DOI:
10.1039/C8RA06806B
(Paper)
RSC Adv., 2018,
8, 33516-33522
Facile synthesis of novel porous self-assembling hydrogen-bonding covalent organic polymers and their applications towards fluoroquinolone antibiotics adsorption†
Received
14th August 2018
, Accepted 24th September 2018
First published on 1st October 2018
Abstract
A series of porous hydrogen-bonding covalent organic polymers (HCOPs) have been synthesized based on three-composite building blocks through a quick and succinct method for fluoroquinolone antibiotics adsorption from aqueous solutions. The porous properties of the HCOPs were regulated and controlled by adjusting the lengths of linkers, and the crystallinity and stability were strengthened due to the introduction of hydrogen bonds in HCOPs. Taking advantage of the porous properties and π-conjugated phenyl rings, as well as functional –CO–NH– and –COOH groups, HCOPs removed organic pollutants from wastewater effectively and showed good reusability. The external adsorption behavior was analyzed using both kinetic analysis and isotherm analysis. The results showed that the adsorption obeys the pseudo-second order kinetic model and follows the Langmuir isotherm model. The obtained maximum adsorption capacity of the four HCOPs was arranged in sequence according to the specific surface areas and pore sizes. Furthermore, the internal mechanisms involving perforated porousness, electrostatic interaction, hydrophobic interaction, π–π electron-donor–acceptor (EDA) interaction and hydrogen bonding formation, were investigated in detail. We envisage broadly applying the HCOPs in the facile and effective management of environmental pollution.
Introduction
Fluoroquinolones, used in pharmaceutical and personal care products (PPCPs), are synthetic antibiotics widely used in human medical and animal husbandry.1 With the improvement of human living standards, such antibiotics are extensively produced and used, and most of them tend to drain into the aquatic environment. Moreover, even a low concentration of antibiotics, will lead to the drug resistance of pathogenic bacteria and pose a serious threat to natural ecosystems and human health. In recent years, different degrees of such antibiotic pollution have been detected in various water environments. For example, in the United States, the concentrations of fluoroquinolone were detected to be 2 and 0.12 μg L−1 in municipal wastewater and surface water, respectively;2 and in the sewage from German hospitals, the concentration of fluoroquinolone was as high as 124. 5 μg L−1.3 Xu et al. investigated the average concentrations of fluoroquinolone in the mainstream of the Yellow River, 25 to 152 ng L−1, whereas the concentrations in the tributaries can reach up to 44 to 240 ng L−1.4 Therefore, it counts for a great deal to discuss the removal of fluoroquinolones from water. In many antibiotic wastewater treatment technologies, adsorption technology distinguishes itself from others with simple operation, little equipment investment cost and energy consumption, and no production of toxic intermediates.5 Considering the defective performance of existing adsorbents, developing new types of adsorbents comes to be burning issues in remediation of wastewater.
On the other hand, covalent organic polymers (COPs) consisted of covalent linkages, including both crystalline and amorphous forms, have been definitely showcased their striking charm across environment domains, healthcare sectors and energy-related fields.6 In general, the synthesis of COPs have been realized through employing finite one or two types of monomers to form limited linkages including B–O linkages, C–N linkages, C–C linkages, N–N linkages, etc. for constructing 2D/3D motifs.7 Apparently, although the progress achieved to what it is today, the restricted species and structure-types of COPs associated with the monotonous building blocks and special bonding modes, making the unsatisfactory full pay of COP values. In this way, exploring novel COPs with multiple linkages and versatile structures is an unquestionable necessity rather than just an option to meet the requirements of promoting application.8
Alternatively, hydrogen-bonded organic frameworks (HOFs) which rely on weak interactions e.g., van der Waals force, hydrogen bonding, π–π stacking and so forth, gradually emerge as a new highly innovative subject ever since first proposed by Chen's Group in 2011.9 However, the weaknesses of weak stability of HOFs outweigh the strengths of mild synthetic conditions. As a result, the practical applications of HOFs in environmental settings have been obstructed. Inspired by the pioneer work well established by Lin et al., a similar concept could be generated to develop hydrogen-bonded covalent organic polymers (HCOPs), which would leverage merits of both COPs and HOFs.10
To evidence this assumption, a series of porous hydrogen-bonding covalent organic polymers (HCOPs) were obtained by self-assembly of multiple types of mixed linkers through catalyst-free dynamic imine chemistry and hydrogen bonding within an extremely short time (Scheme 1). The strategy for preparing novel HCOPs was designed reasonably: (1) three types of monomers involving hydrazines, aldehydes and carboxylics were chosen as building blocks, bringing new features to the existing porous organic materials; (2) the C3-symmetric benzene-1,3,5-tricarbohydrazide (BTCH) containing carbohydrazide functional groups, not only could form hydrogen bonds with carboxylic monomers, but also could construct hydrazone linkages with aldehyde monomers; (3) by adjusting the length of linear monomers providing great potential extensibility and diversity of packing patterns; and moreover, (4) the introduction of hydrogen bonding coupled with covalent bonds affording high chemical stability towards selective adsorbing environmental pollutants. Herein, the resulting HCOPs were explored as a new type of adsorbents for two typical kinds of fluoroquinolone, i.e., ciprofloxacin (CIP) and norfloxacin (NOR) (Scheme 2). Notably, as far as we know, it was the first-time use of HCOPs for adsorbing fluoroquinolone antibiotics to address the environmental issues.
