DOI:
10.1039/C8RA05156A
(Paper)
RSC Adv., 2018,
8, 29172-29178
Adverse effect of nano-TiO2 on the marine macroalgae Gracilaria lemaneiformis (Gracilariales, Rhodophyta): growth and antioxidant activity
Received
15th June 2018
, Accepted 7th August 2018
First published on 16th August 2018
Abstract
Macroalgae, the major contributor of primary productivity in coastal seas, contribute to the material cycle and energy flow in marine ecosystems. The purpose of this work was to evaluate the toxic effect of nano-TiO2 on the growth and antioxidant activity of Gracilaria lemaneiformis. An obvious inhibition of growth was observed in this study. The algae exposed to nano-TiO2 showed a negative growth rate at 20 mg L−1 and 40 mg L−1 during the 15 days exposure. The concentration of soluble protein increased slightly during the first 3 days of exposure, but it gradually diminished thereafter due to the high concentrations of nano-TiO2 and to prolonged exposure. Nano-TiO2 caused oxidative damage in G. lemaneiformis; superoxide anions accumulated, and nitrate reductase activity decreased linearly with the increase in nano-TiO2. Furthermore, extracts of G. lemaneiformis can scavenge DPPH· and hydroxyl radicals for their antioxidant capacity. However, the capacity to scavenge DPPH· and hydroxyl radicals in vitro decreased slightly with the increase in nano-TiO2. The results from this work imply that macroalgae can be an effective biomarker of nano-TiO2 contamination and can be useful indicators to evaluate the oxidative damage of increasing pollutants in marine ecosystems.
Introduction
Macroalgae are important primary producers and play a key role in estuarine and coastal ecosystems. The species and quantity of algae can directly reflect water quality.1 Macroalgae can absorb and utilize nutrients to control eutrophication, and they can improve the quality and stability of marine ecosystems.2,3 The use of seaweeds has become more popular in recent years. They contain a variety of bioactive compounds rich in polysaccharides, carotenoids, essential fatty acids and antioxidants, and they have the potential to replace synthetic compounds such as TBHQ (tert-butylhydroquinone) and BHA (butylated hydroxy anisole), which are considered less healthy for humans.4 However, changes in the environment, such as hazardous substances, solar radiation, and temperature, can affect the biochemical and physiological responses of algae.5
Nanoparticles, which constitute a heterogeneous group of nanostructures, are being increasingly applied in a wide variety of products.6 In particular, nano-TiO2 has been utilized in cosmetics, coatings and environmental remediation.7–9 The annual global commercial production of nano-TiO2 was more than 10
000 metric tons per year, and it is expected to reach approximately 2.5 million metric tons by 2020.10 However, nanoparticles are inevitably released into the environment during the process of manufacturing, use and disposal.11 Nanoparticles, which possess mobility and have many reactive sites on their surface, may be hazardous to the health of organisms and to the environment.12 Tian et al.13 reported that the annual input concentration of nano-TiO2 in the sediment can reach 1.9 mg L−1 in the U.S., Switzerland and Europe. The nano-TiO2 concentration in environmental media, including sediment, surface water, and natural and urban soils, was estimated to be in the range of mg kg−1 to low mg kg−1, which is much higher than other nanoparticles.14,15 Nano-TiO2 with different diameters have different biotoxicity to algae. Sendra found that nano-TiO2 is more toxic than bulk TiO2.16 Theoretically, nanoparticles could enter the cell wall of algae, or they could damage the cell wall when their size is smaller than 40 nm.17 Simultaneously, TiO2 nanoparticles act as a carrier can enhance heavy metal ion accumulation in the algae.18 Organic properties also have significant effects on the toxic behavior of engineered nanomaterials.19 Therefore, a large number of aquatic organisms, such as algae, fungi, and plants, are mostly likely affected by nanoparticles. There is a growing concern about the potential threat of nanoparticles to humans and aquatic organisms, especially microalgae.20 Studies have already shown that nanoparticles can inhibit the growth and photosynthetic capacities of algae, as well as cause oxidative stress and alter enzymatic activity.21,22 A large number of nanotoxicological studies have shown that free radical formation is the main mechanism of the toxicity of nanoparticles, which subsequently induces oxidative stress, cytotoxicity, DNA damage, and apoptosis.23,24
Gracilaria lemaneiformis Greville (Bory de Saint-Vincent), an edible red macroalgae, is cultivated in the southeast coastal area of China, and it is widely used in food additives, medicine, cosmetics and other industries. It plays a significant role in the restoration of eutrophic waters along the coast of China and is an important material in agar and other natural product extraction.25 In addition, G. lemaneiformis is a valuable fodder for marine animals, and therefore there is a large demand for this macroalgae in China. The growing demand for G. lemaneiformis has caused it to become the focus of many studies. Recently, various studies have focused on the effects of changes in the environment and of pollutants, such as metal, NiO nanoparticles and ultraviolet radiation, on the growth of G. lemaneiformis.26–28 Research has shown that heavy metals have a negative effect on the growth, pigment content, and photosynthesis of G. lemaneiformis.29,30 Other studies have also shown that seaweed is an important indicator in pollutant toxicity evaluation.31 However, research about the potential toxicity of nanoparticles on macroalgae is rare. Many studies have revealed the presence of various antioxidants in algae, such as polysaccharides, amino acids, dietary fibres, proteins, polyphenols, vitamins and carotenoids.32 Therefore, macroalgae are a potential source of new antioxidants. Nevertheless, the effect of nanoparticles stress on the scavenge free radical activity of macroalgae has not been studied.
