DOI:
10.1039/C8RA03604G
(Paper)
RSC Adv., 2018,
8, 18784-18791
The physicochemical properties of a room-temperature liquidus binary ionic liquid mixture of [HNMP][CH3SO3]/[Bmim]Cl and its application for fructose conversion to 5-hydroxymethylfurfural
Received
26th April 2018
, Accepted 17th May 2018
First published on 23rd May 2018
Abstract
Via heating first and then cooling, binary ionic liquid (IL) mixture of N-methyl-2-pyrrolidonium methylsulfonate ([HNMP][CH3SO3]) and 1-butyl-3-methylimidazolium chloride ([Bmim]Cl) could form a liquid at room temperature. The glass-transition temperature (Tg) characterized by DSC depends on its composition with Tg being as low as −63 °C. The physicochemical properties of the binary IL mixtures also vary with the composition. With the increase of the mole fraction of [Bmim]Cl, the hydrogen-bond accepting ability (β) of the binary IL mixture increases, but the hydrogen-bond donating ability (α) deceases. In this binary IL mixture, fructose could be effectively converted into 5-hydroxymethylfurfural (HMF) at room temperature. The HMF yields at a given time are found to be well correlated with the physicochemical properties of the binary mixture, especially the α and β values. Under specified conditions, the present IL mixture as medium for fructose dehydration into HMF is comparable to the medium formed by ILs and alcohol, where the alcohols have negative effect on the HMF formation.
Introduction
Ionic liquids (ILs) are organic salts with melting point below 100 °C. Compared with traditional molecular solvents, ILs can be regarded as green solvents due to their low vapor pressure, good thermal stability and large liquidus temperature range. Also the physicochemical properties of ILs could be fine-tuned by altering the component ions or modifying the substituent group on the ions, and thus, ILs are also considered as designer solvents. As media, ILs could dissolve many inorganic compounds, organic compounds and polymers; e.g., some imidazolium-based ILs containing Cl− and OAc− anions are proved to be good solvents for sugar.1,2 In addition, some ILs have been demonstrated to be catalytically active for some chemical reactions via specific solvent–solute interactions.3–5
The physicochemical property of a two-ion IL could also be tuned via its mixing with molecular solvent or another two-ion IL.6–10 The so-called ‘Double Salt Ionic Liquids’ (DSILs) are defined as salts composed of more than two types of ions.6 Usually DSILs are obtained by mixing two simple ILs. Insight into the relationship between the composition of DSIL and its physicochemical property has been presented for several binary IL mixtures.11–13 As far as the melting point is concerned, the variation of the abundance of each ion could result in an IL mixture with the melting point or glass-transition temperature being much lower than its component IL.14 For the viscosity of a DSIL, a linear or non-linear change of viscosity with the composition could be also observed.15,16 The changes mentioned-above are usually accompanied by a composition-dependent variation of the hydrogen-bond accepting and donating abilities, which will eventually affect its interaction with the dissolved solute.17,18 In general, by changing the type of ion and/or the ratio of the ions, one could obtain a binary IL mixture with unique properties (e.g., melting point, viscosity, polarity, hydrogen-bond accepting and donating abilities, etc.) and tune the chemical reactions in it.
5-Hydroxymethylfurfural (HMF) is an important platform compound from which various chemicals could be derived. The use of sugar as feedstock for the preparation of HMF is one of the hotspots in the field of biomass conversion.19,20 In recent years, ILs have been tried as catalysts or media for the production of HMF.21–23 As a sugar-dissolving IL and a proton functionalized IL, [Bmim]Cl and [HNMP][CH3SO3] are frequently used for HMF production. However, both ILs are solid at room temperature, so the dehydration reaction has to be carried out at higher temperature. Higher reaction temperature usually results in byproducts (e.g., organic acids and humin) and also high energy consumption.24 For the room-temperature conversion of fructose to HMF, some organic molecular solvents are used to formulate deep eutectic solvents (DES) with IL to lower the reaction temperature.25,26 Our previous study has shown that the DES containing alcohol as hydrogen-bond donor has a negative effect on the HMF formation. The higher the polarity of the alcohol, the greater the negative effect.25,26 So, it is of great significance to formulate a room temperature liquidus binary IL mixture, which is capable of dissolving sugar and catalyzing sugar dehydration. In the present study, the thermal behavior of the mixture of [Bmim]Cl and [HNMP][CH3SO3] was investigated. It is found interestingly that a liquidus binary mixture of [Bmim]Cl/[HNMP][CH3SO3] could be obtained at room temperature and in this medium fructose can be efficiently converted into HMF. The HMF yields at a given time in binary mixtures of different mole fractions are found to be well correlated with the physicochemical properties of the media.
