DOI:
10.1039/C8RA07037G
(Paper)
RSC Adv., 2018,
8, 37050-37056
Bioinspired interconnected hydrogel capsules for enhanced catalysis†
Received
22nd August 2018
, Accepted 6th October 2018
First published on 2nd November 2018
Abstract
Polysaccharide-based hydrogel capsules with interconnected inner membranes have been prepared. The obtained capsules have a multi-layer internal structure composed of many invaginations of interconnected hydrogel membranes as mimics of the cristae in mitochondria. These cristae-like internal membranes endow the capsules with high specific area, which makes the capsules ideal supporting materials for catalytic species such as metal nanoparticles and enzymes.
1 Introduction
Metal nanoparticle (MNP)-based heterogeneous catalysts have attracted great interest due to their high surface area and enhanced catalytic efficiency.1–4 However, their high surface areas also led to strong interactions between the MNPs and made them prone to aggregation due to their high surface energy,5 which greatly hindered their catalytic efficiency. To overcome this limitation, various strategies have been developed to stabilize MNPs. One of the widely-used approaches was to immobilize MNPs on porous supporting materials. In this vein, polymeric hydrogel networks were shown to be capable of strongly binding to MNPs and significantly enhancing MNPs' kinetic stability in various catalysis applications.6–9 In particular, polysaccharides, the most abundant biopolymers in nature, have been extensively exploited as promising substrates for supporting nanoparticles.10,11 In most cases, polysaccharide-based porous materials for MNP supports were fabricated as MNP-loaded microspheres and the catalytically active MNPs was significantly decreased as the MNPs were trapped in the three dimensional fibrous networks of the microgel. These MNP-gel composites acted as micro-reaction systems or micro-reactors. To further enhance the catalytic efficiency of these micro-reactors by optimizing the structural design remains a great challenge.
On the other hand, nature already provides an elegant example of highly efficient micro-reactor: mitochondria, a double membrane-bound organelle found in the cytoplasm of nearly all eukaryotic cells, has the main function to produce adenosine triphosphate (ATP). The curved inner membrane of mitochondria has many invaginations, known as the cristae, which greatly extended the surface areas of inner membrane and catalytic active species such as the ATP synthetase bound in the inner membrane.12,13 Since heterogeneous reaction occurs on the surface, it is believed that the unique cristae structure with multi-layer inner membranes gives mitochondria high surface area which is responsible for the observed high productivity of mitochondria as a micro-reactor.14,15 Artificial multi-layered membrane hydrogels mimicking the hierarchical natural structures possess high specific area and controlled release property.16–19 The multi-layered structure and tunable physical properties demonstrated a new generation of hydrogel materials. The hierarchical soft material with microstructures in different scales can be a prerequisite for the bio-related function and catalytic applications.20,21 It could be envisioned that multi-layered hydrogels could be an ideal substrate for MNPs catalysis.
Herein we report the design and fabricate of chitosan hydrogel capsules with interconnected inner membrane as a mimic of mitochondria's double-membrane structure by alkaline partial-stimulating method in aqueous solution. As a proof of concept, inner membranes of the resulting micro-reactors was decorated with catalytic active silver nanoparticles (Ag NPs) and the performance of the resulting micro-reactors was evaluated in reduction of 4-nitrophenol. We believed that the utilization of chitosan, a renewable and abundant biopolymer, to fabricate novel micro-reactors with high catalytic efficiency by an environmentally friendly process, shows the potential of sustainable applications.
2 Experimental
2.1 Materials and methods
2.1.1 Materials. Chitosan (CS, degree of deacetylation 85%) was purchased from Sigma-Aldrich. Carboxymethylchitosan (CMCS, degree of carboxylation 80%), silver nitrate (AgNO3), sodium borohydride (NaBH4), trisodium citrate dihydrate (C6H5Na3O7·2H2O), 4-nitrophenol (4-NP), methylene blue (MB), sodium hydroxide (NaOH) and acetic acid (CH3COOH) were provided by local suppliers.
