn = 6.8 kg mol−1, PDI = 2.3; 1H NMR (CDCl3, 300 MHz): δ = 8.47–5.90 (br, 12H), 4.75–3.50 (br, 4H), 2.47–0.62 (br, 47H) ppm; IR (neat): ν = 2923, 2852, 1635, 1607, 1554, 1457, 1353, 1312, 1271, 1220, 1171, 1128, 1022, 906, 772 cm−1; anal. calcd for (C84H110Cl2N4O2S2)n: C, 75.13; H, 8.26; Cl, 5.28; N, 4.17; O, 2.38; S, 4.77; found: C, 72.95; H, 8.09; Br, 2.87; Cl, 4.96; N, 3.96; O, 3.37; S, 4.16.
Results and discussion
Synthesis and characterization
Starting from the commercially-available 1,4-dibromo-2-nitrobenzene, 2-bromo-7-methoxy-9H-carbazole (1) was obtained in the moderate yield of 42% in two steps according to a literature report.60 Alkylation at the 9-position followed by mono-nitration yielded 7-bromo-9-(2-decyltetradecyl)-2-methoxy-3-nitro-9H-carbazole (3) in high yield (two steps, total yield over 70%, Scheme 1). The reduction of 3 by hydrochloric acid and Fe powder afforded the corresponding 3-aminocarbazole derivative, and this compound was directly used for the next condensation reaction with p-chloranil (2,3,5,6-tetrachlorocyclohexa-2,5-diene-1,4-dione) due to the limited stability of amine compound in air. The resulting product (4) was further subjected to a ring closure reaction at high temperature (220 °C) in 1-chloronaphthalene as the solvent, leading to the target linear carbazoledioxazine monomer (CZ) in 28% isolated yield after multiple purifications by Soxhlet extraction and recycling preparative high performance liquid chromatography (HPLC). The chemical structure of the new bifunctional monomer was unambiguously characterized by 1H-, 13C-NMR, IR spectroscopies and MALDI-TOF mass spectrometry. Particular attention was paid to the isomeric structures of this product. The resonance multiplicity of a set of aromatic proton peaks was observed as a singlet (protons d and g in Fig. 1 or protons a and b in Fig. S1†), clearly suggesting the formation of the linear-type structure. This was also consistent with the reported spectral patterns of similar derivatives.45–48 In addition, after ring closure, the proton peaks of N–H and OCH3 as well as the IR peaks ascribed to the C
O and NH vibrations completely disappeared (Fig. 1 and S2†).
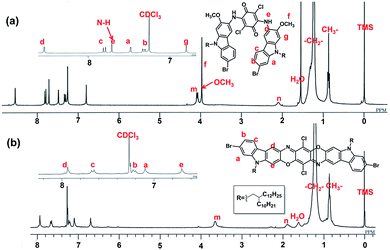 |
| Fig. 1 1H NMR spectra of (a) 4 and (b) CZ in CDCl3. | |
The linear-type dibromocarbazoledioxazine monomer (CZ) was then reacted with the ditin compounds of thiophene (T) and thienothiophene (TT) by the conventional Stille polycondensation to afford the target products, namely PCZT and PCZTT, respectively (Scheme 1). They were washed using a Soxhlet extractor with the sequence of methanol, hexane, and acetone to remove the low molecular weight oligomers. Finally, the chloroform soluble fractions were collected and were found to be reasonably soluble in a variety of chlorinated solvents, such as chloroform, chlorobenzene, and 1,2-dichlorobenzene at room temperature. Thus, the molecular weights (number-average molecular weight (Mn) and weight-average molecular weight (Mw)) were determined by gel permeation chromatography (GPC) measurements using polystyrene as the standard and 1,2-dichlorobenzene as the eluent at 40 °C. Both polymers showed similar molecular weights and polydispersity indices (PDI, Mw/Mn): PCZT (Mn = 6.2 kg mol−1, Mw = 15.5 kg mol−1, PDI = 2.5) and PCZTT (Mn = 6.8 kg mol−1, Mw = 15.4 kg mol−1, PDI = 2.3). The Mn values of PCZT and PCZTT suggested the pentamers (Table 1 and Fig. S3†). This was also supported by the comprehensive elemental analysis of both polymers. Importantly, a small amount of Br was detected (1.74% for PCZT and 2.87% for PCZTT). Under the assumption that the both terminal units are Br–CZ, the Mn was calculated to be 9.1 kg mol−1 for PCZT and 5.5 kg mol−1 for PCZTT, which were consistent with the GPC results. These results also suggested that the rate-determining step of the Stille polycondensation is oxidative addition. Note that we very recently developed a highly efficient Stille polycondensation protocol,61 but this protocol was not applied to this study in order to avoid the side reaction at the Cl moieties of CZ. Interpretation of the conjugated polymer structures by NMR is often difficult due to the significant peak broadening caused by strong intermolecular interactions and wide molecular weight distributions. In particular, in the case of PCZT and PCZTT, the CZ monomer possesses two kinds of halogen moieties, namely, Br and Cl. In order to confirm the selective reaction at the Br moieties, MALDI-TOF mass spectrometry was used as an additional tool for structure verification. As shown in Fig. 2, one could observe some sets of molecular ion peaks ascribed to the desired repeat unit structures. These spectra support the progress of the Stille polycondensation at the Br moieties of the CZ monomer and rule out the possibility of undesired side reactions at the Cl moieties. It should be noted that the peak intensities of the MALDI-TOF mass spectra significantly decrease as the molecular weights increase, although they are dependent on the sample purity, molecular weight distributions, and matrix and instrument settings.