Experiment
Materials
N-methyl-2-pyrrolidonium methylsulfonate ([HNMP][CH3SO3], 99%) and 1-butyl-3-methylimidazolium chloride ([Bmim]Cl, 99%) were purchased from Shanghai Chengjie Chemicals Co., Ltd. N,N-Diethyl-4-nitroaniline (DENA, 97%) and acetonitrile (HPLC grade) were purchased from J&K Chemical Co., Ltd. 4-Nitroaniline (NA, 98%) was purchased from Aladdin Industrial Co., Ltd. 1-Ethyl-4-(methoxycarbonyl)pyridinium iodide (EMCPI, 97%) was purchased from TCI Co., Ltd. Fructose (98%), DMSO (analytical grade) and methanol (analytical grade) were purchased from Sinopharm Chemical Reagent Co., Ltd. Ultrapure water (18.25 MΩ cm) was used throughout the experiments.
DSC traces of binary ionic liquid mixtures
A mixture of [HNMP][CH3SO3] and [Bmim]Cl hosted in an airtight glass vial was kept at 80 °C and stirred until it is molten. The DSC trace of the binary mixture was recorded under the nitrogen atmosphere on a PerkinElmer DSC8500 differential scanning calorimeter. In a typical measurement, the sample (8.0 mg) was first heated to 80 °C and then kept at 80 °C for 5 min. After that, the sample was cooled to −70 °C at a rate of 4 °C min−1 and held at −70 °C for 5 min. Finally, the sample were reheated to 80 °C at a rate of 4 °C min−1.
Typical procedure for fructose conversion into HMF
All fructose dehydration reactions were carried out in a 5 mL airtight glass vial. First, 3.30 mmol [HNMP][CH3SO3] was mixed with a given amount of [Bmim]Cl and the mixture was then heated and stirred until it is molten. The clear mixture was subsequently cooled down to room temperature. After that, 1.65 mmol fructose was added and the resulting system was stirred at 600 rpm for a certain period of time at 25 °C. Finally, the resulting mixture was diluted with ultrapure water and then analyzed by HPLC. All HMF yields reported in this study were an average of triplicate experiments.
HPLC analysis
The separation and the detection of HMF were carried out on an HPLC apparatus equipped with a Shim-pack VP-ODS C18 column (250 mm × 4.6 mm), a Shimadzu LC-20AT pump and a Shimadzu SPD-20A detector at 283 nm. The mobile phase was a mixture of acetonitrile and water (15/85, v/v) and the flow rate was maintained at 0.8 mL min−1. Under the present HPLC conditions, only HMF has a response to the detector with the retention time of 6.679 min. The actual concentration of HMF was obtained directly from the calibration curve (correlation coefficient is 0.999).
In situ 1H NMR analysis
The fructose dehydration at 25 °C was monitored on a Bruker Avance 300 MHz spectrometer. First, 0.35 mmol fructose was dissolved in 12.6 mmol methanol or DMSO. Then a binary IL mixture formed by 0.70 mmol [HNMP][CH3SO3] and 4.2 mmol [Bmim]Cl was added and mixed. The resulting mixture was transferred into a 5 mm (i.d.) NMR tube (a capillary filled with D2O was put into the tube as external standard). Finally, the NMR tube was subsequently inserted into the chamber of the spectrometer, followed by recording the 1H NMR spectra in fixed time intervals.
Viscosity measurement
The viscosity of the binary IL mixtures was measured on a Thermo Scientific HAAKE RheoStress 6000 rheometer at 25 °C. Prior to use, the rheometer was calibrated. All data given in the report were an average of triplicate measurements.
Determination of solvatochromic parameters
Solvatochromic parameters of the probes (NA, DENA and EMCPI) in binary IL mixtures were determined based on the wavelength corresponding to the absorption maximum (λmax) of each probe, which was measured on a UV-2550 UV-Vis spectrophotometer (Shimadzu Co., Suzhou, China). The stock solutions (1 × 10−3 M) of the probes were all made in methanol. The samples for UV-vis measurement were prepared as follows: First, an aliquot of a probe solution (40 μL) was pipetted into a vial; after methanol evaporation, an aliquot of a binary IL mixture (800 μL) was added and thoroughly mixed for 24 h to form a final solution in the binary IL mixture. The final concentration of each probe in the solutions was 5 × 10−5 M.