2.1.2 Preparation of interconnected hydrogel capsules. The hollow capsules were first prepared by adding a CS solution (2 wt% in 0.1 M, acetic acid) dropwise (with the nozzle diameter of 1.80 mm) into CMCS aqueous solution (2 wt%). The resulting hollow capsule with encapsulated CS solution was collected and subsequently rinsed with pure water. After that, the capsule was partially immersed in a NaOH solution (2.5 wt%) for 1 minute, and transferred into pure water for 1 minute. Thus, by simply repeating the base treatment and sequential water soaking, multiple and interconnected CS gel membranes in the CS-CMCS hydrogel capsules could be generated. For comparison, solid hydrogel capsules were prepared by immersing the hollow capsules into (2.5 wt%) NaOH solution for 10 min.
2.1.3 Preparation of Ag NPs loaded capsules. Ag NPs were in situ synthesized in chitosan hydrogel capsules with interconnected inner membranes or the solid hydrogel capsules. First, the capsules were immersed into AgNO3 solution (50 ml, 0.1 M) with magnetic stirring for 3 h at room temperature. During this process, Ag+ was adsorbed evenly into the matrices of capsules through complexing with –NH2. Subsequently, the capsules with Ag+ were collected and rinsed with distilled water for 20 min. After that, the capsules were immersed into 50 ml 1% (w/v) C6H5Na3O7·2H2O aqueous solution with magnetic stirring for 2.5 h at 100 °C. Due to the favorable mechanical and thermal properties, capsules were intact under vigorous stirring. Eventually, the dark Ag NPs loaded capsules were obtained, and rinsed with distilled water to remove excess reductive reagent.
2.1.4 Catalytic activity assays. The hydrogenation of 4-NP into 4-aminophenol (4-AP) by NaBH4 was chosen as the catalytic reaction. The reaction could be monitored by a UV-VIS spectrophotometer with a set time interval. First of all, 4-NP aqueous solution (25 ml, 0.1 mM) was mixed with NaBH4 aqueous solution (5 ml, 10 mM) in flask under stirring at room temperature, leading to a deep yellow solution. After the solution being mixed uniformly, 3 ml of the solution was transferred to a centrifuge tube as a control group. Then, 18 Ag NPs loaded capsules were added into 27 ml of the solution with sustained shaking. For every three minutes, 3 ml solution with 2 capsules were removed from the system for UV-VIS measurement.Similarly, the reduction of MB dye was carried out as follows. MB dye aqueous solution (1.67 ml, 0.1 mM) was mixed with NaBH4 aqueous solution (1.33 ml, 10 mM) in flask under stirring at room temperature, and the amount of the Ag NPs loaded hydrogel capsule was used the same as 2 capsules in 3 ml. The reduction of MB was monitored by the UV-VIS absorption spectroscopy.
2.2 Characterization
The solid-state 13C nuclear magnetic resonance (NMR) spectra of capsules were recorded on a spectrometer operating at 400 MHz frequency (13C-NMR, Bruker Avance, Germany). The thickness of the capsule membrane was measured and calculated by field emission-scanning electron microscope (FE-SEM, Hitachi S-4800, Japan) with 10 kV acceleration voltage. The appearance and structure of chitosan hydrogel capsules with interconnected inner membranes (dipping in water) were observed by a stereoscopic microscope (Micro optical instrument Co. Ltd. WSZ0745TB, China) under transmission light. The compression strength was measured on a universal tensile tester (SANT Test machine Co. Ltd. CMT6503, China) at a speed of 0.5 mm min−1. The specific surface area and the pore size distribution were measured by the Brunauer–Emmett–Teller (BET) and Barrett–Joyner–Halenda (BJH) methods of nitrogen adsorption–desorption analyzer at 77 K (Quantachrome Autosorb-iQ2-MP, USA). To remove all the adsorbed species, the samples were degassed at 105 °C under vacuum. The morphology of Ag NPs loaded capsule was performed on FESEM and SEM coupled with energy dispersive X-ray analysis (SEM-EDS, Hitachi S-4800, Japan). The high resolution transmission electron microscopy images were taken on a JEM-2010HR microscopy at 200 kV (HRTEM, JEOL Co. Ltd JEM-2010HR, Japan). The compositions of capsules were measured with X-ray photo-electron spectroscopy (XPS, Thermofisher Scientific ESCALAB 250, USA) equipped with a monochromatic X-ray source (Al Kα, 1486.6 eV). The X-ray diffraction pattern of capsules were measured by X-ray diffractometer (XRD, Rigaku Co. Smart Lab, Japan). The catalytic activity of Ag NPs loaded capsules was measured via the reduction of 4-NP to 4-AP using UV/VIS spectrophotometer (UV-3600 Shimadzu Co., Japan). The thermal property of hydrogel capsules was evaluated by Thermo Gravimetry (TG, Netzsch TG20F1, Germany) with a heating rate of 10 K min−1 from 30 to 700 °C. For all the tests, the samples were freeze-dried with lyophilizer (Alpha 1-4LSC CHRIST, Germany) at −60 °C for 24 h, except that the stereoscopic microscope observation, universal tensile and UV tests were measured in gel state.
