DOI:
10.1039/C8RA06343E
(Paper)
RSC Adv., 2018,
8, 31382-31387
Room temperature ferromagnetism in metallic Ti1−xVxO2 thin films
Received
27th July 2018
, Accepted 1st September 2018
First published on 6th September 2018
Abstract
Transition metal doped TiO2 diluted magnetic semiconductors have attracted considerable interest due to their room temperature ferromagnetism. However, most TiO2 films are highly insulating, and thus the magnetic properties can not be controlled by tuning the carrier concentration. This will limit their application in controlling magnetization via electrical gating. Here, we deposit rutile Ti1−xVxO2 (x = 0.03 and 0.05) films with the thickness between 30 and 245 nm by the pulsed laser deposition technique, and observe an obvious room temperature ferromagnetic behavior in all films. The high resolution X-ray photoelectron spectroscopy results indicate that V substituting Ti4+ ions in the TiO2 lattice, with the +3 valence state having two unpaired d electrons, is responsible for the local spin. More importantly, the systemic investigations of transport properties for Ti1−xVxO2 films reveal that the films are n-type and have metallic conductivity with a carrier density of about 1020/cm3. Further studies suggest that the oxygen vacancies play a dual role of contributing to the metallic conductivity of the Ti1−xVxO2 films, and also providing the free electrons to mediate the long-range ferromagnetic coupling between two magnetic polarons. These findings may offer promise for gate-tunable ferromagnetism in future semiconductor spintronics.
Introduction
Diluted magnetic semiconductors (DMSs) have attracted enormous attention due to their potential applications in spintronic devices.1–3 To date, the III–V based DMSs, such as Mn doped GaAs, have been well studied, however, the low values of the Curie temperature (TC) hindered their application at room temperature.4,5 An important step forward in the field was the theoretical prediction by Dietl et al. of high temperature ferromagnetism in Mn doped ZnO.6 Subsequently, the Co doped TiO2 thin films with the anatase structure were reported to be ferromagnetic even above 400 K with a magnetic moment of 0.32 μB per Co atom.7 Since then, transition metal (TM) doped TiO2 DMS has attracted particular interest as TiO2 has many advantages, such as low cost, good dielectric properties and high chemical stability.8–12 However, due to the low solubility of TMs in TiO2, extrinsic effects, such as magnetic clusters and impurity phases, are often responsible for the observed ferromagnetism.13–15 Furthermore, many studies have focused on the effects of the methods and growth conditions on the structural and magnetic properties of TiO2 DMS,16–20 and it is also found that most TiO2 films doped with different transition elements are highly insulating.21–26 For example, Griffin et al.21 grew a series of anatase Co:TiO2 films by RF magnetron sputtering, and obtained a saturation magnetic moment of 1.1 μB/Co, while all films were highly insulating. Sharma et al.25 showed that the Mn-doped TiO2 films prepared by the spray pyrolysis technique also exhibited the highly insulating nature with the resistivity of almost 107 Ω cm. It is noted that although some reports demonstrate that the incorporation of nonmagnetic element Nb and Ta in TiO2 can lead to metallic electrical conduction,27–32 the origin of magnetic moments is attributed to cationic vacancies.31,32 A DMS, containing a dilute concentration of magnetic ions imbedded in the host lattices, is characterized by the free carriers mediated exchange interactions between the magnetic ions. In such systems, the magnetization can be controlled by tuning the carrier density via electrical gating. In order to meet this criterion, it is essential to obtain the conductive TiO2 films.
In this work, we obtain the metallically conductive Ti1−xVxO2 films with different thickness by using the pulsed laser deposition (PLD) technique with precise control of oxygen pressure at 3 × 10−3 mTorr. The structural, composition and magnetic results suggest that the observed room temperature ferromagnetism in Ti1−xVxO2 films is intrinsic. Further studies indicate that the oxygen vacancy not only contributes to the metallic conductivity of the Ti1−xVxO2 films, but also it provides the free electrons to mediate the long-range ferromagnetic coupling between two magnetic polarons.
