DOI:
10.1039/C8RA04893B
(Paper)
RSC Adv., 2018,
8, 35681-35688
New nano-Fe3O4-supported Lewis acidic ionic liquid as a highly effective and recyclable catalyst for the preparation of benzoxanthenes and pyrroles under solvent-free sonication†
Received
7th June 2018
, Accepted 10th October 2018
First published on 18th October 2018
Abstract
A novel magnetic nanomaterial-immobilized Lewis acidic ionic liquid was successfully synthesized by the covalent embedding of 3-(3-(trimethoxysilyl)propyl)-1H-imidazol-3-ium chlorozincate (II) ionic liquid to the surface of Fe3O4 nanoparticles. The material was then characterized by FT-IR, SEM, TEM, TGA, ICP-OES, Raman, and EDS. Its performance as a new-generation Lewis acidic catalyst was also examined on the ultrasound-mediated synthesis of benzoxanthenes and pyrroles. Upon completion, the catalyst was simply recovered by an external magnet for multiple reuses without significant lessening of catalytic performance.
Introduction
Owing to their exceptional physical and chemical properties, ionic liquids (ILs) have gained increasing application as green solvents/catalysts for various organic transformations.1–4 Among them, Lewis acidic ionic liquids (LAILs) have been intensively studied as efficient alternatives for traditional Lewis acidic catalysts in many organic syntheses to facilitate higher yielding transformation to wanted products within short reaction times.5–8 Despite the mentioned preeminence, these LAILs still have several drawbacks such as the elaborate procedure for catalyst recovery and product isolation as well as the demand for the excessive amount of ILs in biphasic systems, which in turn would raise concerns about economical and toxicological issues.9,10 As a result, various solid-supported ionic liquids have been progressively developed to overcome those challenges thanks to their capability of being handled, separated, and recycled with ease.11–19 Recently, our group successfully employed a Brønsted acidic ionic liquid gel as a heterogeneous catalyst to assist the preparation of bisindolylmethanes.19 Despite the outstanding recyclability, low yields were inevitable because of the poor dispersion of the ionic liquid gel in the reaction mixture. Being aware of this shortcoming, relevant scientific communities are continuously directing their attention to employ high-surface-area materials as new-generation catalyst supports for multiple organic reactions. Among them, magnetic nanoparticles have been extensively studied on a variety of principal organic transformations including C–C, C–N, and C–O coupling since their magnetism can enable a much simplified work-up procedure where nanoparticle-supported catalysts are promptly separated from the reaction media by an external permanent magnet rather than filtration and centrifugation.12,13,20,21 However, in contrast to the widespread availability of organocatalysts impregnated on solid supports, the preparation of solid-immobilized ionic liquids has been still a great challenge. To the best of our knowledge, there have been only a few reports on the use of magnetic material-supported ionic liquids in some organic reactions.12,15–17,22–29 Many of them are the impregnated forms of Brønsted acidic ionic liquids. Therefore, the demand for designing new magnetic nanoparticle-supported Lewis acidic ionic liquids has still remained a topical interest since various organic transformations can be promoted by Lewis acidic species.
Based on the above fundamental understandings, we reported herein the designation of a novel magnetic nanoparticle-immobilized Lewis acidic ionic liquid and its usage as a prominent catalyst for the preparation of benzoxanthenes and pyrroles, two important heterocyclic scaffolds commonly present in various bioactive natural products, agrochemicals, and pharmaceuticals.30–44 Although a large number of catalytic procedures have been developed for quantitative synthesis of these heterocycles, the attempt of searching for a versatile catalyst fulfilling all green chemistry criteria, such as high-turnover property, excellent recyclability, simplicity in handling and recycling, and the capacity of promoting reactions under mild condition, has been still an unsolved problem. The initial results from our screening effort to find novel ionic liquid-based catalysts for certain organic reactions indicated that magnetic nanomaterial Fe3O4-supported Lewis acidic ionic liquid could be the best candidate most likely satisfying all mentioned requirements. Its outstanding catalytic performance over the multicomponent synthesis of benzoxanthenes and Paal–Knorr condensation to prepare pyrroles in this paper promises its prospectively common use as a novel catalyst in replacement of conventional Lewis acidic ones in organic synthesis. As far as we have known, this is the first report on the synthesis and catalytic reactivity of a magnetic nanoparticle-immobilized Lewis acidic ionic liquid.
Results and discussion
Characterization of LAIL@MNP
The magnetic nano-Fe3O4-immobilized Lewis acidic ionic liquid material (LAIL@MNP) was prepared in accordance to the protocol presented in Scheme 1. Initially, magnetite ferrite nanoparticle was formed via a co-precipitation method while the precursor ionic liquid was independently synthesized by the treatment of (3-chloropropyl)triethoxysilane with imidazole. Then the outermost surface of MNP was coated with the prepared ionic liquid. Finally, ZnCl2 was added and the resulting mixture was heated under reflux for 24 h to afford LAIL@MNP.