Results and discussion
Thermal behavior of the binary IL mixtures
[HNMP][CH3SO3] and [Bmim]Cl (their structures are shown in Scheme 1), commonly used respectively as catalyst and solvent for HMF production, are both solid at ambient temperature, however, a room-temperature liquidus binary IL mixture could be obtained by first heating the solid IL mixture to 80 °C and then cooling down to the room temperature. The resulting liquid did not crystallize in a week, indicating the much slower crystallization process. This supercooling phenomenon could be explored for room-temperature HMF production.
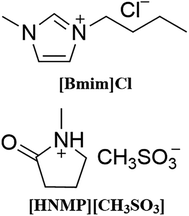 |
| Scheme 1 Structures of [Bmim]Cl and [HNMP][CH3SO3]. | |
For further study, DSC curves of the neat ILs and their mixtures were recorded (see Fig. 1). For neat [HNMP][CH3SO3], an exothermal peak at ca. −8 °C and an endothermal peak at ca. 52 °C were observed during the cooling and reheating, which correspond to the crystallization and the melting of [HNMP][CH3SO3], respectively. The discrepancy between the melting and the freezing temperatures is due to supercooling.27 As for neat [Bmim]Cl, it melts at 68 °C, but after heated, the molten [Bmim]Cl has no crystallization peak and only glass transition phenomenon (at ca. −39 °C and −48 °C) was observed in the course of cooling and reheating at the rate of 4 °C min−1 (as reported in literature they are at −41 °C and −48 °C).28 This phenomenon indicates that the crystallization of [Bmim]Cl is kinetically much slower than that of [HNMP][CH3SO3].29,30 For their mixture, a thermal behaviour similar to the neat [Bmim]Cl was also observed, but the glass-transition temperature (Tg) was shifted to lower temperature (ca. −63 °C). The present thermal behaviours of the binary IL mixture are very similar to those reported elsewhere.27,31 It is noteworthy that with the increase of [Bmim]Cl, the Tg of IL mixture rose from −63 °C to −52 °C. This composition-dependent phenomenon could be rationalized as follows: on the one hand, more Cl− anions, which have smaller size and higher charge density, result in an enhancement of the coulombic interaction between ions; on the other hand, the hydrogen-bond donating ability of [HNMP]+ is stronger than that of [Bmim]+, so the decrease of [HNMP]+ content results in weaker hydrogen bonds and therefore more ordered ionic structure, thereby enhancing ion–ion interaction.32 However, the reason why the Tg values of the binary mixtures in [HNMP][CH3SO3]-rich region change little is not clear and it need further investigation.
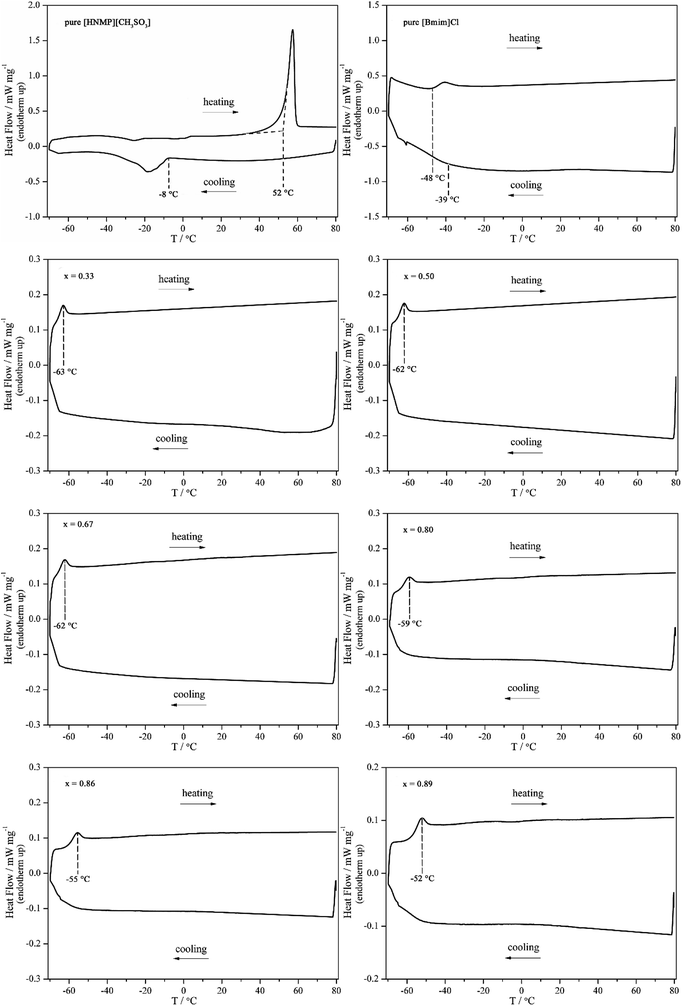 |
| Fig. 1 DSC thermograms of pure ILs and their mixtures. ax represents the mole fraction of [Bmim]Cl. bFor pure ILs, the onset temperature is considered as the melting or freezing temperature; for mixture, the temperature at the peak position was considered as Tg. | |
Physicochemical properties of the binary IL mixtures