Table 1 Molecular weights, optical and electrochemical properties of the CZ monomer and its polymers
|
Mna (g mol−1) |
PDIa |
DPa |
λsol (nm) |
λfilmb (nm) |
λonset (nm) |
Eoptgc (eV) |
φoxd (V) |
φred (eV) |
EHOMO (eV) |
ELUMO (eV) |
ECVg (eV) |
Determined by GPC. Cast onto the glass substrate. Optical band gap (Eoptg) estimated from the onset wavelength of the films. Thin films measured in CH3CN with 0.1 M (nC4H9)4NClO4 at the scan rate of 0.1 V s−1. Potentials vs. Fc/Fc+. |
CZ |
1364.4 |
— |
— |
554, 599 |
573, 629 |
702 |
1.77 |
0.52 |
−1.25 |
−5.27 |
−3.50 |
1.77 |
PCZT |
6200 |
2.5 |
5 |
389, 620 |
399, 663 |
744 |
1.67 |
0.42 |
−1.28 |
−5.17 |
−3.47 |
1.70 |
PCZTT |
6800 |
2.3 |
5 |
412, 624 |
433, 677 |
770 |
1.61 |
0.35 |
−1.29 |
−5.10 |
−3.46 |
1.64 |
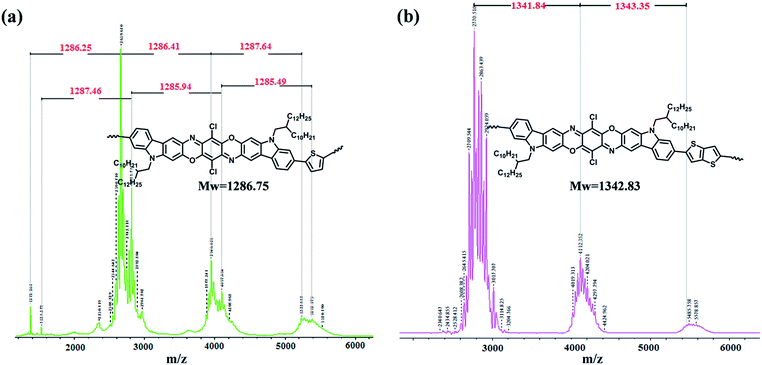 |
| Fig. 2 MALDI-TOF mass spectra of (a) PCZT and (b) PCZTT. | |
Thermal properties
The thermal stability of the polymers was estimated by thermogravimetric analysis (TGA) under a nitrogen atmosphere at the heating rate of 10 °C min−1 (Fig. S4†). Both PCZT and PCZTT exhibited a similar thermal stability profile with the 5% weight loss temperatures (Td) being ∼365 °C (365 °C for PCZT and 368 °C for PCZTT). The Td values were sufficiently high for advanced studies of the TFT fabrication, which requires optimization of the thermal annealing conditions. The thermal transition properties were also investigated by differential scanning calorimetry (DSC) measurements in the temperature range without thermal decomposition under N2 at the heating or cooling rate of 10 °C min−1 (Fig. S5†). Although no sharp transitions appeared, both polymers showed some endothermic and exothermic peaks during the heating and cooling processes, respectively. For example, PCZT displayed a transition at 208 °C in the second heating process, suggesting the existence of some different phases. PCZTT also showed a similar phase behavior.14,15
Optical and electrochemical properties
The absorption spectra of CZ, PCZT, and PCZTT in both solutions and thin films are shown in Fig. 3a. CZ displayed two absorption peaks with an optical band gap (Eoptg) of 1.77 eV estimated from the onset wavelength (λonset) of the film (Table 1). After reacting with the thiophene or thienothiophene co-monomer units, PCZT and PCZTT further extended the effective conjugation length, leading to red-shifted absorption with a narrower band gap of 1.67 and 1.61 eV, respectively.13 The absorption maxima in dilute 1,2-dichlorobenzene solution and in the thin film state (λsol and λfilm) of PCZTT bathochromically shifted as compared to those of PCZT. For example, the film absorption (λfilm) of PCZTT was detected at 433 and 677 nm, while the PCZT film showed the λfilm of 399 and 663 nm (Table 1). Accordingly, PCZTT possessed a longer λonset and narrower Eoptg than PCZT. This superior optical property of PCZTT was reasonably attributed to the stronger electron-donating nature of the thienothiophene unit, which elongates the effective conjugation length and raises the HOMO level.4,13
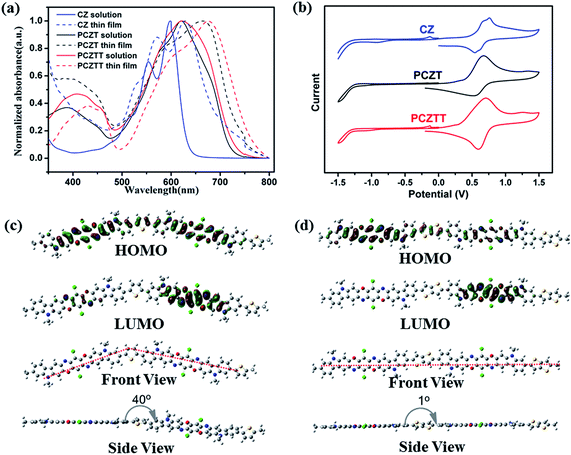 |