3 Results and discussion
3.1 Mechanism of interconnected hydrogel capsules
The fabrication of chitosan hydrogel capsules with interconnected inner membranes involved two major steps: hollow capsules formation and sequential partial alkaline treatment of the capsules. Hollow capsules were first prepared by adding a CS (a positively charged polyelectrolyte) solution dropwise into CMCS (an amphoteric polyelectrolyte) aqueous solution. When the CS drops were added into the CMCS solution, a CS-CMCS polyelectrolyte membrane was formed at the interface, isolating the encapsulated CS solution from further interacting with the surrounding CMCS solution. The thickness of CS-CMCS membrane was measured by FE-SEM, which was about 10 μm (Fig. S1, ESI†). The composition of the CS-CMCS membrane was further confirmed by 13C NMR (Fig. S2, ESI†) and XPS spectra (Fig. S3, ESI†). After that, as illustrated in Scheme 1, the capsule was partially immersed in a NaOH solution and transferred into pure water repeatedly. During this process, a thin layer of CS hydrogel membrane with a crescent shape could be observed inside the capsule. Thus, by simply repeating the base treatment and sequential water soaking, multiple and interconnected CS gel membranes in the CS-CMCS hydrogel capsules could be generated (Scheme 1b). The optical images of the hollow capsules and hydrogel capsules with interconnected inner membranes were shown in Fig. S4 (ESI).†
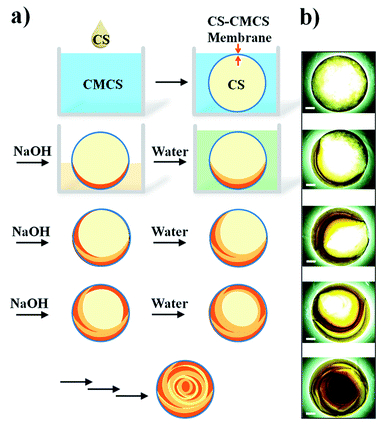 |
| Scheme 1 (a) Schematic illustration of the fabrication of chitosan hydrogel capsules with interconnected inner membranes. (b) Stereoscopic microscope photographs of capsules at different stages. Scale bar: 1 mm. | |
As expected for such a diffusion-reaction process, the thickness of newly formed inner gel membranes could be manipulated by varying the concentration of NaOH solution and the exposure time of the base treatment. As shown in Fig. 1a and c, the thickness of the resulting inner gel membrane was positively related to the concentration of NaOH used for the base treatment. Similarly, it was found that thicker inner gel membranes could be obtained with prolonged treatment time (Fig. 1b and d). These observations implied that the base concentration gradient resulting from diffusion of OH− might play an important role in the formation of the inner gel membranes.
