DOI:
10.1039/C8RA08263D
(Paper)
RSC Adv., 2018,
8, 41603-41611
Monolithic zirconia aerogel from polyacetylacetonatozirconium precursor and ammonia hydroxide gel initiator: formation mechanism, mechanical strength and thermal properties†
Received
6th October 2018
, Accepted 26th November 2018
First published on 12th December 2018
Abstract
Zirconia (ZrO2) aerogels are potential candidates for use at temperatures higher than those attainable with silica aerogels. However, fabricating a robust ZrO2 aerogel with a high thermal stability is still a challenge. The extreme electronegativity of Zr makes the hydrolysis and polycondensation of zirconium precursors difficult to control, leading to poor structural integrity and unsatisfactory physical properties. In the present research, we prepared a ZrO2 aerogel by using a synthetic zirconium precursor, namely polyacetylacetonatozirconium (PAZ), and ammonia hydroxide as the gel initiator. The ammonia hydroxide catalyzes the cross-linking of PAZ via promotion of the dehydration between hydroxyls in PAZ and the acetylacetonate group in PAZ binds the zirconium ion firmly upon the addition of ammonia hydroxide to avoid a gel precipitate. A monolithic ZrO2 aerogel with a large diameter size of 4.4 cm and high optical transmittance was achieved after drying. The surface area and pore volume of the as-dried ZrO2 aerogel were as high as 630.72 m2 g−1 and 5.12 cm3 g−1, respectively. They decreased to 188.62 m2 g−1 and 0.93 cm3 g−1 after being heat-treated at 1000 °C for 2 h. The best mechanical performances of the ZrO2 aerogels showed a compressive strength of 0.21 ± 0.05 MPa and a modulus of 1.9 ± 0.3 MPa with a density of 0.161 ± 0.008 g cm−3. Both pore structures and mechanical performances varied according to the ammonia hydroxide gel initiator used. The thermal insulating properties of the ZrO2 aerogel performed better than a silica aerogel blanket with a thermal conductivity of 0.020 W (m−1 K−1).
1 Introduction
Zirconia (ZrO2) aerogels are open-cellular, nanoporous materials that are randomly assembled by ZrO2 nanoparticles which have a typical mass fracture character.1,2 They were first prepared in 1976 by Teichner et al. through dissolution of zirconium alkoxide in organic solvent, gelation of the sol and supercritical drying of the gel.3 Although forty-two years have passed, ZrO2 aerogels have started to ignite research interest4–7 because of the growing demands for an aerogel which can be used at temperatures higher than those attainable with silica aerogels, which sinter and lose the mesopores at temperatures above 1000 °C. Since ZrO2 possesses a high melting point of 2715 °C, low thermal conductivity among metal oxides, and both acid and base active centers, ZrO2 aerogels are promising potential candidates in applications of high temperature thermal insulation,8 catalysts,9 electrodes in solid oxide fuel cells,10 etc. However, fabricating a monolithic ZrO2 aerogel with high strength and high thermal stability is still a challenge until now. The mechanical properties and thermal stability, which play a crucial role concerning commercial usage, are governed by the starting aerogel structure, which in turn is greatly affected by the synthesis reaction. In fact, the partial positive charge of the Zr atoms (δ+ ≈ 0.65) in alkoxides is much higher than Si atoms (δ+ ≈ 0.32),11 which makes the hydrolysis and polycondensation of zirconium alkoxides difficult to control, leading to poor structural integrity and unsatisfactory physical properties.