Therefore, the primary aim of this work is to investigate the toxic effects of nano-TiO2 on G. lemaneiformis. First, we measured the growth, soluble protein (SP) content and oxidative stress of G. lemaneiformis. Second, we investigated the negative impact of 15 days of exposure to nano-TiO2 on the antioxidant activities of G. lemaneiformis.
Material and methods
Cultivation and treatment of algae
G. lemaneiformis used in this study was collected from a culture zone in Nanao Island (116.6° E, 23.3° N), Guangdong Province, China. After harvesting, the healthy algae were washed to remove visible epiphytes and accumulated sediment. Then, the thalli were continuously acclimatized in Von Stosch enriched (VSE) medium for a week under the following conditions: salinity of 30‰, temperature of 20 ± 1 °C, illumination of 2000 lx, light/dark cycles of 12 h
:
12 h. The culture medium was renewed every other day. After pre-culture, approximately 4 g fresh weight (FW) of healthy thalli were moved to a conical flask with 1 L of culture medium for 3, 7, and 15 days; the culture medium was supplemented with different concentrations of nano-TiO2 ranging from 0, 5, 10, 20, and 40 mg L−1 (three replicates per concentration). Moreover, all other culture conditions remained the same as during the acclimatization period. Nano-TiO2 was acquired from Aladdin (Shanghai, China) and was dissolved in ultrapure water to prepare the stock solutions (0.5 g L−1). The selection of concentrations and the preparation of the nano-TiO2 stock solution were described in previous research.33
Characterization of nanoparticles
Nano-TiO2 size and morphology analyses were performed by transmission electron microscopy (TEM) at an accelerating voltage of 80 kV. A nano-TiO2 suspension was prepared with algal test medium to a concentration of 20 mg L−1. After 30 min of ultrasonic treatment (100 W, 40 kHz, 30 min), nano-TiO2 was applied to a copper grid and air-dried under sunlight for 2 h. Finally, the size and morphology of nano-TiO2 was measured by TEM. After being sonicated in the algal test medium with a particle concentration of 20 mg L−1, the hydrodynamic size and zeta-potential of the nano-TiO2 were characterized by dynamic light scattering (DLS) using a Zetasizer Nano ZS (Malvern Instrument, Westborough, MA).
Measurement of the growth rate and soluble protein
The relative growth rates (RGRs) for the control and treatment groups were calculated from the following formula:34
RGR% d−1 = ln(Wt/W0)/t × 100% |
where W0 refers to the initial FW and Wt is the FW at the end of the experiment. t is the cultivation time (15 days). SP was extracted at 0–4 °C in a phosphate buffer (50 mM, pH 5.5) by grinding 0.5 g FW with a mortar. The homogenates were then centrifuged (2000 rpm, 20 min, 4 °C), and the supernatants were used to determine the SP concentration.35 The analysis of SP concentration was performed with a Coomassie Brilliant Blue Kit according to the manufacturer's instructions (Jiancheng Biotech, Nanjing, China). The absorbance was read at 595 nm by UV-VIS spectrophotometry.
Nitrate reductase activity
The activity of nitrate reductase (NR) was measured by an in vitro assay.36 Briefly, 0.2 g (FW) of thalli was cut into smaller pieces and placed into test tubes containing 5 mL of the assay medium (0.1 M phosphate buffer, pH 7.5, 0.01 mM glucose, 200 mM NaNO3, 0.5 mM Na-EDTA). Then, the mixture was flushed with nitrogen for 2 min (to eliminate the O2 in the medium). Finally, the test tubes were quickly sealed and incubated in the dark for 1 h at 30 °C. After, 1 mL of each extract was loaded into another test tube with 2 mL of a sulfonamide reagent; 5 min later, 2 mL of a hydrochloric acid naphthalene ethylene diamine reagent was added to each tube, and the mixture was allowed to stand for 15 min. Then, the absorbance was read by UV-VIS spectrophotometry at 540 nm.