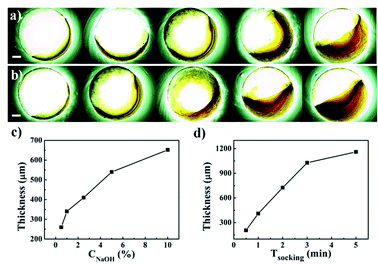 |
| Fig. 1 The effects of the NaOH concentration and exposure time on the thickness of inner gel membranes: (a) photographs of CS-CMCS capsules treated by NaOH solution with concentration of 0.5, 1, 2.5, 5 and 10 wt% for 1 min (from left to right, respectively). (b) Photographs of CS-CMCS capsules treated by NaOH (2.5 wt%) solution for 0.5, 1, 2, 5 and 8 min, respectively. (c) Plots of the thickness of inner gel membranes versus concentration of NaOH solution and (d) exposure time. Scale bar: 1 mm. | |
Fig. 2 indicated the mechanism of the capsule formation. The hollow capsule was partially exposed to basic solution, during which OH− passed through the permeable outer CS-CMCS gel membrane and led to localized deprotonation of pendent –NH3+ groups of CS in the exposed region, significantly reducing the solubility of CS in this region. As a result, a crescent-shaped thin layer of CS gel membrane (L1) appeared in (or “precipitate out of”) the capsule (Fig. 2b). The subsequent water soaking of the capsule led to the diffusion of the excess OH− into the inner region and a crescent-shaped inner gel membrane
with certain thickness was thus obtained (Fig. 2c).22 During this process, CS was neutralized incompletely due to the insufficient OH−. While in the second exposed process (hypothesis of overlap with L1 &
), the residual –NH3+ in
would be neutralized first (Fig. 2d1) and a gap formed between the first two inner membranes, which could be attributed to the shrinkage of the CS volume due to the reduced electrostatic repulsion and recombination of hydrogen bond between CS molecules when the CS precipitated from the solution. After the formation of the
layer, OH− continuously diffused into inner capsule to form the third gel layer L2 (Fig. 2d2). Through repeating solution treatment, additional inner CS membranes could be readily formed. The interconnecting channels between the crescent-shaped inner membranes enabled small molecules to diffuse via the interconnected water-filled channels as a highway to quickly reach each crescent-shaped inner membrane inside the capsule and those molecules inside could also quickly find their way out of the capsule. The values of specific surface area and pore size were illustrated by N2 adsorption–desorption. Fig. S5† showed the N2 adsorption–desorption isotherms of the hydrogel capsules with interconnected inner membranes, which belonged to type II classification and H3 hysteresis loop.23 The values of specific surface area, total pore volume and pore size were calculated to be 36.742 m2 g−1, 0.111 cm3 g−1 and 12.083 nm, respectively, indicating the mesoporous feature of the hydrogel capsules. The value of surface area was higher than that of some former reported chitosan microspheres.24 The considerable physical properties of hydrogel capsules with interconnected inner membranes would greatly increase the matter transfer efficiency, resulting in enhanced productivity of the micro-reactors. In addition, the robust mechanical and thermal properties (Fig. S6†) of hydrogel capsules could be beneficial for the extreme catalytic environment.
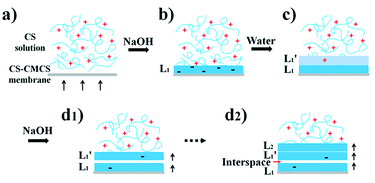 |
| Fig. 2 Proposed mechanism of multi-layered gel membranes formation in the capsules: (a) hollow capsules partially exposed in NaOH solution; (b) and (c) inner gel layers formed in NaOH solution and water, respectively; (d1) the inter-membrane space stimulated by NaOH solution in second exposed process; (d2) the formation of the third gel layer fabricated. | |
3.2 Morphology and structure of silver-loaded interconnected hydrogel capsules
The interconnected hydrogel capsules with large surface areas and interconnecting channels have great potential to be highly effective carriers for catalytic species in the field of heterogeneous catalysis. As a proof of concept, Ag NPs were chosen to be immobilized on outer and inner membrane surfaces to fabricate a nanoparticles-gel composite as a micro-reaction system. The efficiency of this micro-reaction system would be evaluated by the reduction reaction of 4-NP to 4-AP in the next part. The Ag NPs loaded hydrogel capsules were prepared as follows: first, the capsules were treated with silver nitrate solution to immobilize Ag+ ions to CS gel membranes though chelating interactions, and then Ag NPs were generated in situ in the presence of sodium borohydride as reducing reagent.25–27
The resulting nanoparticles-gel composite was characterized by FESEM and SEM-EDS to verify the presence of Ag NPs in membranes of capsules. FESEM photographs clearly showed that Ag NPs were successfully immobilized in the fibrous gel matrices with the diameters about 25 nm (Fig. 3a). Results of SEM-EDS analysis confirmed the presence of Ag NPs in the gel membranes (Fig. 3b).