The polarity (P), hydrogen-bond donating ability (α) and hydrogen-bond accepting ability (β) of the binary IL mixtures at 25 °C were determined using solvatochromic dyes as probes. The P parameter is calculated based on eqn (1), where λmax,EMCPI is the wavelength corresponding to the absorption maximum of EMCPI in the binary IL mixtures (see Table 1). The α and β parameters are calculated based on eqn (2)–(4), where νmax,DENA and νmax,NA are the wavenumbers converted based on the absorption maxima of DENA and NA in binary IL mixtures (see Table 1).33,34 |
π* = 0.314 × (27.52 − νmax,DENA)
| (2) |
|
α = 0.0485P − 2.75 − 0.46π*
| (3) |
|
β = 0.358 × (31.10 − νmax,NA) − 1.125π*
| (4) |
Table 1 The wavelength corresponding to the absorption maximum of a probe in the binary IL mixtures at 25 °Ca
Pure ILs are solid at RT, therefore, the probe solutions in them were prepared at 80 °C.35 The wavelength corresponding to absorption maximum of the probe in each solution was determined via the first-order derivative transformation. |
χ[Bmim]Cl |
0.00 |
0.33 |
0.50 |
0.67 |
0.80 |
0.86 |
0.89 |
1.00 |
λmax,EMCPIb/nm |
345 |
348 |
352 |
354 |
362 |
365 |
370 |
375 |
λmax,DENAb/nm |
411 |
415 |
413 |
414 |
415 |
416 |
417 |
416 |
λmax,NAb/nm |
375 |
388 |
390 |
393 |
397 |
398 |
399 |
398 |
The derived solvatochromic parameters (π*, α and β) as well as the viscosity (η) of the binary IL mixtures are shown in Fig. 2. It is seen that, with the increase of the mole fraction of [Bmim]Cl (x), the absorption maximum of EMCPI moves towards longer wavelength, which indicates the polarity of the binary IL mixtures decreases (the ground state of EMCPI is more polar than its excited state). As for α and β values, Fig. 2A shows that they are both proportional to the x value; with the increase of [Bmim]Cl content, β increases from 0.46 to 0.91, but α decreases from 0.81 to 0.44. This is because in the present binary IL mixtures, Cl− is a better hydrogen-bond acceptor than [CH3SO3]−, while [HNMP]+ is a better hydrogen-bond donor than [Bmim]+.6,11 For the viscosity of the binary mixtures, Fig. 2B shows that it rises nonlinearly as a function of the x value. This trend could be explained using a similar rationale to that for the composition-dependent Tg, i.e., the ions interaction is the major factor, the larger the force, the larger the viscosity.6,32
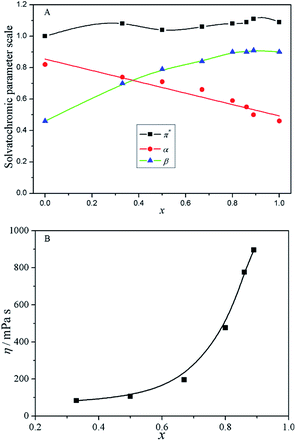 |
| Fig. 2 Variation of solvatochromic parameters (A) and viscosity (B) with mole fraction of [Bmim]Cl in the mixture. | |
Fructose conversion into HMF in binary IL mixtures
Fig. 3 shows the time-dependent HMF yield in IL mixtures of different mole fractions under the reaction conditions of 25 °C and 600 rpm. For a given amount of fructose, the HMF yield in a given time interval increases with the increase of x. Specifically, the HMF yield in 1.0 h varies from the lowest 24.6% to the highest 73.0%, which indicates that the composition of the binary IL mixtures affects the formation rate. Based on the physicochemical properties of the binary mixture (characterized in the previous section), we speculate that the change may be related to the viscosity and/or the hydrogen-bond donating/accepting ability of the medium.