Experimental method
The Ti1−xVxO2 films (x = 0.03 and 0.05) with the thickness of 30–245 nm were grown on SrTiO3 (100) substrate by the PLD technique at a temperature of 800 °C and an oxygen partial pressure of 3 × 10−3 mTorr. The laser pulses were supplied by a KrF excimer source (λ = 248 nm) with an energy density of 2.5 J per cm2 per shot and a frequency of 10 Hz. The nominal Ti1−xVxO2 targets were prepared by a solid-state reaction method using TiO2 (99.99%) and V6O13 (99.97%) powders, and they were ablated for 5 minutes to eliminate surface contamination before deposition. After deposition, the films were annealed in situ for 30 minutes, and then cooled down to room temperature slowly at the same oxygen pressure. The crystal structures of the films were analyzed by θ–2θ X-ray diffraction (XRD) with using Cu Kα radiation (λ = 0.15406 nm). The chemical composition was determined by X-ray photoelectron spectroscopy (XPS) with a monochromatic Al Kα radiation as the X-ray source. The magnetic properties were measured by a superconducting quantum interference device (SQUID) magnetometer. The transport properties of the films were determined in the four-point probe configuration using a Quantum Design physical properties measurement system (PPMS) as a function of temperature.
Results and discussion
Fig. 1 shows the XRD patterns of Ti1−xVxO2 films (x = 0.03 and 0.05) with the thickness of about 100 nm. Here, the XRD pattern for pure TiO2 film deposited as the same condition is also placed at the bottom of figure for comparison. The spectra are plotted on a logarithmic scale to discern any minor secondary phase with small intense reflections. The results show that the Ti1−xVxO2 films are epitaxial with single-phase rutile phase character, with only (200) and (400) reflections detectable. It is noted that the undoped TiO2 film is epitaxial and of anatase phase with the (00l) orientation. It has been proposed that two Ti–O bonds break in the anatase structure, allowing the rearrangement of the Ti–O octahedra, which leads to a smaller volume and the rutile phase.33 The breaking of these bonds is accelerated by the lattice disruptions, which can be introduced by the presence of dopant ions, the oxygen vacancies, and the method of synthesis.34 In our study, the Ti1−xVxO2 films were deposited at a high vacuum (3 × 10−3 mTorr), resulting in a large amount of oxygen vacancies in the films. This will presumably reduce the strain energy that must be overcome before the rearrangement of the Ti–O octahedral can occur,33 and thus promotes the phases transformation.
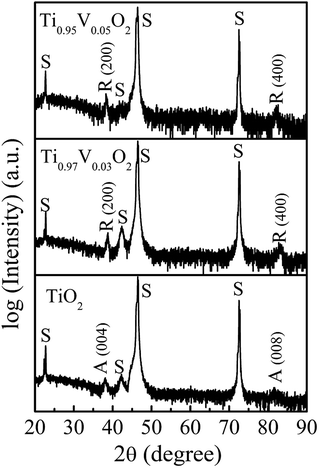 |
| Fig. 1 The XRD patterns of Ti1−xVxO2 films. The peaks marked with “S”, “A” and “R” correspond to the peaks of SrTiO3 substrates, anatase TiO2 and rutile TiO2, respectively. | |
The Ti1−xVxO2 films with different thickness exhibit the obvious room temperature ferromagnetism. The in-plane room temperature magnetic hysteresis (M − H) loops of the films are shown in Fig. 2(a) and (b). The diamagnetic contribution from the substrate is subtracted from the loops. The insets show the magnified M − H loops of the films. The saturation magnetizations (Ms) of the Ti0.97V0.03O2 films with thickness of 30, 50, 135 and 240 nm are 1.9, 1.2, 0.4 and 0.1 μB/V, and their coercive field (Hc) are 165, 110, 75, and 30 Oe, respectively. For the Ti0.95V0.05O2 films, the maximum values of Ms and Hc obtained in the thinner films are 0.5 μB/V and 120 Oe, respectively. It clearly shows the reduction in Ms and Hc with increasing thickness. The observation of the larger Ms value in thinner film could be attributed to more structural or surface defects in the films.35 For comparison of Fig. 2(a) and (b), it can be found that the values of Ms for Ti0.95V0.05O2 films are smaller than those of Ti0.97V0.03O2 films at the same thickness. This could be assigned to an increase in the antiferromagnetic coupling between V ions at shorter separations.36 Fig. 2(c) and (d) display the zero-field cooled (ZFC) and field-cooled (FC) magnetization curves at a field of 100 Oe for the Ti1−xVxO2 films. There is no evidence of the blocking temperature in the whole temperature range of 10–300 K, suggesting that the tiny ferromagnetic nano-clusters are not present in the films.37,38 Moreover, the ZFC/FC curves are distinctly separated from each other without any phase transition from 10 to 300 K, indicating that the TC of the Ti1−xVxO2 films is higher than 300 K.