Superoxide anion free radical content
The level of superoxide anion free radicals (O2˙−) in G. lemaneiformis was determined according to Luo and Wang with some modifications.37 For this analysis, 0.5 g of algae was ground with 3 mL of Na2HPO4–NaH2PO4 buffer (50 mM, pH 7.8) and centrifuged at 10
000 rpm for 10 min. After, 1 mL of supernatant, 0.9 mL of Na2HPO4–NaH2PO4 buffer (50 mM, pH 7.8), and 0.1 mL of hydroxylamine hydrochloride (10 mM) were added to a test tube and mixed homogeneously. Then, the mixture was incubated for 20 min. After, 1 mL of α-naphthylamine (7 mM) and 1 mL of sulfanilic acid (17 mM) were added to the mixture; 20 min later, 1.5 mL of n-butanol was added to the mixture, and the mixture was centrifuged for 10 min at 6000 rpm. The absorbance was read at 530 nm. The level of O2˙− was calculated by using sodium nitrite as a standard solution.
Antioxidant activities of aqueous seaweed extracts
Preparation of the algal extract. G. lemaneiformis was cultured for 15 days in different concentrations of nano-TiO2. Then, the algae were dried and ground into a fine powder. Aqueous extracts of G. lemaneiformis (AEGL) were prepared according to Zandi et al.38 In total, 1.0 g of dried seaweed was extracted in ultrapure water at a ratio of 1
:
100 (w/v). Then, the algae homogenate was centrifuged (7500 rpm, 15 min). The supernatant was filtered through Whatman No. 1 filter paper, sterilized with a Millipore filter with a 0.22 μm pore size and stored at −20 °C. The crude extract was diluted with ultrapure water to 1 mg mL−1 prior to use.
Antioxidant assay for DPPH· radical-scavenging activity. The DPPH· radical-scavenging activity of AEGL was measured according to Yen and Chen.39 A 2.0 mL aliquot of the extract was added to 2.0 mL of a 0.16 mM DPPH· methanol solution. The reaction mixture was vortexed for 1 min and then left to stand for 30 minutes at 25 °C in the dark. Its absorbance was measured at 517 nm. The ability to scavenge DPPH· radicals was calculated by the following formula:
Scavenging effect (%) = [1 − (As − A0)/Ac] × 100 |
where Ac, As, A0 were the absorbance values determined at 517 nm of the control (DPPH· solution without sample), the test sample (DPPH· solution plus test sample), and the sample only (sample without DPPH· solution), respectively.
Hydroxyl radical scavenging activity. The hydroxyl radical (·OH) scavenging activity of AEGL was carried out by the method of a previous study with some modifications.40 Briefly, a Na2HPO4–NaH2PO4 buffer (2.0 mL, 0.2 M, pH 7.40), 10-phenanthroline solution (1.0 mL, 1.865 mM), and AEGL (1.0 mL, 1.0 mg mL−1) were added to a test tube and mixed homogeneously. Then, a FeSO4·7H2O solution (1.0 mL, 1.865 mM) was added to the test tube. The reaction was induced by H2O2 (1.0 mL 0.03%). After incubation in a water bath for 60 min at 37 °C, the absorbance of the reactant solution was detected at 536 nm. The reactant solution without AEGL was used as a negative control, and a sample with no H2O2 was used as a blank control. The scavenging activity of ·OH was calculated using the following equation:
Scavenging effect (%) = [(As − An)/(Ab − An)] × 100 |
where As is the absorbance of the sample, An is the absorbance of the negative control, and Ab is the absorbance of the blank control.
Statistical analyses
All the experimental data are represented as the mean ± SD. Statistical differences were analysed using one-way analysis of variance followed by a Tukey test. *P < 0.05 and **P < 0.01 represent significant and very significant differences between the control and the test samples, respectively.
Results
Characterization of the nano-TiO2
The zeta potential of the nano-TiO2 suspension in the algal test medium is −34.5 ± 1.27 mV and the hydrodynamic size distribution of nano-TiO2 is 53.3–174.3 nm. The results of hydrodynamic size were indicated size vs. intensity. The particle size of the nano-TiO2 diluted with the algal test medium was observed by TEM (Fig. 1). The average particle size of nano-TiO2 was calculated to be less than 20 nm.
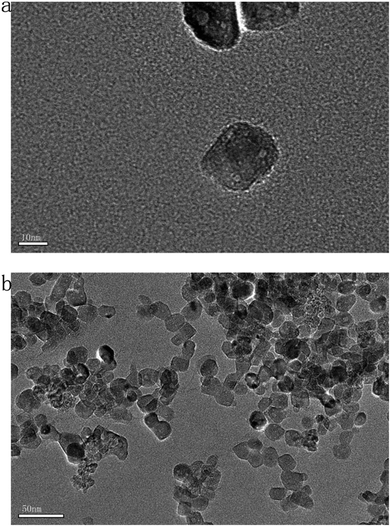 |
| Fig. 1 Nano-TiO2 characterized by TEM: (a) isolated nano-TiO2 suspended in ultrapure water, (b) agglomerated nano-TiO2 suspended in ultrapure water. (Where (a) and (b) have scales of 10 nm and 50 nm, respectively). | |
Effect of nano-TiO2 on the growth rate and SP content of G. lemaneiformis
After 15 days of culture, the RGRs of the G. lemaneiformis decreased gradually with the increase in nano-TiO2 concentration (Fig. 2). A minimum RGRs of −0.31% per day occurred at a nano-TiO2 concentration of 40 mg L−1, and RGRs decreased up to 127.0% compared to the control group. The growth rate of G. lemaneiformis decreased with the increase in nano-TiO2 concentration and with the time of migration, which showed that nano-TiO2 could interfere with the growth of G. lemaneiformis. The SP concentration of G. lemaneiformis was investigated (Fig. 3). Compared with the control group, the content of SP in G. lemaneiformis with exposure to nano-TiO2 slightly increased until the 3rd day (P > 0.05). However, after 7 and 15 days of exposure to nano-TiO2, the SP content decreased. Notably, the content of SP reached a minimum with a 1.91-fold decline at 40 mg L−1 of nano-TiO2 at 15 days.