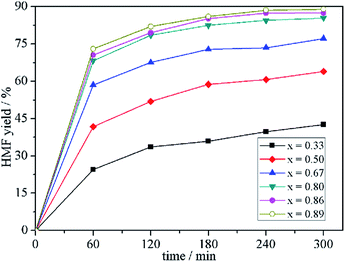 |
| Fig. 3 Kinetic curves of fructose dehydration into HMF in binary IL mixtures of different mole fraction. | |
To explore the effect of the viscosity on the formation rate, a less viscous IL mixture (x = 0.33) and a more viscous IL mixture (x = 0.86) were selected as media for fructose dehydration, and the HMF yield at a given time in the two media under different stirring speeds were compared (see Fig. 4). As shown in Fig. 4, the formation rate of HMF under unstirred condition is low in both media. The HMF yields in 1.0 h and 5.0 h were 10.2% and 23.7% (x = 0.33) and 13.0% and 19.9% (x = 0.86), respectively. Relatively speaking, the increment in HMF yield in the less viscous mixture is larger than that in the more viscous mixture. This result indicates that the more viscous the binary IL mixture is, the greater the mass transfer resistance and the slower the HMF formation rate. To eliminate the mass transfer resistance, the reaction system was subjected to agitation. Under the conditions of 600 rpm and 1200 rpm, HMF yields after 5.0 h were 45.4% and 48.9% (x = 0.33) and 87.4% and 88.0% (x = 0.86), respectively. Compared with that under 600 rpm, further increase of stirring speed could not enhance the HMF formation rate significantly. It follows that without stirring, the viscosity of the media has significant negative effect on the fructose dehydration due to the mass transfer resistance. When the mass transfer resistance was overcome by stirring, the viscosity is no longer the key factor affecting the formation rate of HMF.
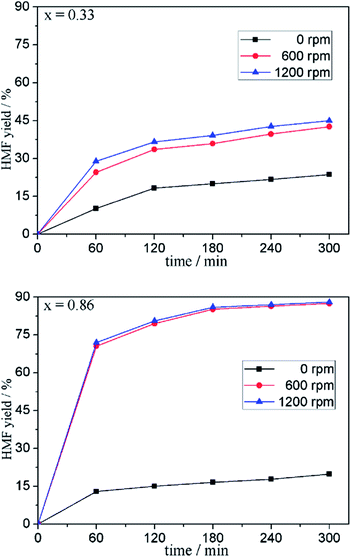 |
| Fig. 4 Effect of the stirring on the formation rate of HMF in two different binary IL mixtures. Reaction condition: First, 1.65 mmol fructose was dissolved into the binary IL mixtures, then the resulting mixtures were kept stirring at 0 rpm, 600 rpm or 1200 rpm for 5.0 h at 25 °C. | |
It is known that the interaction between ILs and reactant/intermediates/products have effects on chemical reactions in the ILs as media.17,36–39 Here, an attempt was made to correlate the formation rate of HMF with the hydrogen-bond donating/accepting ability of the binary IL mixtures. To prevent the interference from the preferential solvation in the [Bmim]Cl-rich medium and from the negative effect of the high viscosity on the mass transfer,17 only data over x = 0.33–0.80 are used for discussion. In this region, it is found that the HMF yields at a given time (t = 300 min) in different media are well correlated with the α and β values (Fig. 5). This indicates that the change of x not only leads to a change of its physicochemical properties, but also affects the formation rate of the involved dehydration reaction.