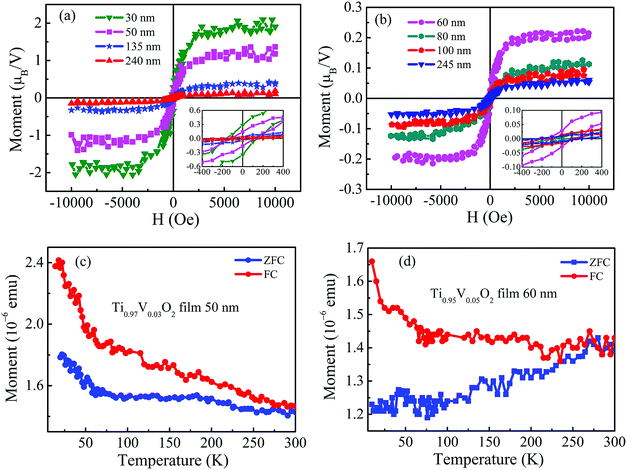 |
| Fig. 2 Room temperature M − H loops of Ti0.97V0.03O2 (a) and Ti0.95V0.05O2 (b) films, respectively, with different thickness. The insets show the magnified M − H loops of the films. (c) and (d) The ZFC/FC curves of the Ti0.97V0.03O2 and Ti0.95V0.05O2 films with thickness of 50 and 60 nm, respectively. | |
The four-point probe geometry was used to obtain the transport properties of the Ti1−xVxO2 films. The results indicate that all of the films show n-type conductivity and the carrier concentration is about 1020/cm3. The temperature (T) dependence of resistivity (ρ) is measured down to 10 K, which is shown in Fig. 3. All the resistivity versus temperature curves show positive slope, indicating an metallic conductivity, and the resistivity slightly increases as the films thickness increases. Hong et al.39 deposited the V-doped TiO2 films on LaAlO3 substrates by the PLD method, and they found that the films were semiconductors and the resistivity at room temperature was as high as 107 Ω cm, which is very different from our films. This may be due to the influence of preparation or processing conditions and the resulting defects on the transport properties of V–TiO2 films. Osorio-Guillén et al.40 studied theoretically the electronic behaviors in V doped anatase TiO2, and showed that VTi introduced deep levels in the gap due to the low 3d energy of the V atoms, resulting in the nonconductive for V-doped TiO2.