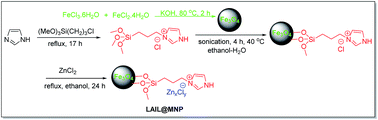 |
| Scheme 1 Synthesis of LAIL@MNP. | |
The grafting of LAIL@MNP was verified by FT-IR analysis. Fig. 1b and c showed the IR spectra of primitively synthesized LAIL@MNP and its recycled sample after the fifth recycling test, respectively. As expected, a good resemblance between two spectra can be seen over a wide range of absorption band, including peaks at 3430 cm−1 (broad absorption corresponding to Si–OH group and adsorbed water), 2921 cm−1 (aliphatic C–H stretch), 1620 cm−1 (C
N stretch of imidazolium ring), and a cluster of two peaks at 1095 and 1043 cm−1 (Si–O–Si stretch). These signals were not observed in the spectrum of plain Fe3O4 (Fig. 1a). This indicated that ionic liquid was successfully grafted onto the surface of the Fe3O4 nanoparticle.
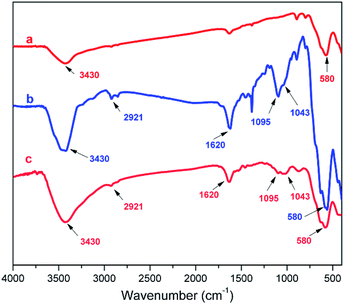 |
| Fig. 1 FT-IR spectra of Fe3O4 (a), primitively prepared LAIL@MNP (b), and the LAIL@MNP sample after the fifth recovery (c). | |
Scanning electron microscopy (SEM) image in Fig. 2 showed the morphology of LAIL@MNP which is depicted as uniform spherical particles. Transmission electron microscopy (TEM) confirmed that dark Fe3O4 core coated by grey silica shell has the average diameter of 10–15 nm (Fig. 2).
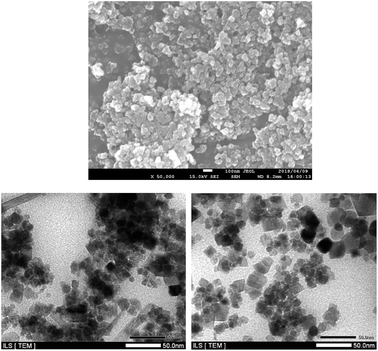 |
| Fig. 2 SEM image of LAIL@MNP (above) and TEM images (below) of as-prepared (left) and quinary recycled LAIL@MNP (right). | |
The thermostability of the LAIL@MNP was studied by thermogravimetric analysis (TGA) (Fig. 3). The TGA curve indicates an initial weight loss of 0.74% in the temperature range below 200 °C, most likely corresponding to the evaporation of residual water from the sample. The entire loss of the grafted LAIL was detected in the region from 220 °C to 400 °C. The percentage of LAIL, as determined, was approximately 3% of the total solid matrix. The Raman peak at 480 cm−1 can be interpreted as stretching vibration of Zn–Cl bond.45 The energy dispersive spectrum (EDS) showed the presence of C, N, O, Fe, Zn, Si, and Cl elements (Fig. 4). The composition of zinc element in LAIL@Fe3O4 was determined as 19
890 ppm (0.306 mmol g−1) by means of inductively coupled plasma mass spectrometry (ICP-MS).
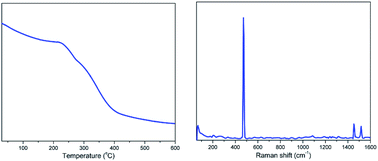 |
| Fig. 3 TGA curve of LAIL@MNP (left) and its Raman spectrum (right). | |
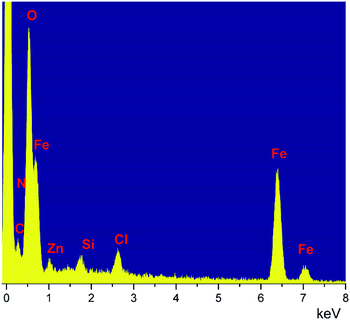 |
| Fig. 4 EDS spectrum of LAIL@MNP. | |
Synthesis of benzoxanthene derivatives
Initially, the catalytic activity of LAIL@MNP was surveyed in an ultrasound-assisted solvent-free multicomponent synthesis of pyran-annulated heterocyclic derivatives from 2-naphthol, dimedone, and benzaldehyde. As can be seen from Table S1,† significantly prolonged exposure to ultrasound (120 min) was necessitated for a quantitative formation of desired product (94%) at room temperature (entry 11). As expected, performing the reaction at an elevated temperature of 80 °C can bring the reaction to completion within 30 min (entry 18). Noticeably, the extremely poor yield of benzoxanthene was observed in control experiment where no catalyst was used (entry 23). Surprisingly, the addition of 1 mg of LAIL@MNP to the reaction mixture can give rise to a tremendous enhancement of reaction yield from 10% to 65% (entry 24). The transformation of given starting materials to the expected product can be brought to completion by increasing the catalyst loading from 1 to 15 mg (Table S1,† entry 18 and 24).