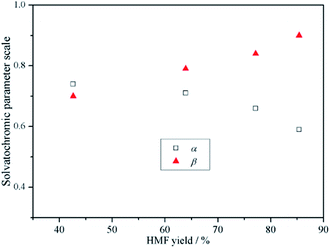 |
| Fig. 5 A plot of α (β) versus HMF yield at a given time (t = 300 min) in different binary IL mixtures. | |
The dehydration of fructose into HMF goes through the following basic route (see Scheme 2). Our previous study has shown that [HNMP][CH3SO3] is helpful for the conversion of fructose into intermediates whether or not [Bmim]Cl is present; the presence of [Bmim]Cl, however can enhance the transformation of intermediates due to the formation of hydrogen bond between the Cl− and the hydroxyl involved in the transformation, which promotes the transfer of proton on [HNMP]+ to the leaving hydroxyl (see Scheme 3).25,26 Since the amount of [HNMP][CH3SO3] as proton donor is much more in the present study than that of fructose, the medium effect on the HMF formation rate should be proportional to the content of [Bmim]Cl, i.e. the β values.
 |
| Scheme 2 Route of fructose dehydration into HMF. | |
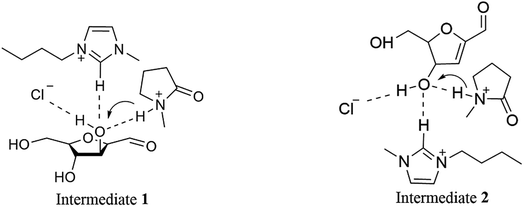 |
| Scheme 3 Proposed mechanism for the concerted activation of the intermediates by [HNMP][CH3SO3] and [Bmim]Cl. | |
Comparison with IL/Alcohol medium
[Bmim]Cl could interact with short-chain alcohols to form DESs, which are liquids at room temperature. The use of these DESs as media for fructose dehydration into HMF has been reported.26 It has been found that the alcohol as HBD will compete with both fructose and intermediates to form hydrogen bonds with ILs, leading to a negative effect on fructose conversion or intermediates transformation. To compare with DESs formulated by IL/alcohol, the alcohol effect on fructose dehydration in the present binary IL mixture was investigated. As shown in Fig. 6, the HMF yield at a given time in the binary mixture with methanol was much lower than that with DMSO (the latter was very close to that without any additive) (note: there is a dilution effect in the presence of additives). This result shows the presence of alcohol has a negative effect on HMF formation rate.
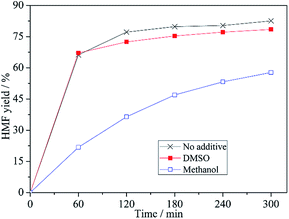 |
| Fig. 6 Effect of additives on the fructose dehydration into HMF in the IL mixture (x = 0.86). Reaction condition: 0.35 mmol fructose was added into a binary mixture composed of 0.7 mmol [HNMP][CH3SO3] and 4.2 mmol [Bmim]Cl, followed by adding 12.6 mmol methanol or DMSO. The resulting mixtures were stirred at 600 rpm for 5 h (25 °C). | |
To investigate the effect of additives, in situ 1H NMR was used to monitor the fructose dehydration process (see Fig. 7). In the medium with methanol, the height of signals of intermediates 1 and 2 (Scheme 2, marked as I and II, respectively) increases gradually with time and moreover, they are much higher at a given time than those of signals of HMF (labelled with ●), indicating that the transformation of intermediates 1 and 2 are very slow. In the medium with DMSO, the formation and transformation of intermediates 1 and 2 are very fast due to the absence of the negative effect of methanol, so the signal of intermediate 1 (peak I) was not detected and the signal of intermediate 2 (peak II) was observed only at the very beginning of the reaction (within 5 min); moreover, the signals of HMF increased with the increase of reaction time. The above result demonstrates that the binary mixture composed of [HNMP][CH3SO3] and [Bmim]Cl could effectively catalyse fructose conversion into HMF, and the presence of methanol has a marked negative effect. Based on our previous research, we speculate that the negative effect is exerted mainly via the hydrogen bond formed between methanol and the ILs. On the one hand, the hydroxyl on methanol can compete with that of fructose to form a six-membered ring with [HNMP]+, weakening the interaction between [HNMP]+ and fructose or intermediates. On the other hand, methanol can also act as HBD to form hydrogen bond directly with Cl− or [HNMP]+, destabilizing the transition state and resulting in an accumulation of intermediates 1 and 2. DMSO as a hydrogen-bond acceptor can neither form hydrogen bond with Cl− nor a six-membered ring with [HNMP]+, thus, the addition of DMSO has little impact on the fructose dehydration into HMF.