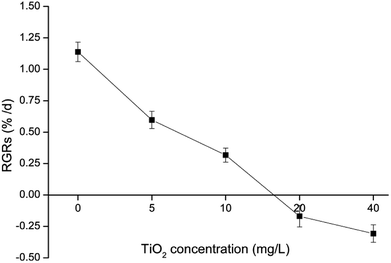 |
| Fig. 2 The effect of nano-TiO2 on the relative growth rates (RGRs) of G. lemaneiformis after 15 days of incubation. | |
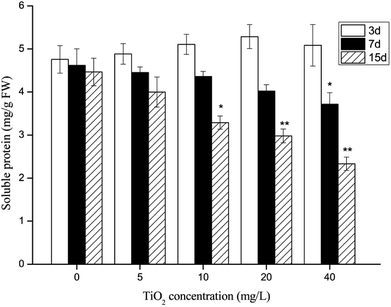 |
| Fig. 3 The effect of nano-TiO2 on soluble protein (SP) content of G. lemaneiformis. Data are presented as the mean ± SD of three replicates. *P < 0.05, **P < 0.01. | |
Effects of nano-TiO2 on NR activity
During the experiment, the NR activity of G. lemaneiformis was affected by the nano-TiO2 concentration and by culture time (Fig. 4). After 3 days of treatment, the activity of NR did not vary, but there was a marked change in NR activity after 7 and 15 days of nano-TiO2 exposure. Compared with the control group, the activity of NR decreased by 1.1–6.6-fold (P < 0.05). Furthermore, when the nano-TiO2 concentration increased, the activity of NR visibly decreased, showing that NR damage occurred in a dose–response manner. The result demonstrated that nano-TiO2 has a potential negative effect on the algae's ability to absorb inorganic nitrogen.
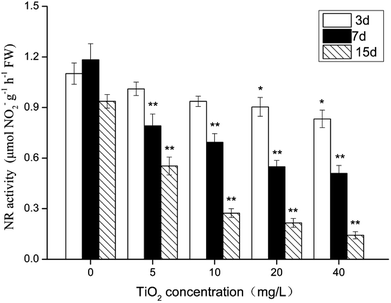 |
| Fig. 4 The effect of nano-TiO2 on nitrate reductase (NR) of G. lemaneiformis. Data are presented as the mean ± SD of three replicates. *P < 0.05, **P < 0.01. | |
Effects of nano-TiO2 on O2˙−
The influence of different nano-TiO2 concentrations on the O2˙− level in G. lemaneiformis is shown in Fig. 5. The O2˙− level in the TiO2-exposed groups increased by 1.1–2.1-fold when compared to the control group, indicating that nano-TiO2 did cause oxidative stress in G. lemaneiformis. After 3 and 7 days of cultivation, the level of O2˙− visibly increased with increasing nano-TiO2 concentrations. On the 15th day, the content of O2˙− reached a maximum with a 209.94% increase at 10 mg L−1 of TiO2, but there was a slight decrease in O2˙− content at 20 mg L−1 and 40 mg L−1 of TiO2.
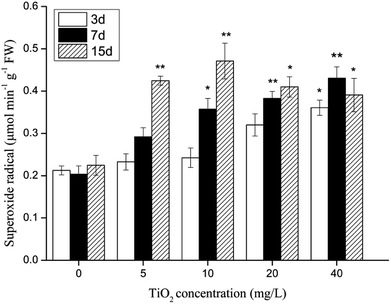 |
| Fig. 5 The effect of nano-TiO2 on superoxide radical of G. lemaneiformis. Data are presented as the mean ± SD of three replicates. *P < 0.05, **P < 0.01. | |
Effect of nano-TiO2 on the radical scavenging capacity of seaweeds
DPPH· radical scavenging. DPPH· radical scavenging activity has been widely used to screen antioxidants, such as polysaccharides, polyphones and water extracts from algae.41–43 The DPPH· radical scavenging capacity of AEGL was determined, and the results are shown in Fig. 6. The DPPH· radical scavenging activity in the groups exposed to nano-TiO2 was lower than in the control group. The highest DPPH· radical scavenging activity was measured in untreated G. lemaneiformis with an inhibition ratio of 47%. 15 days after exposure to nano-TiO2, the antioxidant capacities decreased in a dose–response manner. Compared to unprocessed seaweeds, the DPPH· radical scavenging activity decreased by 1.4–2.2 times for G. lemaneiformis (P < 0.05).