Subsequently, to illustrate the merit of LAIL@MNP, a variety of other catalysts were also studied for their activity with respect to the same reaction for the comparative purpose (Table S2†). In general, the investigated multicomponent reaction seems likely to be favored in acidic media as all metal halides, which are known as typical Lewis acids, can effectively furnish the benzoxanthene product in good yields of at least 80% (entry 5–9). Analogously, an array of different ionic liquids possessing weakly Brønsted acidic imidazolium species can also readily facilitate the formation of the same annulated product without any difficulty (entry 1–4). Meanwhile, most of the metal oxides, because of their predominant Lewis basicity, only exhibited moderate catalytic performance under the given reaction condition (entry 10–19). The invention of LAIL@MNP material having Lewis acidic zinc species immobilized onto magnetic Fe3O4 nanoparticle not only brought about a remarkable yield increment but also enable a quite simple work-up step for product isolation as well as catalyst recovery (entry 21). The effect of solvent on the studied reaction was also investigated, showing that low yield of product was observed with polar as well as nonpolar solvents (Table S2,† entries 22–36).
As can be seen from Table 1, the current method gave access to the desired product with the same ease as those reported in the literature. However, LAIL@MNP still gained prominence over other heterocyclic catalysts owing to its capability to enable the reaction under solventless condition. Additionally, the affordability of ZnCl2 also makes the protocol described herein more advantageous than the others of using expensive transition-metal catalysts.
Table 1 Comparative effectiveness of the current method versus former works for the three-component synthesis of benzoxanthenes
Entry |
Catalytic system |
Temp. (°C) |
Time (h) |
Yield (%) |
1 |
Nano Fe3O4/CS-Ag (15 mg) |
80 |
0.5 |
94 (ref. 46) |
2 |
Ru@SH-MWCNT (15% wt), EtOH/reflux |
80 |
0.5 |
85 (ref. 47) |
3 |
Nano-WO3-SO3H (19 mg) |
100 |
1.05 |
90 (ref. 48) |
4 |
W–ZnO (5.6 mol%), EtOH |
r.t. |
0.17 |
98 (ref. 49) |
5 |
Amberlyst-15 (200 mg), CH3CN |
Reflux |
5 |
94 (ref. 50) |
6 |
AIL@MNP (55 mg), dimedone (2.0 equiv.) |
90 |
0.75 |
89 (ref. 24) |
7 |
Current work: LAIL@MNP (15 mg), solvent-free sonication |
80 |
0.5 |
96 |
With the optimized condition in hand, we then examined the performance of catalyst over a wide range of aldehydes. As indicated in Scheme 2, the reaction generally worked well for saturated aliphatic as well as aromatic aldehydes. On the contrary, α,β-unsaturated aldehydes such as acrolein and cinnamaldehyde almost did not undergo the annulation with 2-naphthol and dimedone even under prolonged reaction time due to the positive charge delocalization arising from resonance effect. As expected, butyraldehyde with less steric hindrance at α-position was more reactive than cyclohexanecarbaldehyde. Under the same condition, 78% yield of the benzoxanthene derived from butyraldehyde was recorded, compared to the yield of 65% noted for the analogue produced from cyclohexanecarbaldehyde. Surprisingly, all benzaldehydes bearing either electron-donating or electron-withdrawing groups afforded the corresponding products from good to excellent yields (70–90%). Interestingly, steric hindrance of bulky o-substituents such as bromo or nitro in aromatic aldehydes did not bring about any detectable negative impact on the reaction as reported above for aliphatic aldehydes. The structural study of benzoxanthenes by X-ray crystallography in the previous literature revealed that the phenyl half is orthogonal rather than coplanar to the other half to minimize the steric strain of the whole molecule.51 Therefore, the steric effect of o-substituents in phenyl moiety is negligible owing to the flat structure of benzene ring. However, this is not the case for an aliphatic half like cyclohexyl since its uneven configuration always causes inevitable steric interaction regardless of its spatial arrangement in the molecule.
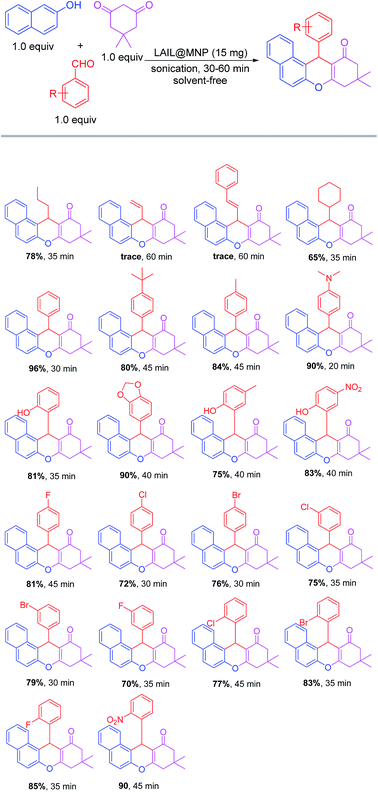 |
| Scheme 2 Reaction scope of the three-component condensation of 2-naphthol, dimedone, and aldehyde in the presence of Fe3O4@SiO2-IL-ZnxCly. | |
Based on the current results and previous literature,32,36,52,53 a proposed mechanistic profile for the synthesis of benzoxanthenes using LAIL@MNP is depicted in Scheme 3. According to this mechanism, the coordination between zinc species and carbonyl oxygen enhances the electrophilicity of carbonyl carbon, giving rise to a better nucleophilic attack of 2-naphthol to benzaldehyde to furnish α,β-unsaturated cyclic ketone intermediate (A′) after the loss of water molecule. Then Michael addition between this intermediate and the enol form of dimedone followed by the dehydration afforded the expected product.