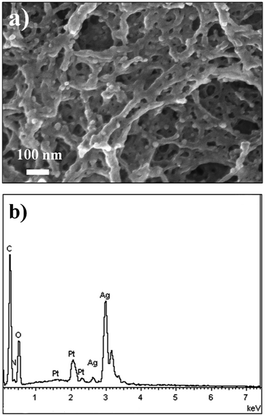 |
| Fig. 3 (a) FESEM photograph and (b) SEM-EDS analysis of Ag NPs in the gel membranes of interconnected hydrogel capsules. | |
The morphology and structure of Ag NPs in the membranes were also studied by TEM and HRTEM. TEM images showed that Ag NPs had a size of 20–25 nm (Fig. 4a), which were in consisted with the results obtained by FESEM. HRTEM and selected area electron diffraction (SAED) analysis showed clear lattice fringes which suggested that Ag NPs exhibited highly ordered crystalline-like structures (Fig. 4b). Result of XRD analysis of Ag NPs loaded gel membranes showed four distinct diffraction peaks at 38.24°, 44.22°, 64.68° and 77.54°, which indexed the planes (111), (200), (220) and (311) of the cubic face-centered silver, respectively (Fig. 4d). While capsules membrane without Ag NPs showed only a broad peak at 22.42° as a characteristic peak of amorphous chitosan (Fig. 4c).
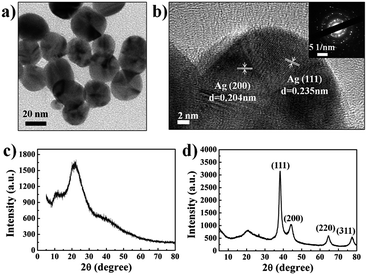 |
| Fig. 4 Analysis of Ag NPs embedded in interconnected hydrogel capsules: (a) TEM image. (b) HRTEM image and (inset) SAED pattern. XRD spectra of the interconnected hydrogel capsules (c) without and (d) with loading Ag NPs. | |
As shown in the XPS spectra (Fig. 5a), the Ag 3d peak was observed in Ag NPs loading capsule. In comparison, the Ag 3d peak was absent in those capsules without Ag NPs loading. The Ag 3d peak in narrow scan XPS spectrum of Ag NPs loading capsule revealed two peaks (Fig. 5b): Ag 3d5/2 and Ag 3d3/2 with binding energy of 373.8 and 367.9 eV, respectively. Compared with the reported value of Ag0 peaks at 374.2 and 368.2 eV,28 the observed Ag0 peaks slightly shifted to lower binding energy, which was believed as a result of the electron transfer from gel matrix to the bound Ag NPs.24 XPS analysis also suggested that the content of C, N, O and Ag elements on the membrane surface were proximately 58.48, 32.7, 7.43 and 1.39% respectively. The average Ag NPs mass content in capsules was estimated to be around 0.3105 mg per interconnected hydrogel capsule (4.50 mg per capsule in dry state in average) determined by ICP-AES.