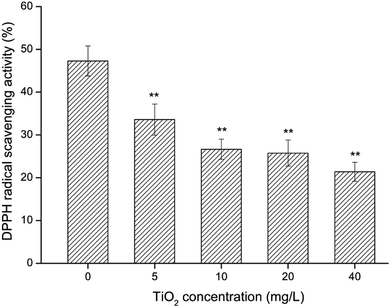 |
| Fig. 6 The DPPH· scavenging ability of AEGL when G. lemaneiformis was exposed to different concentrations of nano-TiO2 for 15 d. Data are presented as the mean ± SD of three replicates. *P < 0.05, **P < 0.01. | |
Hydroxyl radical scavenging activity. The ·OH scavenging activity of AEGL was investigated according to the mechanism of the Fenton reaction. To determine the effect of nano-TiO2 on the ·OH scavenging capacity of G. lemaneiformis, the ·OH scavenging activity in AEGL was examined (Fig. 7). Interestingly, G. lemaneiformis without exposure to nano-TiO2 showed greater radical scavenging activity, whereas the ·OH scavenging activity induced by AEGL decreased significantly with the increase in nano-TiO2 exposure in G. lemaneiformis. 15 days after exposure to nano-TiO2, the ·OH scavenging capacity of G. lemaneiformis decreased from 76% to 36%.
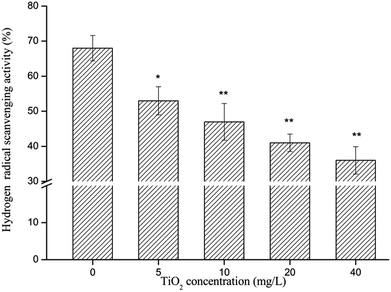 |
| Fig. 7 The hydroxyl radical scavenging ability of AEGL when G. lemaneiformis was exposed to different concentrations of nano-TiO2 for 15 d. Data are presented as the mean ± SD of three replicates. *P < 0.05, **P < 0.01. | |
Discussion
Recently, more attention has been paid to marine macroalgae because of their positive influence on nutrient cycling, energy transformation, and medicine.44–46 In the current study, the effect of nano-TiO2 on the growth rate, oxidative damage and radical scavenging activity of G. lemaneiformis was determined to provide a better understanding of nano-TiO2 toxicity on macroalgae species. This study demonstrated that nano-TiO2 may inhibit the growth of G. lemaneiformis and may decrease the antioxidant capacity of G. lemaneiformis.
In this study, G. lemaneiformis treated with nano-TiO2 showed a distinct decrease in RGRs (Fig. 2). Similar results were observed in K. brevis47 and Chlorella sp.;48 their results indicated that nano-TiO2 greatly inhibited the algal growth with EC50 of 10.69 mg L−1 and EC30 of 30 mg L−1, respectively. The G. lemaneiformis exposed to more than 10 mg L−1 of nano-TiO2 even showed visible, partial necrosis and depigmentation after 15 days in culture. The decrease in the growth rate of G. lemaneiformis was due to the mechanisms of avoidance, repair and protection in resistance to the stress caused by nano-TiO2. Similarly, Wang et al. reported that nano-TiO2 at concentrations ≥20 mg L−1 could significantly inhibit P. tricornutum growth.49 The SP content in seaweed is one of the important indexes to measure the metabolism of algae. Increasing its SP content helps the algae maintain normal cellular metabolism and improves the stress resistance of the seaweed. However, certain concentrations of pollutants alter patterns of protein synthesis, such as inducing nuclease and protease activity in algae, and then reduce the content of SP.50 In this experiment, the SP content slightly increased on the 3rd day at the low concentration of nano-TiO2; the reason for this increase was that G. lemaneiformis initiated a self-protection mechanism to maintain the normal metabolism of the algae cells by increasing the amount of functional proteins and the SP content. Nevertheless, when exposed to nano-TiO2 for 7 and 15 days, the content of SP decreased significantly, which is possibly due to cell damage reaching a critical level and to protein synthesis damage.