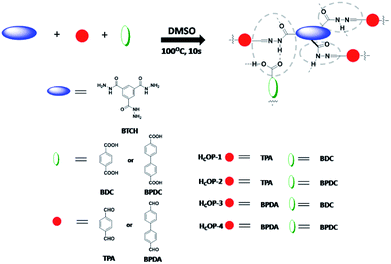 |
| Scheme 1 Representative molecular structures of HCOPs. | |
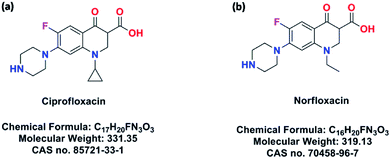 |
| Scheme 2 Chemical information of ciprofloxacin (a) and norfloxacin (b). | |
Experimental
Reagents and materials
All starting reagents, except benzene-1,3,5-tricarbohydrazide,7e were purchased commercially and used directly as received without further purification.
Characterization
The thermogravimetric analyses (TGA) were performed by heating the HCOPs at a heating rate of 10 °C min−1 in N2 flow. CHN elemental chemical analyses of the HCOPs were carried out by an Elementar model Vario Micro analyzer. The fourier transform infrared spectra (FT-IR) of the HCOPs were recorded by using a Nicolet Impact 410 Fourier transform infrared spectrometer through a KBr disc method in a range of 400–4000 cm−1. The surface areas and porosities of the HCOPs were measured on a Micromeritics ASAP 2020 analyzer. The powder X-ray diffraction (PXRD) patterns of the HCOPs were conducted by a Riguku D/MAX2550 diffractometer using CuKα radiation with a wavelength of 1.54178 Å. The morphologies and structures of the HCOPs were probed by field-scanning electron microscopy (FE-SEM, JEOLJXA-840, 15 kV) and the solid-state 13C cross-polarization/magic-angle spinning nuclear magnetic resonance (CP/MAS NMR, Bruker AVANCE III NMR spectrometer, 400 MHz). The UV-vis diffuse reflectance of the HCOPs spectra were recorded by a UV-vis spectrophotometer (UV-2550, Shimadzu) at room temperature. Point zero charge of the HCOPs were measured at various pH with a JS94H (Shanghai, China).
Synthesis of HCOP-1
A mixture of benzene-1,3,5-tricarbohydrazide (BTCH, 0.2 mmol, 0.05 g), terephthalaldehyde (TPA, 0.3 mmol, 0.04 g) and terephthalic acid (BDC, 0.3 mmol, 0.05 g) in dimethyl sulphoxide (DMSO, 10 mL) was stirred and heated at 100 °C for about 10 s. After that, the resulting yellow polymer (HCOP-1) could be achieved with 94% yield. Elemental analysis (wt%) calcd. For {C28H21N6O7}n: C 60.76, H 3.82, N 15.18; found: C 60.55, H 3.97, N 15.74.
Synthesis of HCOP-2
A mixture of benzene-1,3,5-tricarbohydrazide (BTCH, 0.2 mmol, 0.05 g), terephthalaldehyde (TPA, 0.3 mmol, 0.04 g) and biphenyl-4,4′-dicarboxylic acid (BPDC, 0.3 mmol, 0.07 g) in dimethyl sulphoxide (DMSO, 10 mL) was stirred and heated at 100 °C for about 10 s. After that, the resulting yellow polymer (HCOP-2) could be achieved with 95% yield. Elemental analysis (wt%) calcd. For {C34H25N6O7}n: C 64.86, H 4.00, N 13.35; found: C 64.55, H 4.16, N 13.74.