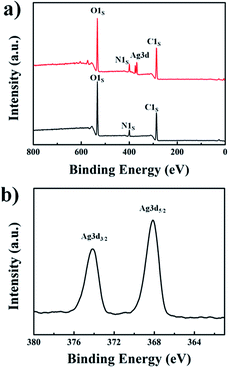 |
| Fig. 5 (a) XPS spectra of interconnected hydrogel capsules (bottom, black line) and the capsules loading Ag NPs (top, red line). (b) XPS narrow scan spectrum of Ag 3d peak. | |
3.3 Catalytic activity
As a model reaction, the catalytic reduction of 4-NP to 4-AP with sodium borohydride was used to investigate catalytic performance of Ag NPs loaded hydrogel capsules with interconnected inner membranes. The progress of the reaction was monitored by UV-VIS spectroscopy (Fig. 6a). The absorbance at ca. 400 nm was attributed to 4-NP ions in the presence of sodium borohydride.29 With the introduction of Ag NPs loaded hydrogel capsules into the reaction system, the absorbance at 400 nm gradually decreased with time and a new peak appeared at 290 nm which was attributed to the product 4-AP.30,31 Sodium borohydride was present in large excess, so its concentration was considered as constant throughout the reaction. Thus reaction rates could be assumed to be independent of the concentration of sodium borohydride, which allowed that the pseudo-first-order kinetics with respect to 4-NP were employed to evaluate the reaction rate,32
where C and C0 are the concentration of 4-NP at reaction time t and 0, and k is the apparent first-order rate constant. The ln(C/C0) against the reaction time was plotted for the reduction of 4-NP, as depicted in Fig. 6b. A linear relation of the plots of ln(C/C0) versus reaction time were observed for the catalytic reduction of 4-NP with Ag NPs loaded hydrogel capsules, which suggested that the reaction followed pseudo-first order kinetics. The k value was calculated based on the slope of the plotted line and the average value of k was estimated to be 0.1312 min−1, which was comparable with some related works of catalytic hydrogel systems listed in Table 1. For comparison, the catalytic activity of solid Ag NPs loaded hydrogel capsules was determined to be 0.1189 min−1 (about 90% of the k for multi-layered capsules), even though the solid hydrogel capsules were with higher Ag NPs loading (about 117% of the Ag amount for multi-layered capsules) as shown in Table 1. Due to the porosity of the hydrogel, the diffusion in the matrix was also essential as the solid hydrogel capsules exhibited a well catalytic activity, although the interconnected hydrogel capsules was more effective.
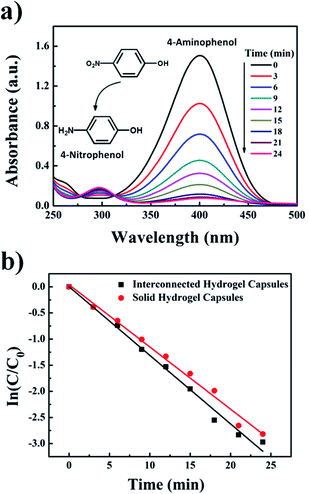 |
| Fig. 6 (a) Representative UV/VIS spectra of 4-NP catalytic reduction with sodium borohydride in the presence of Ag NPs loaded interconnected hydrogel capsules as a function of time. (b) Representative plots of ln(C/C0) against reaction time for the reduction of 4-NP by sodium borohydride in the presence of Ag NPs loaded interconnected hydrogel capsules and solid Ag NPs loaded hydrogel capsules at 400 nm. | |
Table 1 Comparison of kinetic rate constants (k) value of different catalytic hydrogel systems for NaBH4 reducing 4-NP in the recent literatures and our work
Catalyst |
c4-NP (M) |
MNPs loading (mg L−1) |
Amount of catalyst |
k (min−1) |
Ref. |
Ag NPs-embedded semi-IPN hydrogel |
2 × 10−4 |
622 |
10 mg in 2.7 ml |
0.374 |
33 |
Alginate-based biohydrogels (Ag@AMH) |
9.9 × 10−5 |
— |
Four beads in 2.525 ml |
0.27 |
34 |
Poly(NIPAAm-co-AMPS) hydrogel-Ag NPs |
2.16 × 10−3 |
— |
— |
0.0642 (26 °C) |
35 |
DNA hydrogel-Au NPs |
2 × 10−4 |
— |
100 mg in 1 ml |
0.09 |
27 |
P(AMPS) hydrogel-Ni NPs |
7.19 × 10−4 |
118.4 |
50 mg in 50 ml |
0.0563 (30 °C) |
26 |
Chitosan solid hydrogel-Ag NPs |
8.3 × 10−5 |
242 |
2 beads in 3 ml |
0.1189 |
This work |
Chitosan interconnected hydrogel-Ag NPs |
8.3 × 10−5 |
207 |
2 beads in 3 ml |
0.1312 |
This work |
In order to evaluate the efficiency of the catalyst for a long-term use, the recycle experiment was performed with the Ag NPs loaded interconnected hydrogel capsules (see in Fig. S7†). The recyclability of the catalyst exhibited satisfactory activity (>80%) after three cycles, and this macro-size hydrogels was beneficial for recycle and reuse. In addition, the significant reduction of MB was also shown in Fig. S8,† supporting the generic catalytic activity of the Ag NPs loaded hydrogel capsules.