Nitrate reductase, a key enzyme in marine plant metabolism, acts as a catalyst for the reduction of NO3− to NO2−.51 In algae, NR is an inducible enzyme which may be affected by various environmental factors, such as light, metal ions and organic pollutants.28,52 The results in this study demonstrated that NR of G. lemaneiformis was affected by nano-TiO2 concentrations and by culture time. Under nano-TiO2 exposure, the NR activity of G. lemaneiformis was significantly inhibited. It is possible that the algae produced a greater number of ROS under the stress of nano-TiO2, which caused oxidative damage to NR. This phenomenon has also been reported in other literature.53
O2˙− is one of the major components of ROS. It is an inevitable product of algal metabolism. Nanoparticles are known to damage proteins in the DNA and PS II reaction centres,54 to reduce the photosynthetic capacity of algae,55 and even to induce the production of reactive oxygen species (ROS) in aquatic organisms.33 Under normal conditions, ROS content in algae remains low. However, when algae are stressed (e.g., UV-B stress, drought, salt stress, temperature stress and heavy metal stress), the metabolism of ROS in the algae will be inhibited, and the content of ROS will significantly increase. If the intracellular production of ROS is not removed in time, it will lead to an oxidative reaction, such as lipid peroxidation and destruction of the cell membrane. Shiu and Lee56 reported that an increase in ROS in algae was closely related to the extent of oxidative damage. The experimental results showed that with the increase in nano-TiO2 concentration and the extension of exposure time, the O2˙− content of G. lemaneiformis increased, indicating that the level of intracellular ROS in algae increased because of the toxic effects of nano-TiO2. The O2˙− level decreased visibly with the 20 or 40 mg L−1 nano-TiO2 exposure to algae for 15 days; it was probably due to the decay of algae which resulted in relevant enzymes inactive. Dubey and Prasad57 found that UV-B radiation stimulated an increase in the O2˙− content in algae, but excessive O2˙− could inhibit antioxidant enzyme activity or antioxidant synthesis in algae, leading to oxidative stress and resulting in cell toxicity. This is consistent with the results of our study.
Algae are a potential source of drug components in a variety of therapeutic areas.32 The active ingredients that scavenge free radicals in G. lemaneiformis may be polysaccharides, polyphenols, biological glycosides or phycoerythrin. Many studies have shown that these active ingredients in algae have antioxidant effects.58,59 Boonchum et al.41 reported that aqueous extracts of seaweeds showed high antioxidant activity. Considering the serious effect of nano-TiO2 toxicity on G. lemaneiformis, nano-TiO2 may reduce the antioxidant activity of AEGL. Therefore, the effects of nano-TiO2 on the antioxidant activity of AEGL were determined in this study.
The scavenging effect of free radicals was verified by the determination of DPPH· and ·OH scavenging tests. The results indicated that when the G. lemaneiformis was exposed to nano-TiO2, AEGL showed less efficiency in radical scavenging. The reason for this result may be that when the G. lemaneiformis was exposed to nano-TiO2, the level of antioxidant active substances in G. lemaneiformis decreased. In this experiment, the extract of G. lemaneiformis which were not exposed to nano-TiO2 showed a higher rate of scavenging effect on ·OH and DPPH·. Therefore, AEGL may be a potent antioxidant. It is important to maintain human health. Because of its strong antioxidant ability, G. lemaneiformis is expected to be a new marine drug resource.
Conclusion
The results in this study indicated that nano-TiO2 is toxic to G. lemaneiformis, which may result in the inhibition of growth and in oxidative damage. The growth of G. lemaneiformis declined dramatically when G. lemaneiformis was exposed to nano-TiO2. During the experimental period, both SP and NR contents in G. lemaneiformis were decreased by nano-TiO2 concentration and by culture time. After the G. lemaneiformis were exposed to nano-TiO2 for 3 and 7 days, the activities of O2˙− increased linearly as exposure concentration increased. On the 15th day, the O2˙− activities was increased first and then decreased. This showed that nano-TiO2 have oxidative damage to G. lemaneiformis. Furthermore, the aqueous extract of G. lemaneiformis has the potential to eliminate free radicals. However, the antioxidant ability of AEGL was significantly impaired after G. lemaneiformis was exposed to different concentrations of nano-TiO2 for 15 days. A decreased radical scavenging activity of AEGL were observed after G. lemaneiformis exposing to different concentrations of nano-TiO2 for 15 days, and which were linearly associated with nano-TiO2 exposure concentration. Experimental data, based on toxicity tests of nano-TiO2 in this research, may contribute to understanding the ecological effects of oxide nanoparticles.
Conflicts of interest
There are no conflicts to declare.
Acknowledgements
This work was supported by the NSFC-Guangdong Province joint key project (U1301235), Industry-university-research combination project of Guangdong Province (2013B090600009), National Natural Science Foundation of China (Grant No. 41676110) and the Science and Technology Program of Guangdong, China (2014A020217007).
References
- Q. Zhou, J. Zhang, J. Fu, J. Shi and G. Jiang, Anal. Chim. Acta, 2008, 606, 135 CrossRef PubMed.
- A. Neori, M. D. Krom, S. P. Ellner, C. E. Boyd, P. Dan, R. Rabinovitch, P. J. Davison, O. Dvir, D. Zuber and M. Ucko, Aquaculture, 1996, 141, 183–199 CrossRef.