Synthesis of HCOP-3
A mixture of benzene-1,3,5-tricarbohydrazide (BTCH, 0.2 mmol, 0.05 g), 4,4′-biphenyldicarboxaldehyde (BPDA, 0.3 mmol, 0.06 g) and terephthalic acid (BDC, 0.3 mmol, 0.05 g) in dimethyl sulphoxide (DMSO, 10 mL) was stirred and heated at 100 °C for about 10 s. After that, the resulting yellow polymer (HCOP-3) could be achieved with 90% yield. Elemental analysis (wt%) calcd. For {C34H25N6O7}n: C 64.86, H 4.00, N 13.35; found: C 64.85, H 4.07, N 13.39.
Synthesis of HCOP-4
A mixture of benzene-1,3,5-tricarbohydrazide (BTCH, 0.2 mmol, 0.05 g), 4,4′-biphenyldicarboxaldehyde (BPDA, 0.3 mmol, 0.06 g) and biphenyl-4,4′-dicarboxylic acid (BPDC, 0.3 mmol, 0.07 g) in dimethyl sulphoxide (DMSO, 10 mL) was stirred and heated at 100 °C for about 10 s. After that, the resulting yellow polymer (HCOP-4) could be achieved with 92% yield. Elemental analysis (wt%) calcd. For {C40H29N6O7}n: C 68.08, H 4.14, N 11.91; found: C 67.95, H 4.16, N 12.04.
Adsorption experiments
All adsorption experiments were carried out with batch experiments at ambient temperature in air. In a general procedure, 10 mg HCOPs were added into 10 mL solution of fluoroquinolones (2–20 mg L−1). Thereafter, the mixture was stirred on a rotating shaker for a certain time at ambient temperature. Then the supernatant was measured by UV-vis spectrometry at wavelength of 277 nm for ciprofloxacin and 278 nm for norfloxacin at various time intervals to calculate the residue amount of fluoroquinolones in the solution.
Results and discussion
Characterization of HCOPs
Scanning electron microscopy (SEM) images showed that HCOP-1 and HCOP-2 appeared nanoporous interconnected particles, while HCOP-3 and HCOP-4 exhibited uniform rectangular morphologies, respectively (Fig. 1a–d). The PXRD patterns of the HCOPs were in line with the amorphous nature (Fig. S1, ESI†). Interestingly, the sharp peaks were observed in HCOPs, corresponding to the hydrogen bonds which could strengthen the crystallinity.11a The stability of HCOPs was studied in the presence of concentrated HCl. To our surprise, the HCOPs didn't dissolve and the PXRD patterns changed little even after 3 days (Fig. S2, ESI†). As we know, the hydrazone linkage is dynamic and acid-sensitive, we suggest that the high stability of HCOPs possibly results from the hydrogen bonds formed between the BTCH moieties and BDC (or BPDC) moieties in HCOPs.11b
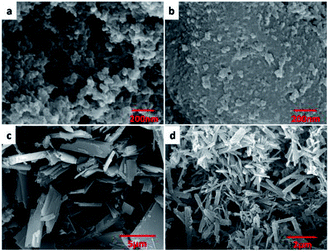 |
| Fig. 1 SEM images of HCOP-1 (a), HCOP-2 (b), HCOP-3 (c) and HCOP-4 (d). | |
The FT-IR spectra of HCOPs showed stretching peaks at 1288 cm−1 that are characteristic of C
N moieties, implying the occurrence of aldehyde–hydrazine condensation reaction in the HCOPs (Fig. S3 and S4, ESI†). Furthermore, when comparing the FT-IR spectra of HCOPs to monomers, we found that the stretching vibration of N–H at 3300 cm−1 in BTCH blue-shifted towards higher wavelength of 3450 cm−1; meanwhile, the C
O stretching band in BDC (or BPDC) red-shifted from 1685 to 1680 cm−1. These shifts can be ascribed to hydrogen-bonding interactions between carbonyl and imide.12a The successful formation of hydrogen bonds and hydrazone bonds in HCOPs were also proved by the 13C CP/MAS NMR analysis at the molecular level. As shown in Fig. 2, the characteristic resonances at 170 ppm and 160 ppm of C
O bonds provided the solid evidence for the presence of BDC (or BPDC) and BTCH, respectively. Meantime, the characteristic resonances at 145 ppm of C
N bonds, proving the successful condensation of TPA (or BPDA) and BTCH again.12b
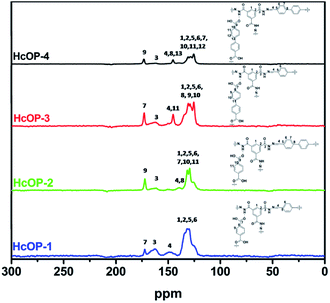 |
| Fig. 2 Solid-state 13C CP/MAS NMR spectra of HCOPs. | |