The result demonstrated that the Ag NPs loaded hydrogel capsules with interconnected inner membranes exhibited decent catalytic performance in the reduction of 4-NP comparing with reported catalysts in macro-hydrogel system.
4 Conclusions
In conclusion, it was demonstrated that hydrogel capsules with cristae-like internal membranes could be prepared from polysaccharides with an iterative base-water treatment protocol. As a proof of concept, Ag NPs were immobilized in the capsules to fabricate a nanoparticles-gel composite as a micro-reaction system for catalytic reduction of 4-nitrophenol to 4-aminophenol. Ag NPs loaded interconnected hydrogel capsules showed excellent catalytic performance in the reduction of 4-nitrophenol. Giving their mitochondria-like unique features such as large reactive surface areas and good diffusion feature, these hydrogel capsules should have great potential to be highly effective carriers for catalytic species in the development of environmental friendly heterogeneous catalytic reaction systems.
Conflicts of interest
There are no conflicts to declare.
Acknowledgements
This work was supported by the National Natural Science Foundation of China (Grant No. 51233008), the Natural Science Foundation of Guangdong Province of China (Grant No. 2014A030311035) and Guangdong YangFan Innovative & Entrepreneurial Research Team Program.
Notes and references
- D. Astruc, F. Lu and J. R. Aranzaes, Angew. Chem., Int. Ed., 2005, 44, 7852 CrossRef CAS PubMed.
- L. Liu and A. Corma, Chem. Rev., 2018, 118, 4981 CrossRef CAS PubMed.
- R. Narayanan and M. A. El-Sayed, J. Am. Chem. Soc., 2003, 125, 8340 CrossRef CAS PubMed.
- M. Hermanek, R. Zboril, I. Medrik, J. Pechousek and C. Gregor, J. Am. Chem. Soc., 2007, 129, 10929 CrossRef CAS PubMed.
- A. Shahzad, W. S. Kim and T. Yu, RSC Adv., 2015, 5, 28652 RSC.
- B. Léger, S. Menuel, A. Ponchel, F. Hapiot and E. Monflier, Adv. Synth. Catal., 2012, 354, 1269 CrossRef.
- H. Hu, J. H. Xin, H. Hu, X. Wang, D. Miao and Y. Liu, J. Mater. Chem. A, 2015, 3, 11157 RSC.
- Y. Lu, P. Spyra, Y. Mei, M. Ballauff and A. Pich, Macromol. Chem. Phys., 2007, 208, 254 CrossRef CAS.
- D. D. Díaz, D. Kühbeck and R. J. Koopmans, Chem. Soc. Rev., 2011, 40, 427 RSC.
- T. R. Chetia, M. S. Ansari and M. Qureshi, Phys. Chem. Chem. Phys., 2016, 18, 5344 RSC.
- N. Sahiner, Prog. Polym. Sci., 2013, 38, 1329 CrossRef CAS.
- T. G. Frey and C. A. Mannella, Trends Biochem. Sci., 2000, 25, 319 CrossRef CAS PubMed.
- S. Cogliati, C. Frezza, M. E. Soriano, T. Varanita, R. Quintana-Cabrera, M. Corrado, S. Cipolat, V. Costa, A. Casarin, L. C. Gomes, E. Perales-Clemente, L. Salviati, P. Fernandez-Silva, J. A. Enriquez and L. Scorrano, Cell, 2013, 155, 160 CrossRef CAS PubMed.