In early studies, zirconium alkoxides were used as the zirconium precursors.12–14 The overfast reaction rate of the sequential hydrolysis and polycondensation was modified by the addition of an acid catalyst.15 The as-received aerogel could be transparent but generally cracked.2 Consequently, the mechanical strengths were not well studied. Due to the high cost of the metal alkoxide and the sensitivity to humidity, zirconium alkoxides were gradually replaced by inorganic zirconium salts, i.e. zirconyl chloride, zirconyl nitrate, and zirconium chloride, especially after the propylene oxide (PO) induced gelation method was developed.15 The PO captures protons generated from the solvated zirconium precursor smoothly, leading to a slow increase of pH value. Hence, the inorganic salt precursors transfer into a stable ZrO2 gel network via hydrolysis and polycondensation. Chervin et al. synthesized a ZrO2 aerogel by using zirconium chlorides as the precursors and PO as the gel initiator.16 A monolithic ZrO2 aerogel with a high surface area of 406 m2 g−1 was obtained, however, the surface area degraded into 159 m2 g−1 and 26 m2 g−1 after calcination at 550 and 1000 °C, respectively, indicating the thermal stability of the aerogel via this method needs to be improved further. In addition, epoxides hold disadvantages such as the fact they are toxic, flammable and explosive and they are listed by the US Environmental Protection Agency as a group B2 possible human carcinogen which makes the epoxide induced gelation method not appropriate for mass production.17 More recently, strategies for post casting SiO2 thin layers on the initial ZrO2 aerogel have been widely accepted as an efficient way to improve thermal stability as well as mechanical strength.18–20
Polyacetylacetonatozirconium (PAZ) is an organozirconium oligomer with a formula expression of {Zr(OH)3acac}n (the acac represents the acetylacetonate group).21 The coordination between the acac and the Zr4+ ion, forming a structural stable hexatomic ring, suppresses the hydrolysis of the Zr4+ ion effectively. The PAZ alcoholic solution is quite stable so it could resist gelation for several days under room temperature even in the presence of excess water.22 The sol–gel transition of PAZ is milder than those of zirconium alkoxides and zirconium inorganic salts. This provides a fine control of the microstructure of sol–gel products, and consequently the related physical properties. In a recent communication,23 we reported the synthesis and characteristics of a monolithic ZrO2 aerogel by using PAZ as the zirconium precursor and PO as the gel initiator. The as-received ZrO2 aerogel has better mechanical strength and high temperature thermal stability at 1000 °C than those synthesized by zirconyl chloride and zirconyl nitrate precursors, indicating the potential usage of PAZ as a novel sol–gel precursor for high performance ZrO2 aerogels.
In the present research, under consideration of the drawbacks of the PO induced gelation method, we tried to synthesize a ZrO2 aerogel by using ammonia hydroxide as the gel initiator. Ammonia hydroxide is a strong base that causes gel precipitates rather than gel monoliths to be obtained when it is reacted with zirconium inorganic salts and/or zirconium alkoxides. However, we found that a rigid, homogeneous monolithic ZrO2 wet gel was achieved by using ammonia hydroxide as the gel initiator and PAZ as the zirconium precursor. Moreover, the surface area and pore volume of the as-dried ZrO2 aerogel were found to be larger than those synthesized by a PO gel initiator. The underlying formation mechanism was explored. The dependence of the microstructure, compressive strength and thermal stability at 1000 °C of the ZrO2 aerogel on the ammonia hydroxide gel initiator were investigated. Lastly, a piece of the ZrO2 aerogel slice with a diameter of 4.4 cm and a thickness of 3 mm was used to study the thermal insulating properties, which included subjecting the slice to an ethanol flame and recording the time-dependent back side temperature.
2 Experimental section
2.1 Chemicals
Polyacetylacetonatozirconium (PAZ, self-synthesis, Mw ∼ 2000–3000), tetraethoxysilane (TEOS, Aladdin, 98%), formamide (FA, Aladdin, 99%), anhydrous ethanol (EtOH, Fuyu, 99.5%), ammonia hydroxide (NH3·H2O, Aladdin, 25–28%) and distilled water (DI).
2.2 Synthesis of the ZrO2 aerogel
For a typical synthesis, 1.3 g PAZ was added into 15 mL anhydrous alcohol with magnetic stirring. After total dissolution, a clear yellow solution was obtained. Then, 5 mL distilled water and 200 μL FA were added into the solution in turn. After being magnetically stirred for 30 min, designated amounts of ammonia hydroxide of 100 μL, 200 μL and 400 μL were added into three PAZ sol samples which were denoted NH3-1, NH3-2 and NH3-3, respectively. Subsequently, the sols were transferred into polypropylene molds, sealed and placed into a water bath at 70 °C. The sol gradually turned into a monolithic gel. After gelation, the wet gel was demolded, aged under room temperature for 1.5 days and washed with ethanol every 12 h. For the strengthening of the skeleton of the wet gel, the wet gel was aged in a TEOS/EtOH mixed solution with a volume ratio of 1
:
1 for 3 days. Then, the unreacted TEOS/EtOH mixed solution was exchanged by EtOH. At last, the wet gel was dried in an autoclave with supercritical fluid N2–ethanol. The temperature and pressure of the supercritical fluid were set as 270 °C and 9 MPa. The autoclave held the desired temperature and pressure for 2 h to effectively remove the solvent.