| Fig. 3 (a) UV-vis-NIR absorption profiles of CZ, PCZT, and PCZTT in dilute 1,2-dichlorobenzene solution and thin film states spin-cast on a glass substrate; (b) cyclic voltammograms of the CZ, PCZT, and PCZTT films drop-cast on a glassy carbon electrode, measured in acetonitrile with 0.1 M (nC4H9)4NClO4 at the scan rate of 0.1 V s−1; (c) calculated HOMO/LUMO orbitals and side-view geometry of PCZT; and (d) calculated HOMO/LUMO orbitals and side-view geometry of PCZTT (using DFT B3LYP/6-31G(d), long and branched alkyl chains are substituted by the methyl group). | |
To determine the frontier energy levels, cyclic voltammograms (CVs) were measured as shown in Fig. 3b and the corresponding data are listed in Table 1. Thin films of the samples were prepared on a glassy carbon electrode, and their CVs were measured in CH3CN with 0.1 M (nC4H9)4NClO4 at room temperature under an argon atmosphere.13 All three samples displayed a well-defined reversible oxidation peak and an irreversible reduction one. The oxidation currents were much higher than the corresponding reduction ones (Fig. 3b), indicating the more facilitated hole injection to these thin films.11,12 The highest occupied molecular orbital (EHOMO) and lowest unoccupied molecular orbital (ELUMO) values were estimated from the onset oxidation (φox) and onset reduction (φre) potentials, respectively. As listed in Table 1, the monomer CZ possessed the EHOMO/ELUMO of −5.27/−3.50 eV, which were comparable to its angular type counterparts.49 The thin film of PCZT showed the φox of 0.42 V and φre of −1.28 V, corresponding to the EHOMO value of −5.17 eV and the ELUMO value of −3.47 eV. The frontier energy levels of PCZTT were similarly determined. While the HOMO level of PCZTT was 0.07 eV higher than that of PCZT, the LUMO levels of both polymers were almost the same within experimental error (merely 0.01 eV). The electrochemical band gaps (ECVg) of both polymers, calculated from the φox and φre values, correlated with their Eoptg (Table 1). For example, the ECVg values of both polymers were 0.03 eV higher than the corresponding Eoptg.13
In order to further understand the optoelectronic properties and molecular geometry of the new polymers, density functional theory (DFT) calculations were performed using the dimer structures as the models with a B3LYP/6-31G(d) basis set.22 The N-alkyl substituents and alkyl side chains were simplified as a methyl group. The molecular orbitals (MOs) and side-view geometry are shown in Fig. 3c and d. One could observe that the HOMO orbitals were well distributed along the backbone, while the LUMO orbitals were located on one side (mainly on the acceptor units). This kind of HOMO/LUMO distribution well explains the CV results. The radical cations formed through the one-electron oxidation are stable due to the delocalization of the HOMO orbitals. On the other hand, the chemical stability of the radical anions formed through a one-electron reduction is limited due to the localized LUMO on the acceptor unit.13 Interestingly, there was an explicit difference in the optimized backbone geometry between PCZT and PCZTT. The PCZT dimer formed an angular-shape causing a twisted backbone with the dihedral angle of 40°. In contrast, the PCZTT dimer possessed an almost planar backbone due to the negligible steric repulsion between the adjacent repeat units (Fig. 3c and d bottom). These calculations are consistent with the experimental optical and electrochemical results.