The HCOP-1 and HCOP-2 are stable in N2 up to 300 °C, while the HCOP-3 and HCOP-4 are stable in N2 up to 350 °C, respectively, as revealed by TGA (Fig. S5, ESI†). The porous structures of the HCOPs were investigated by nitrogen sorption measurements at 77 K.13 In light of the IUPAC classification, the HCOPs all exhibited type IV sorption isotherm curves (Fig. S6 and S7, ESI†). Among the four HCOPs, HCOP-4 displayed the relatively highest N2 uptake with a BET surface area of 43 m2 g−1 (Table S1, ESI†). And the remaining HCOPs were arranged in descending order by the surface areas: HCOP-3 (41 m2 g−1) > HCOP-2 (18 m2 g−1) > HCOP-1 (13 m2 g−1). The Brunauer–Emmett–Teller (BET) surface areas of HCOPs increased along with increasing length of linkers, briefly, the surface areas are proportional to the length of linkers under the reaction conditions used. This finding is in accordance with the previous PTPA network.14
Adsorption properties
Adsorption amounts of ciprofloxacin and norfloxacin onto four HCOPs at different pH values were depicted in Fig. 3a and b. It can be seen that the equilibrium adsorption capacities (qe) of ciprofloxacin and norfloxacin both showed the increasing tendency and then decreasing tendency with pH values varied from 2 to 10. This may be related to the various zeta potential values of HCOPs and the different species of ciprofloxacin (Ka1 = 6.1, Ka2 = 8.7) and norfloxacin (Ka1 = 6.2, Ka2 = 8.5) changing with pH, to be specific, cationic species (pH < 5.9 ± 0.15), zwitterionic species (6.1 < pH < 8.9) and anionic species (pH > 8.9 ± 0.11).15 Typically, ciprofloxacin and norfloxacin contain both N–H and –COOH groups, which can be combined with the H+ and OH− in the solution, thus affecting the fluoroquinolones adsorption onto HCOPs (Fig. 4). Therefore, the changing of pH induced the mutual transformation of the electrostatic interactions and electrostatic repulsive force between fluoroquinolone molecules and the HCOP surfaces, giving rise to the emergence of the maximum adsorption amounts at pH = 6.0. Accordingly, the optimal pH for the four HCOPs over the two kinds of fluoroquinolones was pitched on pH = 6.0, which was for studying go a step further.
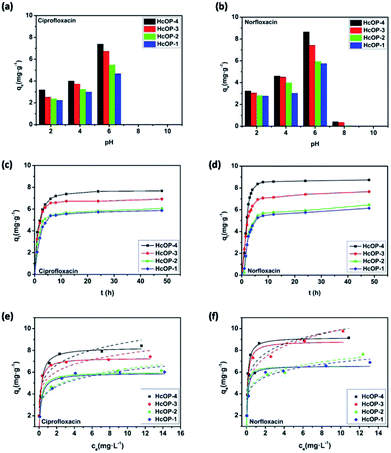 |
| Fig. 3 (a) and (b) Effect of solution pH on antibiotics adsorption onto HCOPs. (c) and (d) the fluoroquinolone adsorption capacity under different contact times (C0 = 10 mg L−1, pH = 6.0). (e) and (f) Langmuir and Freundlich adsorption isotherms of fluoroquinolones adsorption onto HCOPs. Dashed line: Freundlich model; solid line: Langmuir model. | |
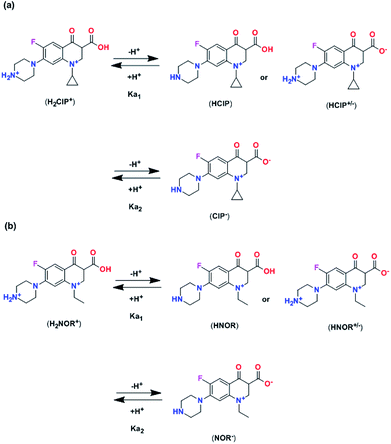 |
| Fig. 4 The speciation of process reactive for ciprofloxacin (a) and norfloxacin (b) in aqueous solution as a function of the solution pH. | |
For the sake of investigating the behaviour of fluoroquinolones onto the HCOPs, batch adsorption experiments were conducted at different reaction times for the initial concentration of 10 mg L−1 (pH = 6.0). As shown in Fig. 3c and d, the adsorption processes of ciprofloxacin and norfloxacin on HCOPs were rapid at the initial adsorption stage of 6 h, after which the adsorption rate no longer changed and eventually entered the adsorption equilibrium process. The adsorption data of the HCOPs over fluoroquinolones were processed by pseudo first- and second-order kinetic models, intraparticle diffusion model (Fig. S10, ESI†).16 Owing to the higher linear fitting coefficient (R2) values and closer calculated equilibrium adsorption capacity (qe,cal) values of the two kinetic models, the second-order kinetic model described the nature of adsorption process more suitably (Tables S2 and S4, ESI†). Moreover, the linear relationship between the qt and t0.5 indicated the combined process of intraparticle diffusion and the external mass transfer in adsorption processes of fluoroquinolones onto the HCOPs being studied (Tables S3 and S5, ESI†). For the adsorbed rates of antibiotics, the orders are in following sequence of HCOP-1 < HCOP-2 < HCOP-3 < HCOP-4, which can be contributed to the differences in specific surface areas and pore sizes.17 Besides, the removal efficiencies (E) of fluoroquinolones onto the same kind of HCOPs increased as Eciprofloxacin < Enorfloxacin, which is related to the hydrophobic interactions between the adsorbent and adsorbate.