- H. Yang, Y. Zhou, Y. Mao, X. Li, Y. Liu and F. Zhang, J. Appl. Phycol., 2005, 17, 199–206 CrossRef.
- J. K. Patra, S. K. Rath, K. B. Jena, V. K. Rathod and H. Thatoi, Turk. J. Biol., 2008, 119–125 Search PubMed.
- Z. Wang, X. Wang and C. Ke, J. Appl. Phycol., 2014, 26, 1889–1897 CrossRef.
- R. Dastjerdi and M. Montazer, Colloids Surf., B, 2010, 79, 5–18 CrossRef PubMed.
- A. Jaroenworaluck, W. Sunsaneeyametha, N. Kosachan and R. Stevens, Surf. Interface Anal., 2010, 38, 473–477 CrossRef.
- R. M. Wang, B. Y. Wang, Y. F. He, W. H. Lv and J. F. Wang, Polym. Adv. Technol., 2010, 21, 331–336 CrossRef.
- W. Zhang, Y. Chen, C. Chen, C. Chen and X. Liu, Mater. Rev., 2015, 16(49–54), 239–244 Search PubMed.
- C. O. Robichaud, A. E. Uyar, M. R. Darby, L. G. Zucker and M. R. Wiesner, Environ. Sci. Technol., 2009, 43, 4227–4233 CrossRef PubMed.
- J. Ji, Z. Long and D. Lin, Chem. Eng. J., 2011, 170, 525–530 CrossRef.
- B. D. Chithrani, A. A. Ghazani and W. C. W. Chan, Nano Lett., 2006, 6, 662–668 CrossRef PubMed.
- Y. S. Tian, F. Gottschalk, K. Hungerbühler and B. Nowack, Environ. Pollut., 2014, 185, 69 CrossRef PubMed.
- F. Gottschalk, T. Sonderer, R. W. Scholz and B. Nowack, Environ. Sci. Technol., 2009, 43, 9216 CrossRef PubMed.
- Y. Tian, Y. Zhao, H. Zeng, Y. Zhang and B. Zheng, Carbohydr. Polym., 2016, 154, 231–240 CrossRef PubMed.
- M. Sendra, I. Moreno-Garrido, M. P. Yeste, J. M. Gatica and J. Blasco, Environ. Pollut., 2017, 227, 39–48 CrossRef PubMed.
- E. Navarro, A. Baun, R. Behra, N. B. Hartmann, J. Filser, A. J. Miao, A. Quigg, P. H. Santschi and L. Sigg, Ecotoxicology, 2008, 17, 372–386 CrossRef PubMed.
- Z. Luo, Z. Wang, Y. Yan, J. Li, C. Yan and B. Xing, Environ. Pollut., 2018, 238, 631 CrossRef PubMed.
- P. Neale, A. Jamting, E. O'Malley, J. Herrmann and B. Escher, Environ. Sci.: Nano, 2015, 2, 86–93 RSC.
- Z. Clemente, V. L. Castro, C. M. Jonsson and L. F. Fraceto, Int. J. Environ. Res., 2012, 6, 33–50 Search PubMed.
- S. Azman, M. I. M. Said, F. Ahmad and M. Mohamad, Biomass Bioenergy, 2014, 66, 103–109 CrossRef.
- H. Wu, H. Jiang, C. Liu and Y. Deng, S. Afr. J. Bot., 2015, 100, 27–32 CrossRef.
- M. Ghosh, M. Bandyopadhyay and A. Mukherjee, Chemosphere, 2010, 81, 1253–1262 CrossRef PubMed.
- B. Xia, B. Chen, X. Sun, K. Qu, F. Ma and M. Du, Sci. Total Environ., 2015, 508, 525–533 CrossRef PubMed.
- C. Ye, H. Liao and Y. Yang, J. Sea Res., 2014, 90, 10–15 CrossRef.
- Z. X. Han, M. Zhang and C. X. Lv, Adv. Mater. Res., 2012, 518–523, 942–945 Search PubMed.
- M. L. Fernando, Z.-V. Leonardo, P. Colepicolo, M.-S. Eliane and S. C. Vinicius, J. Appl. Phycol., 2013, 25, 1939–1947 CrossRef.
- Z. Xu and K. Gao, Mar. Pollut. Bull., 2012, 64, 99–105 CrossRef PubMed.
- J. Xia, Y. Li, J. Lu and B. Chen, Bull. Environ. Contam. Toxicol., 2004, 73, 979–986 CrossRef PubMed.
- X. F. Zhu, D. H. Zou, J. B. Jian, W. Z. Chen, H. H. Liu and H. Du, Mar. Sci. Bull., 2009, 20, 1438–1444 Search PubMed.
- M. Y. Babu, L. Palanikumar, N. Nagarani, V. J. Devi, S. R. Kumar, C. M. Ramakritinan and A. K. Kumaraguru, Environ. Sci. Pollut. Res., 2014, 21, 9604–9616 CrossRef PubMed.