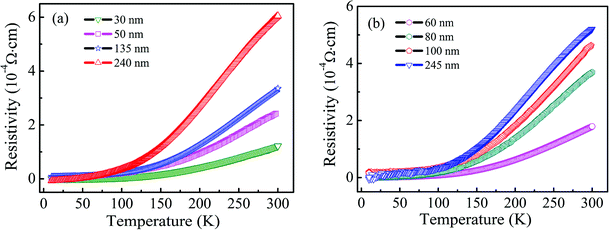 |
| Fig. 3 The temperature dependent resistivity as a function of thickness of Ti0.97V0.03O2 (a) and Ti0.95V0.05O2 (b) films. | |
Now, the possible origins of room temperature ferromagnetism and metallic behavior in the Ti1−xVxO2 films will be explored. If some portion of V is in the +3 or +4 valence states, or Ti is in the +3 valence state, and then the V3+, V4+ or Ti3+ will act as a localized spin, which is prerequisite to induce the magnetic ordering.41 The n-type donors of V5+ and oxygen vacancies may contribute to the metallic conductivity of the films. In order to examine these possibilities, the XPS measurement was performed to determine the chemical states of Ti, O and V in the Ti1−xVxO2 films. Fig. 4(a) shows the XPS survey spectra of Ti0.95V0.05O2 film with the thickness of 180 nm. No additional peaks corresponding to secondary phases are detected, which is in accordance with the XRD and ZFC/FC measurements. Fig. 4(b) shows the Ti 2p spectrum for the same sample with Ti 2p3/2 and Ti 2p1/2 located at 458.5 and 464.3 eV, respectively, suggesting that Ti is in the +4 state.25,42 The peak separation between the 2p3/2 and 2p1/2 lines is 5.8 eV, which is also consistent with the Ti4+ oxidation state.43,44 The binding energies of V 2p3/2 and V 2p1/2 shown in Fig. 4(c) are 515.2 and 523.4 eV, respectively, indicating that V is in the +3 state.45,46 Fig. 4(d) displays the spectra of O1s, which are divided into two peaks, referred to as O1 and O2. The peaks near 530.2 and 531.9 eV correspond to the binding energy of lattice oxygen in TiO2 and oxygen defects, respectively.47 It can be seen that an amount of oxygen vacancies exists in the Ti0.95V0.05O2 film, which can be ascribed to the films deposited at a very low deposition oxygen pressure (3 × 10−3 mTorr). Additionally, the substitution of Ti4+ by V3+ ions will also increase the concentration of oxygen vacancies due to the necessity for the charge balance. Based on the XPS results, it is reasonable to claim that in the Ti1−xVxO2 films the V3+ ions provide the local magnetic moment and the metallic conductivity can be attributed to the ionized donors of oxygen vacancies. This result is consistent with the theoretical calculations by Osorio-Guillén et al. that V dopants could convert nonmagnetic TiO2 into a ferromagnet as VTi can introduce a partially occupied, spin-polarized level, which could promote ferromagnetism.40
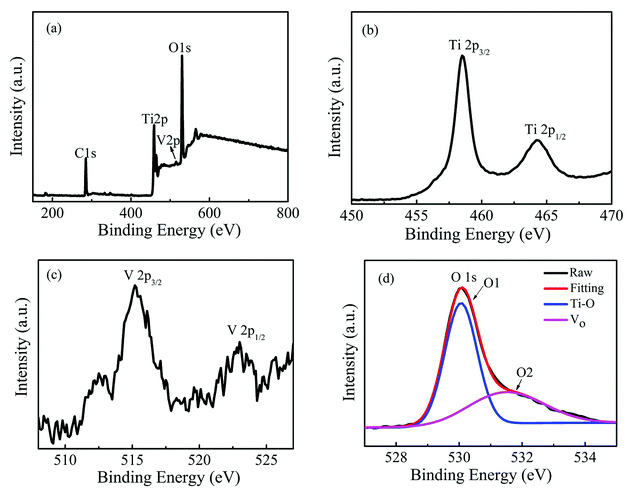 |
| Fig. 4 XPS spectra of Ti0.95V0.05O2 film with the thickness of 180 nm: the survey spectra (a) and high-resolved spectra of Ti 2p (b), V 2p (c) and O 1s (d), respectively. | |
There are different mechanisms for ferromagnetic coupling in the literature for TiO2-based DMS, such as carrier-mediated exchange48 and bound magnetic polaron (BMP) model.49 Tian et al.36 speculated that the ferromagnetic coupling between V ions mediated by oxygen vacancies at interfaces may account for the observed room temperature ferromagnetism in V-doped TiO2 nanoparticles. Hong et al.50 reported the large value of magnetic moment of 4.2 μB/V for the V:TiO2 films and suggested that the room temperature ferromagnetism did not come from V clusters but from V-doped TiO2 matrix. Du et al.51 used a first principles to study the magnetic properties of anatase Ti1−xVxO2, and showed that the oxygen vacancy induced magnetic polaron could produce long-range ferromagnetic interaction between largely separated V impurities. In the present work, V is chosen as a dopant because it is impossible to form any ferromagnetic secondary phase of V metal and V oxide, ruling out the extrinsic origin of the ferromagnetism. Indeed, the XRD, XPS and ZFC/FC results suggest that the observed room temperature ferromagnetism in Ti1−xVxO2 films is intrinsic. Moreover, there are amount of oxygen vacancies in the Ti1−xVxO2 films, and the films exhibit the metallic behavior (Fig. 3) with the high carrier concentration of 1020/cm3. In this regard, we propose that the doped V3+ ions ferromagnetically couple with the electrons trapped by the oxygen vacancies, and form the BMPs, the carriers mediated the long-range ferromagnetic coupling between the magnetic polarons is a more possible mechanism in Ti1−xVxO2 films. This is in agreement with our previous theoretical results.52
Conclusions
In summary, we have prepared rutile Ti1−xVxO2 (x = 0.03 and 0.05) films with different thickness by using the pulsed laser deposition technique, and observed ferromagnetism at room temperature. The structural, composition and magnetic results suggested that the room temperature ferromagnetism in Ti1−xVxO2 films was intrinsic. More importantly, the Ti1−xVxO2 films showed n-type and metallic conductivity. Further studies indicate that the oxygen vacancy not only contributes to the metallic conductivity of the Ti1−xVxO2 films, but also it provides the free electrons to mediate the long-range ferromagnetic coupling between two magnetic polarons.