Fabrication and measurements of thin film transistors
To further study the different backbone geometries effect on the charge transport properties, organic TFTs with bottom-gate/top-contact structures were fabricated by spin-coating the polymer solutions onto an octadecyltrimethoxysilane (OTMS)-treated SiO2 (300 nm)/n+-Si substrate.13,22 After thermal annealing at some selected temperatures (100, 150, 250, or 300 °C), ∼50 nm thick gold was deposited as the source and drain contacts using a shadow mask. As anticipated from the determined shallow HOMO (ca. −5.1 eV) and LUMO (ca. −3.5 eV) levels, both polymers showed unipolar p-type transistor performances (Fig. 4a to d). For example, the as-cast films of PCZT and PCZTT exhibited hole mobilities (μh) of 1.4 × 10−4 and 5.1 × 10−5 cm2 V−1 s−1, respectively (Fig. 4a and c and Table 2). Subsequently, we tried thermal annealing to optimize the TFT performances. Unexpectedly, the mobility of PCZT decreased to 3.5 × 10−5 cm2 V−1 s−1 after the thermal annealing at 100–150 °C, but it recovered to 1.4 × 10−4 cm2 V−1 s−1 when the thermal annealing temperature was raised to 250 °C (Fig. 4e and Table 2). The highest μh of 3.0 × 10−4 cm2 V−1 s−1 was achieved after thermal annealing at 300 °C (Fig. 4b and e and Table 2). On the other hand, the mobility of PCZTT had a linear correlation with the thermal annealing temperature (Fig. 4e). The highest μh of 1.4 × 10−3 cm2 V−1 s−1 was achieved with the annealing temperature of 300 °C (Fig. 4d). It should be noted that this value is relatively good, considering the amorphous thin film structures of these polymers (for the GIWAXS results, vide infra).11,12,57,62 Overall, PCZTT with a planar backbone demonstrated four times higher mobility than that of PCZT with a twisted backbone (Table 2). Further improvements of the mobilities would be possible by the combination with other prominent building blocks to enhance the intramolecular CT character and thin film crystallinity.63
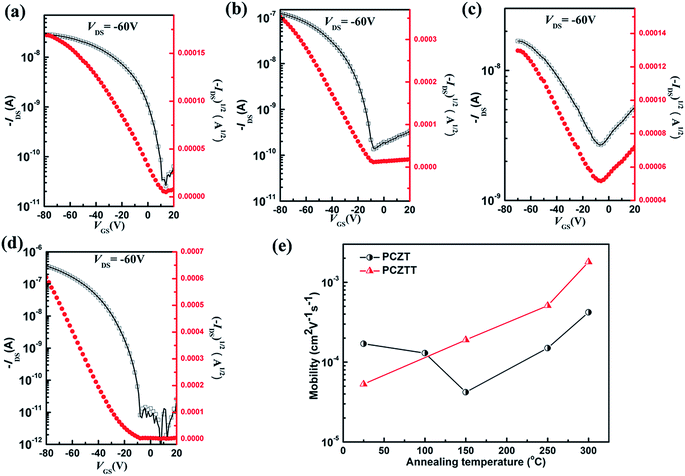 |
| Fig. 4 Transfer characteristics of TFTs based on the thin films of (a) PCZT without annealing; (b) PCZT annealed at the optimized temperature of 300 °C; (c) PCZTT without annealing; (d) PCZTT annealed at the optimized temperature of 300 °C (L = 100 μm and W = 1 mm. All the measurements were done under ambient conditions). (e) Effects of annealing temperatures on the hole mobilities of PCZT and PCZTT. | |
Table 2 Summary of TFT device performances
Sample/annealing temp. |
μh [μh_max]a (cm2 V−1 s−1) |
Vth (V) |
Ion/Ioff |
Mobility values are measured in air. The mobilities under optimized annealing conditions are indicated in boldface. The average values are calculated over 3 devices and the highest values are listed in parentheses. |
PCZT (N/A) |
1.4 × 10−4 [1.7 × 10−4] |
13 |
102–103 |
100 °C |
1.1 × 10−4 [1.3 × 10−4] |
−6 |
102–103 |
150 °C |
3.5 × 10−5 [4.2 × 10−5] |
−10 |
102–103 |
250 °C |
1.4 × 10−4 [1.5 × 10−4] |
−8 |
102–103 |
300 °C |
3.0 × 10−4 [4.2 × 10−4] |
−8 |
102–103 |
PCZTT (N/A) |
5.1 × 10−5 [5.3 × 10−5] |
−7 |
101–102 |
150 °C |
1.8 × 10−4 [1.9 × 10−4] |
−15 |
102–103 |
250 °C |
4.8 × 10−4 [5.1 × 10−4] |
−30 |
102–103 |
300 °C |
1.4 × 10−3 [1.8 × 10−3] |
−6 |
105–106 |
Characterization of molecular organization and film morphologies