- C. A. Mannella, Biochim. Biophys. Acta, 2006, 1762, 140 CrossRef CAS PubMed.
- G. A. Perkins, J. Tjong, J. M. Brown, P. H. Poquiz, R. T. Scott, D. R. Kolson, M. H. Ellisman and G. A. Spirou, J. Neurosci., 2010, 30, 1015 CrossRef CAS PubMed.
- B. C. Zarket and S. R. Raghavan, Nat. Commun., 2017, 8, 193 CrossRef PubMed.
- N. Lin, A. Gèze, D. Wouessidjewe, J. Huang and A. Dufresne, ACS Appl. Mater. Interfaces, 2016, 8, 6880 CrossRef CAS PubMed.
- Y. Xiong, K. Yan, W. E. Bentley, H. Deng, Y. Du, G. F. Payne and X.-W. Shi, ACS Appl. Mater. Interfaces, 2014, 6, 2948 CrossRef CAS PubMed.
- M. He, Y. Zhao, J. Duan, Z. Wang, Y. Chen and L. Zhang, ACS Appl. Mater. Interfaces, 2014, 6, 1872 CrossRef CAS PubMed.
- J. Nie, W. Lu, J. Ma, L. Yang, Z. Wang, A. Qin and Q. Hu, Sci. Rep., 2015, 5, 7635 CrossRef CAS PubMed.
- S. Cho, Y. Li, M. Seo and E. Kumacheva, Angew. Chem., Int. Ed., 2016, 55, 14014 CrossRef CAS PubMed.
- S. Ladet, L. David and A. Domard, Nature, 2008, 452, 76 CrossRef CAS PubMed.
- M. Kruk and M. Jaroniec, Chem. Mater., 2001, 13, 3169 CrossRef CAS.
- B. Duan, F. Liu, M. He and L. Zhang, Green Chem., 2014, 16, 2835 RSC.
- S. Butun and N. Sahiner, Polymer, 2011, 52, 4834 CrossRef CAS.
- N. Sahiner, H. Ozay, O. Ozay and N. Aktas, Appl. Catal., A, 2010, 385, 201 CrossRef CAS.
- A. Zinchenko, Y. Miwa, L. I. Lopatina, V. G. Sergeyev and S. Murata, ACS Appl. Mater. Interfaces, 2014, 6, 3226 CrossRef CAS PubMed.
- D. Lin, H. Wu, R. Zhang and W. Pan, Chem. Mater., 2009, 21, 3479 CrossRef CAS.
- S. Jana, S. K. Ghosh, S. Nath, S. Pande, S. Praharaj, S. Panigrahi, S. Basu, T. Endo and T. Pal, Appl. Catal., A, 2006, 313, 41 CrossRef CAS.
- A. A. Antipov, G. B. Sukhorukov, Y. A. Fedutik, J. Hartmann, M. Giersig and H. Möhwald, Langmuir, 2002, 18, 6687 CrossRef CAS.
- S. K. Ghosh, M. Mandal, S. Kundu, S. Nath and T. Pal, Appl. Catal., A, 2004, 268, 61 CrossRef CAS.
- S. K. Das, M. M. R. Khan, A. K. Guha and N. Naskar, Green Chem., 2013, 15, 2548 RSC.
- M. V. Patwadkar, C. S. Gopinathbc and M. V. Badiger, RSC Adv., 2015, 5, 7567 RSC.
- L. Ai, H. Yue and J. Jiang, J. Mater. Chem., 2012, 22, 23447 RSC.
- M. Wang, J. Wang, Y. Wang, C. Liu, J. Liu, Z. Qiu, Y. Xu, S. F. Lincoln and X. Guo, Colloid Polym. Sci., 2016, 294, 1087 CrossRef CAS.
Footnote |
† Electronic supplementary information (ESI) available. See DOI: 10.1039/c8ra07037g |
|
This journal is © The Royal Society of Chemistry 2018 |
Click here to see how this site uses Cookies. View our privacy policy here.