Conflicts of interest
There are no conflicts to declare.
Acknowledgements
This work was supported by the National Key R&D Program of China (No. 2017YFB0405703), and National Natural Science Foundation of China (No. 61434002).
Notes and references
- S. A. Wolf, D. D. Awschalom, R. A. Buhrman, J. M. Daughton, S. von Molnár, M. L. Roukes, A. Y. Chtchelkanova and D. M. Treger, Science, 2001, 294, 1488 CrossRef PubMed.
- T. Dietl, Nat. Mater., 2010, 9, 965 CrossRef PubMed.
- S. Ogale, D. Kundaliya, S. Mehraeen, L. F. Fu, S. X. Zhang, A. Lussier, J. Dvorak, N. D. Browing, Y. Idzerda and T. Venkatesan, Chem. Mater., 2008, 20, 1344 CrossRef.
- L. Chen, X. Yang, F. H. Yang, J. H. Zhao, J. Misuraca, P. Xiong and S. Von Molnár, Nano Lett., 2011, 11, 2584 CrossRef PubMed.
- L. Chen, S. Yan, P. F. Xu, J. Lu, W. Z. Wang, J. J. Deng, X. Qian, Y. Ji and J. H. Zhao, Appl. Phys. Lett., 2009, 95, 182505 CrossRef.
- T. Dietl, H. Ohno, F. Matsukura, J. Cibert and D. Ferrand, Science, 2000, 287, 1019 CrossRef PubMed.
- Y. Matsumoto, M. Murakami, T. Shono, T. Hasegawa, T. Fukumura, M. Kawasaki, P. Ahmet, T. Chikyow, S. Koshihara and H. Koinuma, Science, 2001, 291, 854 CrossRef PubMed.
- S. Duhalde, M. F. Vignolo, F. Golmar, C. Chiliotte, C. E. Rodríguez Torres, L. A. Errico, A. F. Cabrera and M. Rentería, Phys. Rev. B, 2005, 72, 161313 CrossRef.
- J. Chen, P. Rulis, L. Quyang, S. Satpathy and W. Y. Ching, Phys. Rev. B, 2006, 74, 235207 CrossRef.
- Y. J. Lee, M. P. de Jong and W. G. van der Wiel, Phys. Rev. B, 2011, 83, 134404 CrossRef.
- B. Choudhury, R. Verma and A. Choudhury, RSC Adv., 2014, 4, 29314 RSC.
- A. Chanda, K. Rout, M. Vasundhara, S. R. Joshi and J. Singh, RSC Adv., 2018, 8, 10939 RSC.
- J. Y. Kim, J. H. Park, B. G. Park, H. J. Noh, S. J. Oh, J. S. Yang, D. H. Kim, S. D. Bu, T. W. Noh, H. J. Lin, H. H. Hsieh and C. T. Chen, Phys. Rev. Lett., 2003, 90, 017401 CrossRef PubMed.