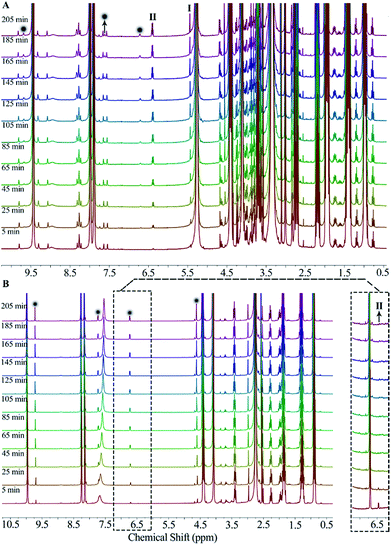 |
| Fig. 7 1H NMR spectra collected during fructose dehydration in the IL mixture (x = 0.86) with (A) methanol or (B) DMSO. | |
Conclusions
For the first time, a room temperature liquidus binary IL mixture of [HNMP][CH3SO3]/[Bmim]Cl was prepared, the composition-dependent physicochemical properties were characterized, the binary mixture as medium for the room-temperature fructose dehydration into HMF was tried, and a correlation between the formation rate of HMF and the physicochemical parameters were made. It was shown that a liquidus binary IL mixture could be formed at room temperature when the ILs were subjected to heating and then cooling down to room temperature. No precipitation was observed in a week at ambient temperature. The P, α and β values of the binary IL mixtures vary in a regular way with the change of the mole fraction of the two ILs. The viscosity of the binary mixtures has an impact on the fructose dehydration but it is not the key factor when an agitation was exerted. The HMF yields at a given time in different binary IL mixtures are well correlated with the physicochemical properties (especially α and β). Under specified conditions, the present IL mixture as medium for fructose dehydration into HMF is comparable to the medium formed by ILs and alcohol, where the alcohols have negative effect on the HMF formation.
Conflicts of interest
There are no conflicts to declare.
Acknowledgements
We are grateful for the financial support from the Fundamental Research Funds of Shandong University (2015CJ005), the Provincial Key R & D Project of Shandong (2015GSF121035) and the National Natural Science Foundation of China (21773143).
Notes and references
- P. Moyer, M. D. Smith, N. Abdoulmoumine, S. C. Chmely, J. C. Smith, L. Petridis and N. Labbe, Phys. Chem. Chem. Phys., 2018, 20, 2508–2516 RSC.
- R. P. Swatloski, S. K. Spear, J. D. Holbrey and R. D. Rogers, J. Am. Chem. Soc., 2002, 124, 4974–4975 CrossRef PubMed.
- S. T. Keaveney, R. S. Haines and J. B. Harper, Org. Biomol. Chem., 2015, 13, 3771–3780 Search PubMed.
- M. Gazitua, R. A. Tapia, R. Contreras and P. R. Campodonico, New J. Chem., 2014, 38, 2611–2618 RSC.
- X. Kang, X. Sun and B. Han, Adv. Mater., 2016, 28, 1011–1030 CrossRef PubMed.
- G. Chatel, J. F. B. Pereira, V. Debbeti, H. Wang and R. D. Rogers, Green Chem., 2014, 16, 2051–2083 RSC.
- H. Niedermeyer, J. P. Hallett, I. J. Villar-Garcia, P. A. Hunt and T. Welton, Chem. Soc. Rev., 2012, 41, 7780–7802 RSC.
- J. Zhu, K. Yu, Y. Zhu, R. Zhu, F. Ye, N. Song and Y. Xu, J. Mol. Liq., 2017, 232, 182–187 CrossRef.
- Y. Xu, J. Chem. Thermodyn., 2013, 64, 126–133 CrossRef.
- Y. Xu, H. Zhu and L. Yang, J. Chem. Eng. Data, 2013, 58, 2260–2266 CrossRef.
- P. Bharmoria, K. Damarla, T. J. Trivedi, N. I. Malek and A. Kumar, RSC Adv., 2015, 5, 99245–99252 RSC.
- Y. Huang, X. Zhang, Y. Zhao, S. Zeng, H. Dong and S. Zhang, Phys. Chem. Chem. Phys., 2015, 17, 26918–26929 RSC.
- F. D'Anna, S. Marullo, P. Vitale and R. Noto, ChemPhysChem, 2012, 13, 1877–1884 CrossRef PubMed.