In order to evaluate the adsorption capacities of fluoroquinolones onto the HCOPs, the adsorption amounts were tested as a function of various initial antibiotic concentrations (Fig. 3e and f). Both the Freundlich and Langmuir models were performed to fit test data (Fig. S11, ESI†).18 By comparing the linear fitting coefficient (R2) values of both ciprofloxacin and norfloxacin, we can draw a conclusion that the adsorption process was interpreted reasonably well by the Langmuir isotherm model (Tables S6–S9, ESI†). Based on the data listed, the maximum adsorption capacity (qm) values calculated from the Langmuir model of four HCOPs over two fluoroquinolones were both in the order of HCOP-4 > HCOP-3 > HCOP-2 > HCOP-1, which impelled us to believe that the more favorable adsorption over HCOP-4 than the adsorption over HCOP-3, HCOP-2 or HCOP-1. This phenomenon is commonly observed in the adsorption process of porous materials, there, greater specific surface area appear to favour the higher adsorption capacity.19 The other side, the qm values of norfloxacin were larger than that of ciprofloxacin when using the same HCOP as adsorbent. It is worth noting that with the values, HCOPs could exceed some commercial materials and even some synthetic zeolites, providing the great feasibility as a popular alternative of existing adsorbents which avoids complex synthesis (Table S10†). Afterwards, the morphologies and structures of the HCOPs after adsorption were characterized by SEM images and FT-IR analysis in succession. As shown in Fig. S12–16,† the morphologies and structures of fluoroquinolone-loaded networks did not change significantly with regard to the parent networks, which further elaborated on the stability of HCOPs.
Since the sodium chloride commonly existed in the most wastewater, the influence of ionic strength (NaCl) on fluoroquinolones adsorption onto cross-sectional HCOP-4 was carried out. As shown in Fig. 5a, the decreased adsorption amounts under low NaCl conditions (0 to 0.2 M) may be explained by the competitive adsorption of Na+ cations with positive fluoroquinolones on the HCOP-4 surface. However, when ionic strength is large enough, the salting out effect between fluoroquinolone molecules and NaCl, which leading to the decreased solubility of fluoroquinolone molecules in aqueous solution, resulting in the enhanced hydrophobic interactions between fluoroquinolones and HCOP-4. Therefore, more fluoroquinolones were facilitated to diffuse the surface of the HCOP-4, bringing about the increased adsorption amounts of fluoroquinolones under high initial NaCl concentrations (0.5 to 1 M).20
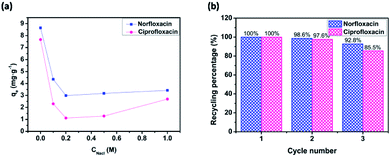 |
| Fig. 5 (a) Effect of coexisting ions on adsorption capacity of fluoroquinolones onto HCOPs at pH = 6.0. (b) Relative adsorption capacity of fluoroquinolones onto HCOPs after recycling. | |
The recyclability of the HCOP adsorbents towards fluoroquinolones were investigated by using generated adsorbents for the subsequent adsorption cycles under the same conditions to explore the practical value. Similarly, HCOP-4 was selected as representative adsorbent for detailed investigation. The results in Fig. 5b demonstrated that the recycling percentage of norfloxacin still maintained 92.8% (while 85.5% for that of ciprofloxacin) of the initial capacity after three recycles, defining the good repeatable application of HCOPs in treatment of fluoroquinolones pollution.