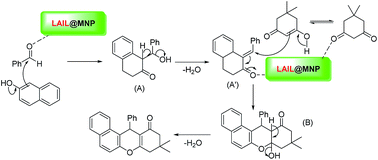 |
| Scheme 3 Proposed mechanism for one-pot multicomponent synthesis of benzoxanthenes. | |
Synthesis of pyrrole
The Paal–Knorr synthesis of 2,5-dimethyl-1-phenyl-1H-pyrrole from aniline and acetonylacetone was chosen as the second example to examine the catalytic performance of LAIL@MNP and the results are listed in Table S3.† In the presence of 15 mg of LAIL@MNP, the reaction proceeded smoothly to provide the expected pyrrole in quantitative yield of 91% after 30 min of sonication.
To exemplify the novelty of LAIL@MNP, the same reaction was probed over a wide range of catalysts and the results are presented in Table S4.† Similar to the above discussed multicomponent reaction, Paal–Knorr condensation has been also known to take place in slightly acidic rather than basic media. Consequently, all metal halides with Lewis acidic properties can straightforwardly bring the yield of pyrrole to more than 80% (entry 4–8), while metal oxides with amphoteric or basic nature only resulted in poor to moderate formation of the desired pyrrole under the same condition (entry 9–18). Without a rival, LAIL@MNP was again proven as the best candidate to induce a complete transformation to the target pyrrole (entry 20).
The comparison of the present method with formerly reported works was summarized in Table 2. Most obviously, the fact that LAIL@MNP promoted the synthesis of pyrrole under solvent-free condition can be easily outlined as the most noteworthy advantage compared with existing catalysts.
Table 2 Comparison of the present synthesis of pyrrole with previous works in literature
Entry |
Catalyst |
Temp.a (°C) |
Time (h) |
Yieldb (%) |
r.t.: room temperature. Isolated yield. |
1 |
Bi(NO3)3·5H2O (50 mol%), CH2Cl2 |
r.t. |
10 |
95 (ref. 54) |
2 |
I2 (0.1 mmol), THF |
r.t. |
9 |
89 (ref. 55) |
3 |
Sc(OTf)3 (5 mol%), CH2Cl2 |
r.t. |
0.5 |
77 (ref. 56) |
4 |
In(OTf)3 (5 mol%), CH2Cl2 |
r.t. |
0.5 |
90 (ref. 57) |
5 |
[BMIm]Cl |
r.t. |
3 |
96 (ref. 58) |
6 |
SbCl3/SiO2 (0.1 mmol), hexane |
r.t. |
1 |
91 (ref. 59) |
7 |
BiCl2/SiO2 (7.5 mmol), hexane |
r.t. |
1 |
92 (ref. 60) |
8 |
Vitamin B1 (5 mol%), ethanol |
r.t. |
1 |
90 (ref. 61) |
9 |
α-Zr(KPO4)2 (6 mol%) |
r.t. |
2 |
78 (ref. 62) |
10 |
Montmorillonite, KSF (1 g), CH2Cl2 |
r.t. |
10 |
95 (ref. 63) |
11 |
PS/AlCl3 (15 mol%), CH3CN |
Reflux |
1.25 |
90 (ref. 64) |
12 |
This work: LAIL@MNP, solvent-free sonication |
r.t. |
0.5 |
91 |
The tolerance of the presently studied Paal–Knorr reaction towards different functionalities was described in Scheme 4. In general, the reaction was apparently governed by both electronic and steric effect. Therefore, p-substituted anilines bearing an electron-donating (–OMe) or a weakly electron-withdrawing group (–I) can most easily undergo Paal–Knorr cyclization to offer corresponding products in quantitative yields more than 90%. Meanwhile, the analog containing strongly electron-withdrawing group, p-nitroaniline, can be only converted to the desired pyrrole with a quite modest yield of 48% even though the reaction time was extended up to 60 min. Shifting the methoxy group to ortho-position with respect to reactive amino site resulted in a diminished yield. The dominance of steric effect can be unambiguously observed for all o-substituted anilines bearing bulky groups, such as methyl, chloro, bromo, and phenyl. For instance, the pyrrole derived from 2,5-dichloroaniline was formed in much lower yield than those generated from 3,4- and 3,5-dichloroaniline isomers. Noticeably, regardless of its small size, o-methoxy group was also able to exhibit a certain steric effect suppressing the complete transformation to desired products wherein pyrroles derived from 2-hydroxy-5-methylaniline and 2-hydroxy-5-nitroaniline were isolated in 78 and 63% yield, respectively. Interestingly, selective pyrrole cyclization can be achieved thanks to the directing effect of present substituents. As illustrated in Scheme 4, only the amino group at meta-position to nitro substituent was exclusively subjected to the Paal–Knorr condensation with acetonylacetone while the other was intact. Finally, the extension of substrate scope to phenylhydrazines and triethylenetetramine was also applicable for this method.