Although the TFT performances of the CZ-based polymers were somewhat correlated with their HOMO levels (PCZTT has a shallower HOMO level and higher μh than PCZT), it is necessary to investigate the organization of the thin films by GIWAXS measurements. The 2D-GIWAXS patterns provided information about the molecular packing structures (Fig. 5). The corresponding 1D profiles are shown in Fig. S7.† The pristine thin film of PCZT displayed very weak reflections (Fig. 5a and S7a†). Only after thermal annealing at 300 °C, one could observe a primary reflection peak (100) located at 2θ = 4.0° along the qxy direction (in-plane direction). In addition, a reflection peak (010) located at 2θ = 25.7° along the qz direction (out-of-plane) was found in its 1D profile (Fig. S7b†). This result indicated that PCZT tends to adopt a face-on backbone packing orientation with the chain-to-chain and π–π stacking distances of 22.0 Å and 3.5 Å, respectively (Fig. S8a†).13,25 On the other hand, the thin film of PCZTT exhibited a primary diffraction peak (100) located at 2θ = 4.9° along the qz direction (out-of-plane) with a chain-to-chain packing distance of 18.0 Å (Fig. 5c and S7c†). This result suggested that PCZTT tends to adopt an edge-on backbone packing orientation (Fig. S8b†). Furthermore, the thermal annealing treatment at 300 °C explicitly intensified the primary diffraction peak (Fig. 5d and S7d†). Therefore, the higher mobility of PCZTT was reasonably attributed to its edge-on backbone packing orientations toward the Si substrate, which was favorable for the in-plane charge transport in the TFT devices (Fig. S8b†).10,13,14,22,25 Overall, the GIWAXS results were well correlated with the TFT performances, and it was revealed that the polymers with a planar backbone show a better charge carrier transport property than those with a twist backbone.10,11,13,22,25
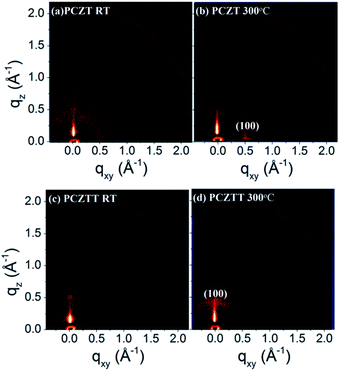 |
| Fig. 5 GIWAXS patterns of the thin films at different annealing temperatures. PCZT: (a) RT and (b) 300 °C; PCZTT: (c) RT and (d) 300 °C. | |
To elucidate the thermal annealing effects on the thin film quality, the surface morphology of the thin films was investigated using tapping-mode atomic force microscopy (AFM).13,14 As shown in Fig. 6, the thin films of PCZT showed a relatively amorphous morphology. A root-mean-square (RMS) roughness of 0.63 nm was observed for the pristine film (Fig. 6a). Thermal annealing at 150 °C increased the RMS value to 0.86 nm, whereas it decreased to 0.50 nm at the thermal annealing temperature of 300 °C (Fig. 6b and c). A smoother surface morphology would result in a better interfacial contact with the Au electrode, and accordingly, the charge carrier transport would be facilitated.14,22,25 Therefore, the thin film annealed at 150 °C showed the lowest mobility, while annealing at 300 °C led to the highest mobility in the case of PCZT. On the other hand, the RMS values of the PCZTT linearly decreased as the thermal annealing temperature increased. For instance, the pristine film showed a relatively rough surface morphology (RMS = 3.77 nm) with some small “holes” and aggregates, indicating an inferior interconnectivity and a low quality thin film (Fig. 6d). In contrast, much smoother surface morphologies (RMS = 1.52–1.64 nm) with the interconnected grains were obtained after thermal annealing at 150–300 °C (Fig. 6e and f). As a consequence, largely interconnected grains would facilitate the charge carrier transport of the PCZTT-based TFTs.14,22,25 Overall, the results were consistent with the observed changes in μh upon thermal annealing.