Adsorption mechanism
To get a deep understanding of the mechanisms of adsorption for fluoroquinolones onto the four HCOPs, we speculated that it may be attributed to a combination of perforated porousness, electrostatic interaction, hydrophobic interaction, π–π electron-donor–acceptor (EDA) interaction and hydrogen bonding formation (Fig. 6).21 Remarkably, the perforated porousness assumed enormous importance in the adsorption progress as discussed before that the large specific surface area is propitious to high adsorption capacity. The electrostatic interaction was without question one of the major effecting factors for antibiotics adsorption onto the HCOPs. We are informed of the zeta potential curves that when the pH was at 6.0, the surfaces of all the HCOPs were negatively charged (Fig. S8, ESI†); in contrast, the norfloxacin and ciprofloxacin can exist as cationic forms with positive charge. On these grounds, adsorption amounts were enhanced as expected due to the electrostatic attraction force between antibiotic molecules and the HCOPs surfaces. Additionally, in terms of higher E and qm values for norfloxacin in comparison with ciprofloxacin onto HCOPs, the dominated role of hydrophobicity in fluoroquinolone adsorption has been fully given weight. Now that norfloxacin and ciprofloxacin could function as π-acceptors on account of electron-withdrawing –F groups; conversely, –CO–NH− functional groups endow the HCOPs with π-electron-rich skeletons, which could function as π-donors, the π–π EDA interaction are prone to effect significantly in the fluoroquinolones adsorption onto the HCOPs. Last, hydrogen bond information also has a certain impact on the fluoroquinolones adsorption onto the HCOPs. The –CO–NH– and –COOH functional groups on HCOPs make them could act as hydrogen bond-donors as well as hydrogen bond-acceptors; correspondingly, the norfloxacin and ciprofloxacin could act as both hydrogen bond-acceptors and hydrogen bond-donors due to the –NH, –COOH and –F functional groups.
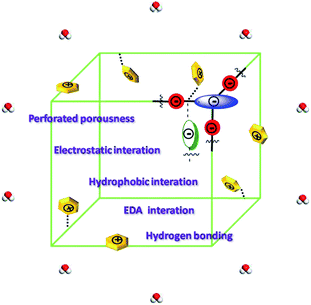 |
| Fig. 6 Schematic diagram of possible mechanisms for adsorptive removal of fluoroquinolones. | |
Conclusions
In conclusion, four novel porous hydrogen-bonding covalent organic polymers (HCOPs) have been developed based on three-composite building blocks as carriers to adsorb emerging fluoroquinolone pollutants via a facile method for the first time. The specific surface areas and pore sizes of these porous HCOPs, which bringing about the diverse in adsorption capacity, could be regulated to a certain extent by varying the lengths of strut. Or rather, the other three HCOPs exhibited comparably low adsorption capacity in contrast to HCOP-4, which is a result that the absorbing abilities adhere to the order of porous characteristics. In the meantime, the functional groups on the skeletons of HCOPs, such as –CO–NH– and –COOH groups, favored the adsorption of fluoroquinolones. In addition, it can be inferred that other possible controlling mechanisms such as electrostatic interaction, hydrophobic interaction, π–π electron-donor–acceptor (EDA) interaction and hydrogen bonding formation also accompanied with effect on the fluoroquinolones adsorption onto HCOPs.
Conflicts of interest
There are no conflicts to declare.
Acknowledgements
This work was supported by the National Natural Science Foundation of China (Project No. 21601177, 41572213 and 41772241) and the 111 Project (B16020).
Notes and references
- F. Pomati, S. Castiglioni, E. Zuccato, R. Fanelli, D. Vigetti, C. Rossetti and D. Calamari, Environ. Sci. Technol., 2006, 40, 2442–2447 CrossRef CAS PubMed.
- K. G. Karthikeyan and M. T. Meyer, Sci. Total Environ., 2006, 361, 196–207 CrossRef CAS PubMed.