- S. R. Shinde, S. B. Ogale, J. S. Higgins, H. Zheng, A. J. Millis, V. N. Kulkarni, R. Ramesh, R. L. Greene and T. Venkatesan, Phys. Rev. Lett., 2004, 92, 166601 CrossRef PubMed.
- A. I. Rykov, K. Nomura, J. Sakuma, C. Barrero, Y. Yoda and T. Mitsui, Phys. Rev. B, 2008, 77, 014302 CrossRef.
- T. C. Kaspar, S. M. Heald, C. M. Wang, J. D. Bryan, T. Droubay, V. Shutthanandan, S. Thevuthasan, D. E. McCready, A. J. Kellock, D. R. Gamelin and S. A. Chambers, Phys. Rev. Lett., 2005, 95, 217203 CrossRef PubMed.
- S. Duhalde, M. F. Vignolo, F. Golmar, C. Chiliotte, C. E. Rodríguez Torres, L. A. Errico, A. F. Cabrera, M. Rentería, F. H. Sánchez and M. Weissmann, Phys. Rev. B, 2005, 72, 161313(R) CrossRef.
- D. L. Hou, R. B. Zhao, H. J. Meng, L. Y. Jia, X. J. Ye, H. J. Zhou and X. L. Li, Thin Solid Films, 2008, 516, 3223 CrossRef.
- S. A. Ahmed, J. Magn. Magn. Mater., 2016, 402, 178 CrossRef.
- R. N. Aljawfi, A. Vij, K. H. Chae, S. Dalela, P. A. Alvi, M. A. Al-Maghrabi and S. Kumar, Appl. Surf. Sci., 2018, 445, 287 CrossRef.
- K. A. Griffin, A. B. Pakhomov, C. M. Wang, S. M. Heald and K. M. Krishnan, Phys. Rev. Lett., 2005, 94, 157204 CrossRef PubMed.
- T. Zhao, S. R. Shinde, S. B. Ogale, H. Zheng, T. Venkatesan, R. Ramesh and S. D. Sarma, Phys. Rev. Lett., 2005, 94, 126601 CrossRef PubMed.
- T. Droubay, S. M. Heald, V. Shutthanandan, S. Thevuthasan, S. A. Chambers and J. Osterwalder, J. Appl. Phys., 2005, 97, 046103 CrossRef.
- K. G. Roberts, M. Varela, S. Rashkeev, S. T. Pantelides, S. J. Pennycook and K. M. Krishnan, Phys. Rev. B, 2008, 78, 014409 CrossRef.
- S. Sharma, S. Chaudhary, S. C. Kashyap and S. K. Sharma, J. Appl. Phys., 2011, 109, 083905 CrossRef.
- L. M. Xu, Y. P. Yu, X. J. Xing, X. Y. Wu and S. W. Li, Appl. Phys. A, 2008, 92, 361 CrossRef.
- Y. Furubayashi, T. Hitosugi, Y. Yamamoto, K. Inaba, G. Kinoda, Y. Hirose, T. Shimada and T. Hasegawa, Appl. Phys. Lett., 2005, 86, 252101 CrossRef.
- C. Tasaki, N. Oka, T. Yagi, N. Taketoshi, T. Baba, T. Kamiyama, S. Nakamura and Y. Shigesato, Jpn. J. Appl. Phys., 2012, 51, 035802 Search PubMed.
- J. Y. Yang, Y. L. Han, L. He, R. F. Dou, C. M. Xiong and J. C. Nie, Appl. Phys. Lett., 2012, 100, 202409 CrossRef.
- J. Suh, T. Sarkar, H. S. Choe, J. Park, T. Venkatesan and J. Wu, Appl. Phys. Lett., 2018, 113, 022103 CrossRef.
- S. Zhang, S. B. Ogale, W. Yu, X. Gao, T. Liu, S. Ghosh, G. P. Das, A. T. S. Wee, R. L. Greene and T. Venkatesan, Adv. Mater., 2009, 21, 2282 CrossRef.
- T. P. Sarkar, K. Gopinadhan, M. Motapothula, S. Saha, Z. Huang, S. Dhar, A. Patra, W. M. Lu, F. Telesio, I. Pallecchi, Ariando, D. Marré and T. Venkatesan, Sci. Rep., 2015, 5, 13011 CrossRef PubMed.