- P. Burtin, Electron. J. Environ., Agric. Food Chem., 2003, 2, 498–503 Search PubMed.
- F. Li, Z. Liang, X. Zheng, W. Zhao, M. Wu and Z. Wang, Aquat. Toxicol., 2015, 158, 1 CrossRef PubMed.
- A. M. Carroll and A. A. Biewener, Bot. Mar., 1986, 29, 147–154 Search PubMed.
- Y. Wang, Y. Feng, H. Wang, M. Zhong, W. Chen and H. Du, J. Appl. Phycol., 2016, 28, 1847–1858 CrossRef.
- A. Corzo and F. X. Niell, J. Exp. Mar. Biol. Ecol., 1991, 146, 181–191 CrossRef.
- G. Luo and A. Wang, Acta Phytophysiol. Sin., 1990, 16, 239–244 Search PubMed.
- K. Zandi, S. Tajbakhsh, I. Nabipour, Z. Rastian, F. Yousefi, S. Sharafian and K. Sartavi, Afr. J. Biotechnol., 2013, 9, 6787–6790 Search PubMed.
- G. C. Yen and H. Y. Chen, J. Agric. Food Chem., 1995, 43, 27–32 CrossRef.
- J. Ming, C. Yaxin, L. Jinrong and Z. Hui, Prog. Biochem. Biophys., 1996, 23, 553–555 Search PubMed.
- W. Boonchum, Y. Peerapornpisal, D. Kanjanapothi, J. Pekkoh, C. Pumas, U. Jamjai, D. Amornlerdpison, T. Noiraksar and P. Vacharapiyasophon, Int. J. Agric. Biol., 2011, 13, 95–99 Search PubMed.
- W. Tao, R. Jónsdóttir and G. Ólafsdóttir, Food Chem., 2009, 116, 240–248 CrossRef.
- S. Wu, M. Lu and S. Wang, Biotech, 2017, 7, 38 Search PubMed.
- O. Raize, Y. Argaman and S. Yannai, Biotechnol. Bioeng., 2004, 87, 451–458 CrossRef PubMed.
- X. Wu, Z. Tian, L. Hu, S. Huang and J. Cai, RSC Adv., 2017, 7, 32795–32805 RSC.
- J. I. Yang, C. C. Yeh, J. C. Lee, S. C. Yi, H. W. Huang, C. N. Tseng and H. W. Chang, Molecules, 2012, 17, 7241–7254 CrossRef PubMed.
- F. Li, Z. Liang, X. Zheng, W. Zhao, M. Wu and Z. Wang, Aquat. Toxicol., 2015, 158, 1–13 CrossRef PubMed.
- J. Ji, Z. Long and D. Lin, Chem. Eng. J., 2011, 170, 525–530 CrossRef.
- Y. Wang, X. Zhu, Y. Lao, X. Lv, Y. Tao, B. Huang, J. Wang, J. Zhou and Z. Cai, Sci. Total Environ., 2016, 565, 818–826 CrossRef PubMed.
- J. L. Pérez-Lloréns, E. Benítez, J. J. Vergara and J. A. Berges, Eur. J. Phycol., 2003, 38, 55–64 CrossRef.
- E. Young, P. Lavery, B. V. Elven, M. Dring and J. A. Berges, Mar. Ecol.: Prog. Ser., 2005, 288, 103–114 CrossRef.
- J. Yu and Y. F. Yang, J. Exp. Mar. Biol. Ecol., 2008, 367, 142–148 CrossRef.
- T. Han, S. H. Kang, J. S. Park, H. K. Lee and M. T. Brown, Aquat. Toxicol., 2008, 86, 176–184 CrossRef PubMed.
- M. Yooiam, R. Chaichana and T. Satapanajaru, Chem. Speciation Bioavailability, 2014, 26, 257–265 CrossRef.
- C. M. Lalau, R. A. Mohedano, É. C. Schmidt, Z. L. Bouzon, L. C. Ouriques, R. W. dos Santos, C. C. Da, D. S. Vicentini and W. G. Matias, Protoplasma, 2015, 252, 221 CrossRef PubMed.
- C. T. Shiu and T. M. Lee, J. Exp. Bot., 2005, 56, 2851–2865 CrossRef PubMed.
- G. Dubey and S. M. Prasad, Appl. Biochem. Biotechnol., 2015, 175, 2703–2728 CrossRef PubMed.
- H. H. Ammar, S. Lajili, R. B. Said, D. L. Cerf, A. Bouraoui and H. Majdoub, Daru, J. Pharm. Sci., 2015, 23, 1–8 CrossRef PubMed.
- N. Heffernan, N. P. Brunton, R. J. Fitzgerald and T. J. Smyth, Mar. Drugs, 2015, 13, 509–528 CrossRef PubMed.
Footnote |
† Ling Zhao and Pinghe Yin contributed to the work equally. |
|
This journal is © The Royal Society of Chemistry 2018 |
Click here to see how this site uses Cookies. View our privacy policy here.