- A. Hartmann, E. M. Golet, S. Gartiser, A. C. Alder, T. Koller and R. M. Widmer, Arch. Environ. Contam. Toxicol., 1999, 36, 115–119 CrossRef CAS PubMed.
- W. H. Xu, G. Zhang, S. C. Zou, Z. H. Ling, G. L. Wang and W. Yan, Water Environ. Res., 2009, 81, 248–254 CrossRef CAS PubMed.
- Z. J. Liang, Z. W. Zhao, T. Y. Sun, W. X. Shi and F. Y. Cui, J. Hazard. Mater., 2016, 305, 8–14 CrossRef CAS PubMed.
-
(a) J. L. Segura, M. J. Mancheno and F. Zamora, Chem. Soc. Rev., 2016, 45, 5635–5671 RSC;
(b) A. P. Côte, A. I. Benin, N. W. Ockwig, M. O'Keeffe, A. J. Matzger and O. M. Yaghi, Science, 2005, 310, 1166–1170 CrossRef PubMed;
(c) X. Han, Q. Xia, J. Huang, Y. Liu, C. Tan and Y. Cui, J. Am. Chem. Soc., 2017, 139, 8693–8697 CrossRef CAS PubMed;
(d) B. Sun, C. H. Zhu, Y. Liu, C. Wang, L. J. Wan and D. Wang, Chem. Mater., 2017, 29, 4367–4374 CrossRef CAS;
(e) W. J. Luo, Y. X. Zhu, J. Y. Zhang, J. J. He, Z. G. Chi, P. W. Miller, L. P. Chen and C. Y. Su, Chem. Commun., 2014, 50, 11942–11945 RSC;
(f) L. Guo, M. Wang, X. F. Zeng and D. P. Cao, Mater. Chem. Front., 2017, 1, 2643–2650 RSC;
(g) Q. Sun, B. Aguila and S. Q. Ma, Mater. Chem. Front., 2017, 1, 1310–1316 RSC;
(h) H. S. Yang, Y. L. Zhu, Y. Du, D. Z. Tan, Y. H. Jin and W. Zhang, Mater. Chem. Front., 2017, 1, 1369–1372 RSC.
-
(a) M. Calik, T. Sick, M. Dogru, M. Döblinger, S. Datz, H. Budde, A. Hartschuh, F. Auras and T. Bein, J. Am. Chem. Soc., 2016, 138, 1234–1239 CrossRef CAS PubMed;
(b) D. A. Vazquez-Molina, G. M. Pope, A. A. Ezazi, J. L. Mendoza-Cortes, J. K. Harper and F. J. Uribe-Romo, Chem. Commun., 2018, 54, 6947–6950 RSC;
(c) Y. C. Yuan, B. Sun, A. M. Cao, D. Wang and L. J. Wan, Chem. Commun., 2018, 54, 5976–5979 RSC;
(d) F. Yuan, J. Tan and J. Guo, Sci. China: Chem., 2018, 61, 143–152 CrossRef CAS;
(e) L. Lin, H. D. Guan, D. L. Zou, Z. J. Dong, Z. Liu, F. F. Xu, Z. G. Xie and Y. X. Li, RSC Adv., 2017, 7, 54407–54415 RSC.
- S. Chandra, T. Kundu, K. Dey, M. Addicoat, T. Heine and R. Banerjee, Chem. Mater., 2016, 28, 1489–1494 CrossRef CAS.
-
(a) Y. B. He, S. C. Xiang and B. L. Chen, J. Am. Chem. Soc., 2011, 133, 14570–14573 CrossRef CAS PubMed;
(b) Q. Yin, P. Zhao, R. J. Sa, G. C. Chen, J. Lü, T. F. Liu and R. Cao, Angew. Chem., Int. Ed., 2018, 57, 7691–7696 CrossRef CAS PubMed;
(c) Z. F. Ju, G. L. Liu, Y. S. Chen, D. Q. Yuan and B. L. Chen, Chem. - Eur. J., 2017, 23, 4774–4777 CrossRef CAS PubMed;
(d) W. Q. Yan, X. P. Yu, T. Yan, D. F. Wu, E. Ning, Y. Qi, Y. F. Han and Q. W. Li, Chem. Commun., 2017, 53, 3677–3680 RSC;
(e) H. L. Wang, H. Wu, J. L. Kan, G. G. Chang, Z. Z. Yao, B. Li, W. Zhou, S. C. Xiang, J. C. G. Zhao and B. L. Chen, J. Mater. Chem. A, 2017, 5, 8292–8296 RSC;
(f) Y. Zhou, B. Liu, X. D. Sun, J. T. Li, G. H. Li, Q. S. Huo and Y. L. Liu, Cryst. Growth Des., 2017, 17, 6653–6659 CrossRef CAS.