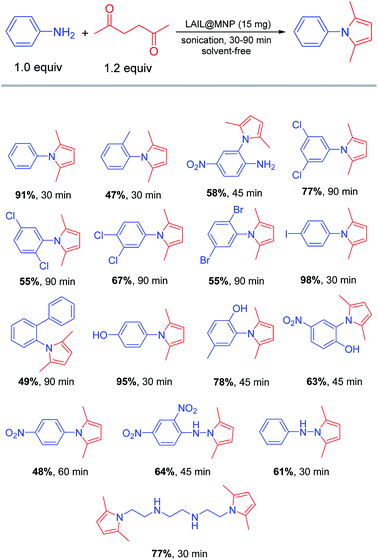 |
| Scheme 4 Synthesis of pyrrole derivatives. | |
A proposed mechanism for the formation of pyrrole catalyzed by LAIL@MNP is depicted in Scheme 5. In a similar fashion to the above mechanism for the multicomponent synthesis of benzoxanthene, carbonyl oxygen of acetonylacetone is first coordinated to zinc center to generate a better electrophilic species which more readily reacts with amine to give intermediate adduct (C). Following intramolecular nucleophilic cyclization with the loss of two molecules of water delivers the desired pyrrole.
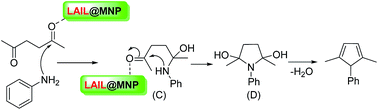 |
| Scheme 5 A plausible mechanism for the synthesis of pyrroles. | |
The recyclability of LAIL@MNP was examined over both benzoxanthene and pyrrole synthesis under the reported optimized condition (Fig. 5). Upon the completion of each reaction, the corresponding product was diluted with ethyl acetate (15 mL) and the catalyst was separated by a magnet. The restored catalyst was washed with ethyl acetate (2 × 5 mL) and ethanol (3 × 5 mL). Then it was desiccated in vacuo for 2 h and reutilized in the next recycling experiments. Nearly quantitative LAIL@MNP could be recovered from each run. The stability and efficacy of LAIL@MNP remained constant through five consecutive recycling tests. No apparent modification in morphology and size of particles was detected in the TEM image of primitively prepared as well as recovered sample of LAIL@MNP (Fig. 2). The FT-IR spectra of LAIL@MNP samples also suggested no obvious change in functionality (Fig. 1).
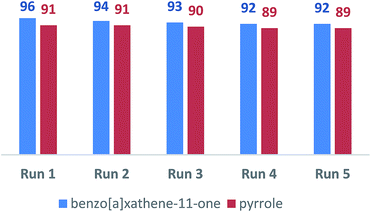 |
| Fig. 5 Recycling tests. | |
The primary issue of heterogeneous catalysts is the leaching of reactive species from solid support, resulting in deactivation of the catalyst. To evaluate the leaching effect of LAIL@MNP, the reaction between aniline and acetonylacetone was temporarily stopped after 10 min by diluting the ongoing reaction mixture with a sufficient amount of ethyl acetate. The catalyst was then removed and the ethyl acetate solution was analyzed by GC, indicating that 58% yield of pyrrole was formed at that time. Next, ethyl acetate solvent was decanted and the resultant free-catalyst reaction mixture was sonicated for further 20 min. At the end of this stage, the yield of pyrrole only slightly increased from 58 to 62%, showing that only a trace amount of zinc species was leached into the reaction mixture during the reaction course. The leaching level of LAIL@MNP was also evaluated by ICP-OES whereby the Zn content in reaction mixture was determined as 1.8 ppm, indicating a negligibly slight leaching from the solid support.
Experimental
Preparation of magnetic Fe3O4 nanoparticle (MNPs)65
MNP was synthesized by simple co-precipitation of ferrous and ferric ions in a basic condition medium. Typically, FeCl3·6H2O (20 mmol) and FeSO4·7H2O (10 mmol) were dissolved in 100 mL deionized water. The mixture was stirred at 80 °C and then KOH (12 mmol) was added and stirred continuously within 2 h. The black precipitate, after being collected by a permanent magnet, was washed with water (3 × 100 mL) and ethanol (3 × 50 mL). This MNP material was dried at 60–70 °C under vacuo for 1 h.