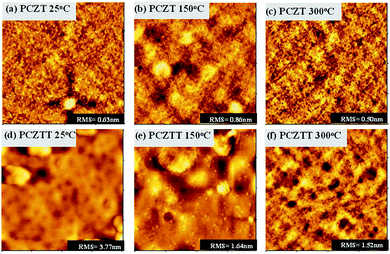 |
| Fig. 6 Tapping-mode AFM topography images of the thin films of (a to c) PCZT and (d to f) PCZTT, prepared by thermal annealing at different temperatures. Annealing temperatures (for 10 min) are depicted in each image. AFM size: 2 × 2 μm2. | |
Conclusion
In summary, a new linear-type dibromocarbazoledioxazine derivative was synthesized and fully characterized. By using this building block as a bifunctional monomer, two CZ-based semiconducting polymers, namely PCZT and PCZTT, were, for the first time, synthesized by Stille polycondensation. The LUMO levels of both polymers were almost the same due to the dominating electron-withdrawing effect of the CZ unit, while there were differences in the HOMO levels and backbone planarity. PCZTT possessed by 0.07 eV a shallower HOMO level and more planar backbone, producing a narrower band gap. The charge-transporting properties, estimated from the TFT performances, suggested that PCZTT showed a four times higher hole mobility than PCZT. In addition to the HOMO level, the enhanced hole mobility was due to the edge-on backbone packing orientations toward the Si substrate, originating from the stronger intermolecular interactions induced by the planar backbone of PCZTT. This study highlights the promising use of the CZ-based semiconductors in the field of organic electronics. It is also interesting to note that a subtle change in the spacer structure (thiophene vs. thienothiophene) dramatically alters the molecular packing orientations and TFT performances. Further improvements of the CZ-based organic semiconductors by backbone and side chain engineering are currently underway in our laboratory.
Conflicts of interest
There are no conflicts to declare.
Acknowledgements
This work was partially supported by the Heiwa Nakajima Foundation, the Ogasawara Foundation for the Promotion of Science and Engineering, the Yazaki Memorial Foundation for Science and Technology, and the Support for Tokyotech Advanced Researchers. The authors thank Mr Hiroshi Iida and Mr Masato Koizumi (Center for Advanced Materials Analysis, Tokyo Institute of Technology) for operating the GIWAXS instrument and MALDI-TOF mass spectrometer, respectively.
Notes and references
- X. Li, H. Wang, J. A. Schneider, Z. Wei, W. Lai, W. Huang, F. Wudl and Y. Zheng, J. Mater. Chem. C, 2017, 5, 2781–2785 RSC.
- Y. Cai, X. Zhang, X. Xue, D. Wei, L. Huo and Y. Sun, J. Mater. Chem. C, 2017, 5, 7777–7783 RSC.
- Y. Wang, Y. Liu, S. Chen, R. Peng and Z. Ge, Chem. Mater., 2013, 25, 3196–3204 CrossRef CAS.
- Y. Wang, F. Yang, Y. Liu, R. Peng, S. Chen and Z. Ge, Macromolecules, 2013, 46, 1368–1375 CrossRef CAS.
- Y. Wang, M. F. G. Klein, J. Hiyoshi, S. Kawauchi, W. W. H. Wong and T. Michinobu, J. Photopolym. Sci. Technol., 2015, 28, 385–391 CrossRef CAS.
- Y. Liu, Y. Wang, L. Ai, Z. Liu, X. Ouyang and Z. Ge, Dyes Pigm., 2013, 121, 363–371 CrossRef.
- W. Li, M. Otsuka, T. Kato, Y. Wang, T. Mori and T. Michinobu, Beilstein J. Org. Chem., 2016, 12, 1401–1409 CrossRef CAS PubMed.
- Q. Liu, Y. Liu, Y. Wang, L. Ai, X. Ouyang, L. Han and Z. Ge, New J. Chem., 2013, 37, 3627–3633 RSC.
- W. Wu, J. Li, Z. Zhao, X. Yang and X. Gao, Org. Chem. Front., 2017, 4, 823–827 RSC.
- Y. Wang, H. Masunaga, T. Hikima, H. Matsumoto, T. Mori and T. Michinobu, Macromolecules, 2015, 48, 4012–4023 CrossRef CAS.
- Y. Wang, M. Nakano, T. Michinobu, Y. Kiyota, T. Mori and K. Takimiya, Macromolecules, 2017, 50, 857–864 CrossRef CAS.
- Y.-Y. Lai, H.-H. Chang, Y.-Y. Lai, W.-W. Liang, C.-E. Tsai and Y.-J. Cheng, Macromolecules, 2015, 48, 6994–7006 CrossRef CAS.
- Y. Wang, T. Kadoya, L. Wang, T. Hayakawa, M. Tokita, T. Mori and T. Michinobu, J. Mater. Chem. C, 2015, 3, 1196–1207 RSC.
- H. Li, F. Liu, X. Wang, C. Gu, P. Wang and H. Fu, Macromolecules, 2013, 46, 9211–9219 CrossRef CAS.
- Y. Wang, R. Hosokawa, T. Mori and T. Michinobu, Bull. Chem. Soc. Jpn., 2017, 90, 1041–1049 CrossRef CAS.
- I. Osaka, M. Akita, T. Koganezawa and K. Takimiya, Chem. Mater., 2012, 24, 1235–1243 CrossRef CAS.