- G. J. Maximo, R. J. B. N. Santos, P. Brandao, J. M. S. S. Esperanca, M. C. Costa, A. J. A. Meirelles, M. G. Freire and J. A. P. Coutinho, Cryst. Growth Des., 2014, 14, 4270–4277 Search PubMed.
- D. Y. Song and J. Chen, J. Chem. Eng. Data, 2014, 59, 257–262 CrossRef.
- N. Zhao, J. Jacquemin, R. Oozeerally and V. Degirmenci, J. Chem. Eng. Data, 2016, 61, 2160–2169 CrossRef.
- E. Priede, S. Brica, E. Bakis, N. Udris and A. Zicmanis, New J. Chem., 2015, 39, 9132–9142 RSC.
- S. Marullo, F. D'Anna, P. R. Campodonico and R. Noto, RSC Adv., 2016, 6, 90165–90171 RSC.
- I. K. M. Yu and D. C. W. Tsang, Bioresour. Technol., 2017, 238, 716–732 CrossRef PubMed.
- P. Zhou and Z. Zhang, Catal. Sci. Technol., 2016, 6, 3694–3712 Search PubMed.
- Y. Ma, S. Qing, L. Wang, N. Islam, S. Guan, Z. Gao, X. Mamat, H. Li, W. Eli and T. Wang, RSC Adv., 2015, 5, 47377–47383 RSC.
- C. Shi, Y. Zhao, J. Xin, J. Wang, X. Lu, X. Zhang and S. Zhang, Chem. Commun., 2012, 48, 4103–4105 RSC.
- F. D'Anna, S. Marullo, P. Vitale, C. Rizzo, P. Lo Meo and R. Noto, Appl. Catal., A, 2014, 482, 287–293 CrossRef.
- T. Wang, M. W. Nolte and B. H. Shanks, Green Chem., 2014, 16, 548–572 RSC.
- J. Zhang, X. Yu, F. Zou, Y. Zhong, N. Du and X. Huang, ACS Sustainable Chem. Eng., 2015, 3, 3338–3345 CrossRef.
- J. Zhang, Y. Xiao, Y. Zhong, N. Du and X. Huang, ACS Sustainable Chem. Eng., 2016, 4, 3995–4002 CrossRef.
- G. Annat, M. Forsyth and D. R. MacFarlane, J. Phys. Chem. B, 2012, 116, 8251–8258 CrossRef PubMed.
- M. Kick, K. Philipp and A. König, Fluid Phase Equilib., 2013, 338, 172–178 CrossRef.
- O. Yamamuro, Y. Minamimoto, Y. Inamura, S. Hayashi and H. O. Hamaguchi, Chem. Phys. Lett., 2006, 423, 371–375 CrossRef.
- K. Nishikawa, S. Wang, H. Katayanagi, S. Hayashi, H.-o. Hamaguchi, Y. Koga and K.-i. Tozaki, J. Phys. Chem. B, 2007, 111, 4894–4900 CrossRef PubMed.
- M. Kunze, S. Jeong, E. Paillard, M. Winter and S. Passerini, J. Phys. Chem. C, 2010, 114, 12364–12369 Search PubMed.
- K. Fumino, S. Reimann and R. Ludwig, Phys. Chem. Chem. Phys., 2014, 16, 21903–21929 RSC.
- A. Duereh, Y. Sato, R. L. Smith and H. Inomata, J. Phys. Chem. B, 2016, 120, 4467–4481 CrossRef PubMed.
- Y. Marcus, Chem. Soc. Rev., 1993, 22, 409–416 RSC.
- Y. Fukaya, A. Sugimoto and H. Ohno, Biomacromolecules, 2006, 7, 3295–3297 CrossRef PubMed.
- S. H. Mushrif, S. Caratzoulas and D. G. Vlachos, Phys. Chem. Chem. Phys., 2012, 14, 2637–2644 RSC.
- C. C. Weber, A. F. Masters and T. Maschmeyer, J. Phys. Chem. B, 2012, 116, 1858–1864 CrossRef PubMed.
- H. Kimura, M. Nakahara and N. Matubayasi, J. Phys. Chem. A, 2013, 117, 2102–2113 CrossRef PubMed.
- X. Yu, M. Wang and X. Huang, J. Mol. Liq., 2016, 216, 354–359 CrossRef.
|
This journal is © The Royal Society of Chemistry 2018 |
Click here to see how this site uses Cookies. View our privacy policy here.