General procedure for the synthesis of LAIL@MNP
The imidazolium chloride ionic liquid was synthesized pursuant to a procedure reported previously.66 A mixture of 3-chloroethoxy-propylsilane (5.0 mmol, 1.2 mL) and imidazole (5.0 mmol, 0.34 g) was stirred at reflux for 17 h. The ionic liquid obtained as a yellowish viscous liquid was diluted in 50 mL of ethanol–water (1
:
1 volume ratio) solution. To the freshly prepared suspension of MNP material in 100 mL of 1
:
1 ethanol–water was added the above ionic liquid solution and the mixture was sonicated at 40 °C for 4 h. The resultant IL@MNP was washed with dichloromethane and dried at 70 °C in vacuo. To synthesize LAIL@MNP, a mixture of IL@MNP (1.0 g) and ZnCl2 (1.0 mmol) in ethanol (50 mL) was refluxed for 24 h. After cooling to room temperature, the catalyst was separated by a magnet, washed with ethanol, and dried at 100 °C for 5 h. The LAIL@MNP was characterized by FT-IR, SEM, TEM, TGA, Raman, and SEM-EDS. The loading amount of Zn metal was determined based on ICP-OES.
General procedure for one-pot multicomponent reaction
A mixture of benzaldehyde (1.0 mmol, 0.106 g), 2-naphthol (1.0 mmol, 0.144 g), and dimedone (1.0 mmol, 0.140 g) was reacted under solvent-free sonication in the presence of LAIL@MNP (15 mg) at 80 °C for 30 min. Upon completion of the reaction (monitored by TLC), the resulting mixture was diluted by ethyl acetate (15 mL) and the solid catalyst was eliminated from organic solution by a magnet. The catalyst was washed with ethyl acetate (2 × 5 mL) followed by ethanol (3 × 5 mL) and then reused for next cycles after drying under vacuum. Meanwhile, the organic solution was dried over MgSO4 and then concentrated under reduced pressure. The resultant crude product was recrystallized from ethanol to yield pure benzoxanthene whose structure was verified by 1H and 13C NMR.
General procedure for Paal–Knorr reaction
A mixture of aniline (1.0 mmol), acetonylacetone (1.2 mmol) and LAIL@MNP (15 mg) was reacted under solvent-free sonication within an appropriate interval. Upon completion of the reaction (monitored by TLC and GC), the resulting mixture was diluted by ethyl acetate (15 mL) and the solid catalyst was eliminated from the organic solution by a magnet. The catalyst was washed with ethyl acetate (2 × 5 mL) followed by ethanol (3 × 5 mL) and then reused for next cycles after drying under vacuum. Meanwhile, the organic solution was dried over MgSO4 and then concentrated under reduced pressure. The crude product was purified through column chromatography using hexane–ethyl acetate (9
:
1). The purified pyrrole was then authenticated by 1H and 13C NMR, GC-MS or HRMS (ESI).
Conclusions
We have designed a novel magnetically separable LAIL@MNP material which can be used as a green solid Lewis acid catalyst in various organic reactions. Embedding reactive Lewis acidic sites onto high-surface area Fe3O4 nanomaterial greatly overcame the issue of poor dispersion which is common for heterogeneous catalysts. Owing to this feature, LAIL@MNP can exhibit better catalytic performance than conventional Lewis acid in acid-mediated organic reactions such as multicomponent and Paal–Knorr reactions to synthesize benzoxanthenes and pyrroles, respectively, in good to excellent yields. Additionally, the efficiency of the protocol was also owing to the use of ultrasonic irradiation by means of which locally high collapse temperature induced by the successive formation and collapse of cavitation bubbles in reaction media can extraordinarily enhance the reaction rate. Moreover, the catalyst was easily separated from the reaction mixture by an external magnetic field and reused without any significant loss of catalytic activity after five runs.
Conflicts of interest
There are no conflicts to declare.
Acknowledgements
This project is financially supported by Vietnam National Foundation for Science and Technology Development (NAFOSTED) through research grant 104.01–2016.59.
Notes and references
- M. A. Martins, C. P. Frizzo, A. Z. Tier, D. N. Moreira, N. Zanatta and H. G. Bonacorso, Chem. Rev., 2014, 114, PR1–PR70 CrossRef PubMed.
- Q. Wang, W. Hou, S. Li, J. Xie, J. Li, Y. Zhou and J. Wang, Green Chem., 2017, 19, 3820–3830 RSC.
- K. Goossens, K. Lava, C. W. Bielawski and K. Binnemans, Chem. Rev., 2016, 116, 4643–4807 CrossRef CAS PubMed.
- D. J. Kim, K. H. Oh and J. K. Park, Green Chem., 2014, 16, 4098–4101 RSC.
- A. S. Amarasekara, Chem. Rev., 2016, 116, 6133–6183 CrossRef CAS PubMed.
- H. T. Nguyen and P. H. Tran, RSC Adv., 2016, 6, 98365–98368 RSC.
- X. Zhang, R. Zhang, X. Meng, H. Liu, Z. Liu, H. Ma, C. Xu and P. A. A. Klusener, Appl. Catal., A, 2018, 557, 64–71 CrossRef CAS.