- R. D. Shannon and J. A. Pask, J. Am. Ceram. Soc., 1965, 48, 391 CrossRef.
- F. C. Gennari and D. M. Pasquevich, J. Mater. Sci., 1998, 33, 1571 CrossRef.
- X. F. Liu and R. H. Yu, J. Appl. Phys., 2007, 102, 083917 CrossRef.
- Z. M. Tian, S. L. Yuan, S. Y. Yin, S. Q. Zhang, H. Y. Xie, J. H. Miao, Y. Q. Wang, J. H. He and J. Q. Li, J. Magn. Magn. Mater., 2008, 320, L5 CrossRef.
- X. L. Yang, Z. T. Chen, C. D. Wang, Y. Zhang, X. D. Pei, Z. J. Yang, G. Y. Zhang, Z. B. Ding, K. Wang and S. D. Yao, J. Appl. Phys., 2009, 105, 053910 CrossRef.
- V. Fernandes, J. J. Klein, N. Mattoso, D. H. Mosca, E. Silveira, E. Ribeior, W. H. Schreiner, J. Varalda and A. J. A. de Oliverira, Phys. Rev. B, 2007, 75, 121304 CrossRef.
- N. H. Hong, J. Sakai and A. Hassini, Appl. Phys. Lett., 2004, 84, 2602 CrossRef.
- J. Osorio-Guillén, S. Lany and A. Zunger, Phys. Rev. Lett., 2008, 100, 036601 CrossRef PubMed.
- F. X. Jiang, X. H. Xu, J. Zhang, X. C. Fan, H. S. Wu and G. A. Gehring, Appl. Phys. Lett., 2010, 96, 052503 CrossRef.
- D. Gonbeau, C. Guimon, G. P. Guillouzo, A. Levasseur, G. Meunier and R. Dormoy, Surf. Sci., 1991, 254, 81 CrossRef.
- H. Li, W. Shi, W. Huang, E. P. Yao, J. Han, Z. Chen, S. Liu, Y. Shen, M. Wang and Y. Yang, Nano Lett., 2017, 17, 2328 CrossRef PubMed.
- M. E. Kurtoglu, T. Longenbach, K. Sohlberg and Y. Gogotsi, J. Phys. Chem. C, 2011, 115, 17392 CrossRef.
- H. Yoon, M. Choi, T. W. Lim, H. Kwon, K. Ihm, J. K. Kim, S. Y. Choi and J. Son, Nat. Mater., 2016, 15, 1113 CrossRef PubMed.
- G. Kim, J. Yoon, H. Yang, H. Lim, H. Lee, C. Jeong, H. Yun, B. Jeong, E. Crumlin, J. Lee, J. Lee, H. Ju and B. S. Mun, J. Appl. Phys., 2016, 120, 205305 CrossRef.
- L. T. Tseng, X. Luo, N. Bao, J. Ding, S. Li and J. Yi, Mater. Lett., 2016, 170, 142 CrossRef.
- Y. Yamada, K. Ueno, T. Fukumura, H. T. Yuan, H. Shimotani, Y. Iwasa, L. Gu, S. Tsukimoto and Y. Ikuhara, Science, 2011, 332, 1065 CrossRef PubMed.
- S. Kumar, J. S. Park, D. J. Kim, M. H. Lee, T. K. Song, S. Gautam, K. H. Chae, S. S. Kim and M. H. Kim, Ceram. Int., 2015, 41, S370 CrossRef.
- N. H. Hong, J. Sakai and W. Prellier, Phys. Rev. B, 2004, 70, 195204 CrossRef.
- X. Du, Q. Li, H. Su and J. Yang, Phys. Rev. B, 2006, 74, 233201 CrossRef.
- S. F. Qi, F. X. Jiang, J. P. Fan, H. Wu, S. B. Zhang, G. A. Gehring, Z. Y. Zhang and X. H. Xu, Phys. Rev. B, 2011, 84, 205204 CrossRef.
|
This journal is © The Royal Society of Chemistry 2018 |
Click here to see how this site uses Cookies. View our privacy policy here.