- Y. X. Lin, X. F. Jiang, S. T. Kim, S. B. Alahakoon, X. S. Hou, Z. Y. Zhang, C. M. Thompson, R. A. Smaldone and C. F. Ke, J. Am. Chem. Soc., 2017, 139, 7172–7175 CrossRef CAS PubMed.
-
(a) X. H. Guo, Y. Tian, M. C. Zhang, Y. Li, R. Wen, X. Li, X. F. Li, Y. Xue, L. J. Ma, C. Q. Xia and S. J. Li, Chem. Mater., 2018, 30, 2299–2308 CrossRef CAS;
(b) S. X. Duan, J. X. Li, X. Liu, Y. N. Wang, S. Y. Zeng, D. D. Shao and T. Hayat, ACS Sustainable Chem. Eng., 2016, 4, 3368–3378 CrossRef CAS.
-
(a) O. Kotova, R. Daly, C. M. G. dos Santos, M. Boese, P. E. Kruger, J. J. Boland and T. Gunnlaugsson, Angew. Chem., Int. Ed., 2012, 51, 7208–7212 CrossRef CAS PubMed;
(b) J. Q. Pan, L. P. Guo, S. Q. Zhang, N. Wang, S. B. Jin and B. E Tan, Chem.–Asian J., 2018, 13, 1674–1677 CrossRef CAS PubMed.
- K. W. Wang, L. M. Yang, X. Wang, L. P. Guo, G. Cheng, C. Zhang, S. B. Jin, B. E. Tan and A. Cooper, Angew. Chem., Int. Ed., 2017, 56, 14149–14153 CrossRef CAS PubMed.
- Y. Z. Liao, J. Weber, B. M. Mills, Z. H. Ren and C. F. J. Faul, Macromolecules, 2016, 49, 6322–6333 CrossRef CAS.
- S. Q. Li, X. D. Zhang and Y. M. Huang, J. Hazard. Mater., 2017, 321, 711–719 CrossRef CAS PubMed.
-
(a) S. Lagergren, K. Sven. Vetenskapsakad. Handl., 1898, 24, 1–39 Search PubMed;
(b) Y. S. Ho and G. McKay, Process Biochem., 1999, 34, 451–465 CrossRef CAS;
(c) S. X. Duan, R. F. Tang, Z. C. Xue, X. X. Zhang, Y. Y. Zhao, W. Zhang, J. H. Zhang, B. Q. Wang, S. Y. Zeng and D. Z. Sun, Colloids Surf., A, 2015, 469, 211–223 CrossRef CAS.
- Z. Hasan, J. Jeon and S. H. Jhung, J. Hazard. Mater., 2012, 209–210, 151–157 CrossRef CAS PubMed.
-
(a) I. Langmuir, J. Am. Chem. Soc., 1918, 40, 1361–1403 CrossRef CAS;
(b) H. Freundlich and W. Heller, J. Am. Chem. Soc., 1939, 61, 2228–2230 CrossRef CAS.
- J. Dong, F. F. Xu, Z. J. Dong, Y. S. Zhao, Y. Yan, H. Jin and Y. X. Li, RSC Adv., 2018, 8, 19075–19084 RSC.
- X. M. Peng, F. P. Hu, F. L. Y. Lam, Y. J. Wang, Z. M. Liu and H. L. Dai, J. Colloid Interface Sci., 2015, 460, 349–360 CrossRef CAS PubMed.
-
(a) H. Zhao, X. Liu, Z. Cao, Y. Zhan, X. D. Shi, Y. Yang, J. L. Zhou and J. Xu, J. Hazard. Mater., 2016, 310, 235–245 CrossRef CAS PubMed;
(b) J. Ma, M. X. Yang, F. Yu and J. Zheng, Sci. Rep., 2015, 5, 13578, DOI:10.1038/srep13578.
Footnote |
† Electronic supplementary information (ESI) available: Fig. S1–S16, Table S1–S10 and additional experimental and characterization details. See DOI: 10.1039/c8ra06806b |
|
This journal is © The Royal Society of Chemistry 2018 |
Click here to see how this site uses Cookies. View our privacy policy here.