- F. Abbasi, N. Azizi and M. Abdoli-Senejani, Appl. Organomet. Chem., 2017, 31, 3790–3796 CrossRef.
- H. Niedermeyer, J. P. Hallett, I. J. Villar-Garcia, P. A. Hunt and T. Welton, Chem. Soc. Rev., 2012, 41, 7780–7802 RSC.
- J. P. Hallett and T. Welton, Chem. Rev., 2011, 111, 3508–3576 CrossRef CAS PubMed.
- P. C. Marr and A. C. Marr, Green Chem., 2016, 18, 105–128 RSC.
- A. Pourjavadi, S. H. Hosseini, M. Doulabi, S. M. Fakoorpoor and F. Seidi, ACS Catal., 2012, 2, 1259–1266 CrossRef CAS.
- R. K. Sharma, S. Dutta, S. Sharma, R. Zboril, R. S. Varma and M. B. Gawande, Green Chem., 2016, 18, 3184–3209 RSC.
- J. D. Patil, S. A. Patil and D. M. Pore, RSC Adv., 2015, 5, 21396–21404 RSC.
- N. Azgomi and M. Mokhtary, J. Mol. Catal. A: Chem., 2015, 398, 58–64 CrossRef CAS.
- H. N. Dadhania, D. K. Raval and A. N. Dadhania, Catal. Sci. Technol., 2015, 5, 4806–4812 RSC.
- X. Liang, Ind. Eng. Chem. Res., 2014, 53, 17325–17332 CrossRef CAS.
- Y.-M. Wang, V. Ulrich, G. F. Donnelly, F. Lorenzini, A. C. Marr and P. C. Marr, ACS Sustainable Chem. Eng., 2015, 3, 792–796 CrossRef CAS.
- P. H. Tran, X.-T. T. Nguyen and D.-K. N. Chau, Asian J. Org. Chem., 2018, 7, 232–239 CrossRef CAS.
- B. Zhen, Q. Jiao, Y. Zhang, Q. Wu and H. Li, Appl. Catal., A, 2012, 445–446, 239–245 CrossRef CAS.
- Y. Kong, R. Tan, L. Zhao and D. Yin, Green Chem., 2013, 15, 2422–2433 RSC.
- Y. Zhang and C. Xia, Appl. Catal., A, 2009, 366, 141–147 CrossRef CAS.
- J. Miao, H. Wan and G. Guan, Catal. Commun., 2011, 12, 353–356 CrossRef CAS.
- Q. Zhang, H. Su, J. Luo and Y. Wei, Green Chem., 2012, 14, 201–208 RSC.
- B. Karimi, F. Mansouri and H. Vali, Green Chem., 2014, 16, 2587–2596 RSC.
- P.-H. Li, B.-L. Li, H.-C. Hu, X.-N. Zhao and Z.-H. Zhang, Catal. Commun., 2014, 46, 118–122 CrossRef CAS.
- Z. Wu, Z. Li, G. Wu, L. Wang, S. Lu, L. Wang, H. Wan and G. Guan, Ind. Eng. Chem. Res., 2014, 53, 3040–3046 CrossRef CAS.
- H. N. Dadhania, D. K. Raval and A. N. Dadhania, Res. Chem. Intermed., 2017, 44, 117–134 CrossRef.
- Q. Zhang, X.-M. Ma, H.-X. Wei, X. Zhao and J. Luo, RSC Adv., 2017, 7, 53861–53870 RSC.
- J. Safaei-Ghomi and F. Eshteghal, Ultrason. Sonochem., 2017, 38, 488–495 CrossRef CAS PubMed.
- N. Azizi and F. Shirdel, J. Mol. Liq., 2016, 222, 783–787 CrossRef CAS.
- F. Taghavi, M. Gholizadeh, A. S. Saljooghi and M. Ramezani, RSC Adv., 2016, 6, 87082–87087 RSC.
- A. R. Moosavi-Zare, M. A. Zolfigol, O. Khaledian, V. Khakyzadeh, M. D. Farahani, M. H. Beyzavi and H. G. Kruger, Chem. Eng. J., 2014, 248, 122–127 CrossRef CAS.
- A. R. Moosavi-Zare, M. A. Zolfigol, M. Zarei, A. Zare and V. Khakyzadeh, J. Mol. Liq., 2013, 186, 63–69 CrossRef CAS.
- J. M. Khurana and D. Magoo, Tetrahedron Lett., 2009, 50, 4777–4780 CrossRef CAS.
- G. C. Nandi, S. Samai, R. Kumar and M. S. Singh, Tetrahedron, 2009, 65, 7129–7134 CrossRef CAS.
- A. Kornienko and J. J. La Clair, Nat. Prod. Rep., 2017, 34, 1051–1060 RSC.
- M. Fleige and F. Glorius, Chem.–Eur. J., 2017, 23, 10773–10776 CrossRef CAS PubMed.
- F. Bonyasi, M. Hekmati and H. Veisi, J. Colloid Interface Sci., 2017, 496, 177–187 CrossRef CAS PubMed.