- M. Akita, I. Osaka and K. Takimiya, Materials, 2013, 6, 1061–1071 CrossRef CAS PubMed.
- K. Kawabata, M. Saito, N. Takemura, I. Osaka and K. Takimiya, Polym. J., 2017, 49, 169–176 CrossRef CAS.
- M. S. White, M. Kaltenbrunner, E. D. Głowacki, K. Gutnichenko, G. Kettlgruber, I. Graz, S. Aazou, C. Ulbricht, D. A. M. Egbe, M. C. Miron, Z. Major, M. C. Scharber, T. Sekitani, T. Someya, S. Bauer and N. S. Sariciftci, Nat. Photonics, 2013, 7, 812–816 CrossRef.
- P. Heremans, A. K. Tripathi, A. de Jamblinne de Meux, E. C. P. Smits, B. Hou, G. Pourtois and G. H. Gelinck, Adv. Mater., 2016, 28, 4266–4282 CrossRef CAS PubMed.
- Y. Wang and T. Michinobu, Bull. Chem. Soc. Jpn., 2017, 90, 1388–1400 CrossRef CAS.
- Y. Wang, T. Hasegawa, H. Matsumoto, T. Mori and T. Michinobu, Adv. Funct. Mater., 2017, 27, 1604608 CrossRef.
- G. Kim, S.-J. Kang, G. K. Dutta, Y.-K. Han, T. J. Shin, Y.-Y. Noh and C. A. Yang, J. Am. Chem. Soc., 2014, 136, 9477–9483 CrossRef CAS PubMed.
- H. Chen, Y. Guo, G. Yu, Y. Zhao, J. Zhang, D. Gao, H. Liu and Y. Liu, Adv. Mater., 2012, 24, 4618–4622 CrossRef CAS PubMed.
- Y. Wang, A. T. Tan, T. Mori and T. Michinobu, J. Mater. Chem. C, 2018 10.1039/C7TC04993E.
- Y. Wang and T. Michinobu, J. Mater. Chem. C, 2016, 4, 6200–6214 RSC.
- F. S. Melkonyan, W. Zhao, M. Drees, N. D. Eastham, M. J. Leonardi, M. R. Butler, Z. Chen, X. Yu, R. P. H. Chang and M. A. Ratner, J. Am. Chem. Soc., 2016, 138, 6944–6947 CrossRef CAS PubMed.
- J.-H. Kim, S. A. Shin, J. B. Park, C. E. Song, W. S. Shin, H. Yang, Y. Li and D.-H. Hwang, Macromolecules, 2014, 47, 1613–1622 CrossRef CAS.
- I. Osaka, Y. Houchin, M. Yamashita, T. Kakara, N. Takemura, T. Koganezawa and K. Takimiya, Macromolecules, 2014, 47, 3502–3510 CrossRef CAS.
- H. S. Kim, G. Huseynova, Y.-Y. Noh and D.-H. Hwang, Macromolecules, 2017, 50, 7550–7558 CrossRef CAS.
- C. Wang, H. Dong, W. Hu, Y. Liu and D. Zhu, Chem. Rev., 2011, 112, 2208–2267 CrossRef PubMed.
- Y. Zhao, Y. Guo and Y. Liu, Adv. Mater., 2013, 25, 5372–5391 CrossRef CAS PubMed.
- W. Li, K. H. Hendriks, M. M. Wienk and R. A. J. Janssen, Acc. Chem. Res., 2016, 49, 78–85 CrossRef CAS PubMed.
- T. Lei, J.-Y. Wang and J. Pei, Acc. Chem. Res., 2014, 47, 1117–1126 CrossRef CAS PubMed.
- W. Jiang, Y. Li and Z. Wang, Acc. Chem. Res., 2014, 47, 3135–3147 CrossRef CAS PubMed.
- N. Liang, K. Sun, Z. Zheng, H. Yao, G. Gao, X. Meng, Z. Wang, W. Ma and J. Hou, Adv. Energy Mater., 2016, 6, 1600060 CrossRef.
- Z. Wu, C. Sun, S. Dong, X.-F. Jiang, S. Wu, H. Wu, H.-L. Yip, F. Huang and Y. Cao, J. Am. Chem. Soc., 2016, 138, 2004–2013 CrossRef CAS PubMed.
- M. Gsänger, D. Bialas, L. Huang, M. Stolte and F. Würthner, Adv. Mater., 2016, 28, 3615–3645 CrossRef PubMed.
- N. C. Debnath and S. A. Vaidya, Prog. Org. Coat., 2006, 56, 159–168 CrossRef CAS.
- P. T. C. Lee, C.-W. Chiu, T.-M. Lee, T.-Y. Chang, M.-T. Wu, W.-Y. Cheng, S.-W. Kuo and J.-J. Lin, ACS Appl. Mater. Interfaces, 2013, 5, 5914–5920 CAS.