- A. Kamal, S. Faazil, M. Shaheer Malik, M. Balakrishna, S. Bajee, M. R. H. Siddiqui and A. Alarifi, Arabian J. Chem., 2016, 9, 542–549 CrossRef CAS.
- L. Zhang, J. Zhang, J. Ma, D.-J. Cheng and B. Tan, J. Am. Chem. Soc., 2017, 139, 1714–1717 CrossRef CAS PubMed.
- J. Sączewski, J. Fedorowicz, M. Gdaniec, P. Wiśniewska, E. Sieniawska, Z. Drażba, J. Rzewnicka and Ł. Balewski, J. Org. Chem., 2017, 82, 9737–9743 CrossRef PubMed.
- Z. Gong, Y. Lei, P. Zhou and Z. Zhang, New J. Chem., 2017, 41, 10613–10618 RSC.
- H. Truong Nguyen, D.-K. Nguyen Chau and P. H. Tran, New J. Chem., 2017, 41, 12481–12489 RSC.
- H. Pir, N. Günay, Ö. Tamer, D. Avci, E. Tarcan and Y. Atalay, Mater. Sci.-Pol., 2013, 31, 357–371 CrossRef.
- E. E. Reza Mohammadi, M. Ghavami and M. Z. Kassaee, J. Mol. Catal. A: Chem., 2014, 393, 309–316 CrossRef.
- K. Tabatabaeian, M. A. Zanjanchi, M. Mamaghani and A. Dadashi, Res. Chem. Intermed., 2016, 42, 5049–5067 CrossRef CAS.
- S. R. Ali Amoozadeh, J. Mol. Catal. A: Chem., 2015, 396, 96–107 CrossRef.
- F. S. Arbosara, F. Shirini, M. Abedini and H. F. Moafi, J. Nanostruct. Chem., 2015, 5, 55–63 CrossRef CAS.
- B. Das, B. Ravikanth, R. Ramu, K. Laxminarayana and B. V. Rao, J. Mol. Catal. A: Chem., 2006, 255, 74–77 CrossRef CAS.
- K. Aggarwal and J. M. Khurana, J. Mol. Struct., 2015, 1079, 21–34 CrossRef CAS.
- X. J. Sun, J. F. Zhou and P. S. Zhao, J. Heterocycl. Chem., 2011, 48, 1347–1350 CrossRef CAS.
- V. Rama, K. Kanagaraj and K. Pitchumani, Tetrahedron Lett., 2012, 53, 1018–1024 CrossRef CAS.
- B. K. Banik, I. Banik, M. Renteria and S. K. Dasgupta, Tetrahedron Lett., 2006, 46, 2643–2645 CrossRef.
- R. H. Peiris, C. Hallé, H. Budman, C. Moresoli, S. Peldszus, P. M. Huck and R. L. Legge, Water Res., 2010, 44, 185–194 CrossRef CAS PubMed.
- J. Chen, H. Wu, Z. Zheng, C. Jin, X. Zhang and W. Su, Tetrahedron Lett., 2006, 47, 5383–5387 CrossRef CAS.
- A. Rahmatpour, J. Organomet. Chem., 2012, 712, 15–19 CrossRef CAS.
- B. Wang, Y. Gu, C. Luo, T. Yang, L. Yang and J. Suo, Tetrahedron Lett., 2004, 45, 3417–3419 CrossRef CAS.
- M. R. P. Hossein Reza Darabi, K. Aghapoor, A. Mirzaee, F. Mohsenzadeh, N. Asadollahnejad, H. Taherzadeh and Y. Balavar, Environ. Chem. Lett., 2012, 10, 5–12 CrossRef.
- K. Aghapoor, L. Ebadi-Nia, F. Mohsenzadeh, M. Mohebi Morad, Y. Balavar and H. R. Darabi, J. Organomet. Chem., 2012, 708–709, 25–30 CrossRef CAS.
- K. A. Hossein Reza Darabi and F. M. Abbas Darestani Farahani, Environ. Chem. Lett., 2012, 10, 369–375 CrossRef.
- M. Curini, F. Montanari, O. Rosati, E. Lioy and R. Margarita, Tetrahedron Lett., 2003, 44, 3923–3925 CrossRef CAS.
- B. K. Banik, S. Samajdar and I. Banik, J. Org. Chem., 2004, 69, 213–216 CrossRef CAS PubMed.
- A. Rahmatpour and J. Aalaie, Heteroat. Chem., 2011, 22, 85–90 CrossRef CAS.
- J. Safari and Z. Zarnegar, C. R. Chim., 2013, 16, 920–928 CrossRef.
- S. Nazari, S. Saadat, P. K. Fard, M. Gorjizadeh, E. R. Nezhad and M. Afshari, Monatsh. Chem., 2013, 144, 1877–1882 CrossRef CAS.
Footnote |
† Electronic supplementary information (ESI) available. See DOI: 10.1039/c8ra04893b |
|
This journal is © The Royal Society of Chemistry 2018 |
Click here to see how this site uses Cookies. View our privacy policy here.