- P. T. C. Lee, C.-W. Chiu, L.-Y. Chang, P.-Y. Chou, T.-M. Lee, T.-Y. Chang, M.-T. Wu, W.-Y. Cheng, S.-W. Kuo and J.-J. Lin, ACS Appl. Mater. Interfaces, 2014, 6, 14345–14352 CAS.
- M. Buccella, A. Dorigato, F. Crugnola, M. Caldara and L. Fambri, J. Appl. Polym. Sci., 2015, 132, 41452 CrossRef.
- W. Zhang, J. Wang, J. Mao, L. Hu, X. Wu and C. Guo, Tetrahedron Lett., 2016, 57, 1985–1989 CrossRef CAS.
- A. Kimoto and Y. Tajima, Org. Lett., 2012, 14, 2282–2285 CrossRef CAS PubMed.
- M. Ikeda, K. Kitahara and H. Nishi, J. Heterocycl. Chem., 1990, 27, 1575–1579 CrossRef CAS.
- M. Ikeda, K. Kitahara and H. Nishi, J. Heterocycl. Chem., 1991, 28, 1165–1171 CrossRef CAS.
- M. Ikeda, K. Kitahara, H. Nishi, H. Nakahara and K. Fukuda, Chem. Lett., 1991, 20, 1177–1180 CrossRef.
- M. Ikeda, K. Kitahara and H. Nishi, J. Heterocycl. Chem., 1992, 29, 289–294 CrossRef CAS.
- H. Tatsumi, Y. Wang, Y. Aizawa, M. Tokita, T. Mori and T. Michinobu, J. Phys. Chem. C, 2016, 120, 26686–26694 CAS.
- N. Blouin and M. Leclerc, Acc. Chem. Res., 2008, 41, 1110–1119 CrossRef CAS PubMed.
- S. Wakim, S. Beaupré, N. Blouin, B. Aich, S. Rodman, R. Gaudiana, Y. Tao and M. Leclerc, J. Mater. Chem., 2009, 19, 5351–5358 RSC.
- S. H. Park, A. Roy, S. Beaupré, S. Cho, N. Coates, J. S. Moon, D. Moses, M. Leclerc, K. Lee and A. J. Heeger, Nat. Photonics, 2009, 3, 297–302 CrossRef CAS.
- S. Beaupré and M. Leclerc, J. Mater. Chem. A, 2013, 1, 11097–11105 Search PubMed.
- N. Blouin, M. Leclerc, B. Vercelli, S. Zecchin and G. Zotti, Macromol. Chem. Phys., 2006, 207, 175–182 CrossRef CAS.
- N. Blouin, A. Michaud, S. Wakim, P.-L. T. Boudreault, M. Leclerc, B. Vercelli, S. Zecchin and G. Zotti, Macromol. Chem. Phys., 2006, 207, 166–174 CrossRef CAS.
- J. Lu, F. Liang, N. Drolet, J. Ding, Y. Tao and R. Movileanua, Chem. Commun., 2008, 5315–5317 RSC.
- S. Cai, L. Chen, D. Zha and Y. A. Chen, J. Polym. Sci., Part A: Polym. Chem., 2013, 51, 624–634 CrossRef CAS.
- S. Habuchi, H. Fujita, T. Michinobu and M. Vacha, J. Phys. Chem. B, 2011, 115, 14404–14415 CrossRef CAS PubMed.
- H. Piwoński, T. Michinobu and S. Habuchi, Nat. Commun., 2017, 8, 15256 CrossRef PubMed.
- J. W. Choi, C. Kulshreshtha, G. P. Kennedy, J. H. Kwon, S. Jung and M. Chae, Sol. Energy Mater. Sol. Cells, 2011, 95, 2069–2076 CrossRef CAS.
- Y. Wang, T. Hasegawa, H. Matsumoto, T. Mori and T. Michinobu, Adv. Mater., 2018 DOI:10.1002/adma.201707164.
- N. S. Baek, S. K. Hau, H.-L. Yip, O. Acton, K.-S. Chen and A. K.-Y. Jen, Chem. Mater., 2008, 20, 5734–5736 CrossRef CAS.
- Y. Wang, T. Hasegawa, H. Matsumoto, T. Mori and T. Michinobu, Adv. Funct. Mater., 2017, 27, 1701486 CrossRef.
Footnote |
† Electronic supplementary information (ESI) available: 1H NMR spectra of the monomer and oligomers, TGA and DSC curves, current–voltage (I–V) characteristics of TFT devices. See DOI: 10.1039/c8ra01088a |
|
This journal is © The Royal Society of Chemistry 2018 |
Click here to see how this site uses Cookies. View our privacy policy here.