2.3 Characterization
IR spectra were recorded on a Nicolet 5DX-FTIR spectrometer using the KBr pellet method in the range of 4000–375 cm−1. N2 adsorption–desorption at 77 K was measured by the JW-BK112 surface area and pore size analyzer after the samples were evacuated at 180 °C for 5 h under vacuum. The pore size distribution and the pore volume were determined via the BJH (Barrett–Joyner–Halenda) method from the adsorption curve. The morphologies of the obtained aerogel were determined by SUPRA™ 55 Thermal Field Emission Scanning Electron Microscopy. Transmission electron microscopy (TEM) images were recorded using a JEM-200CX electron microscope operating at 20 kV. The density was determined by measuring the weight and volume of the aerogel, respectively. X-ray diffraction (XRD) was performed on a Bruker D8 advance X-ray diffractometer at 40 kV and 100 mA with CuKα (λ = 1.540598 Å) radiation, employing a scanning rate of 5° min−1 in the 2θ range from 10° to 80°. The compressive strength of the aerogel was measured by using a WDW-5 Electronic Testing Machine under a quasi-static condition at a cross head speed of 1 mm min−1.
3 Results and discussion
3.1 Gelation and appearance
The reaction between ammonia hydroxide and the zirconium precursors usually achieves a precipitate due to the over-fast reaction rate. Huang et al. prepared a ZrO2 aerogel via the sol–gel synthesis of the ZrO2 wet gel by using zirconium sulfate as the zirconium precursor and ammonia as the gel initiator followed by ethanol SCD.24 The produced wet gel was slurry and only ZrO2 aerogel powders rather than a monolith were obtained after drying. However, in our research, the PAZ sol remained clear and unchanged for several days at room temperature after the addition of ammonia hydroxide which is very different from those of the zirconium inorganic salts and zirconium alkoxides counterparts. After being transferred into a 70 °C water bath, the PAZ sol gradually lost flowability and turned into a rigid, homogeneous wet gel. The gel time is about 1.5 h for NH3-1. With the increase of ammonia hydroxide, the gel time decreases to about 45 min and 30 min for NH3-2 and NH3-3, respectively. The NH3-1 wet gel has a high homogeneity so that a large sized ZrO2 aerogel with high optical transmission was achieved after drying as shown in Fig. 3. It is interesting to explore the underlying formation mechanism of the monolithic gel instead of precipitate during the reaction between the PAZ precursor and ammonia hydroxide gel initiator. The NH3-1 wet gel was ambiently dried in a drying oven at 50 °C. After that, the IR spectrum of the as-received NH3-1 xerogel was measured to be compared to that of PAZ. As shown in Fig. 1a, in PAZ, the absorption peak at 1588 cm−1 is ascribed to the stretching vibration of the carbonyl coming from the coordinated acac group. The absorption peak at 1530 cm−1 comes from the ethylene linkage of the acac group.25 These two absorption peaks were both observed in the NH3-1 xerogel indicating the acac group remained in the xerogel as well as the NH3-1 wet gel. The absorption peak at 3446 cm−1 broadens in the NH3-1 wet gel due to the formation of hydrogen bonds between the hydroxyls. The emerged blunt peaks near 500 cm−1 in the NH3-1 xerogel is evidence of the formation of Zr–O–Zr networks.26 We inferred that, with the addition of ammonia hydroxide followed by the increase of the pH value, dehydration between the hydroxyls in PAZ occurred, leading to blunt peaks near 500 cm−1. With the formation of Zr–O–Zr networks, the interactions of the PAZ precursor oligomer were promoted, boosting the emergence of hydrogen bonds which is a consequence of the broadened peak at 3446 cm−1 in NH3-1. Both the molecular structures (the middle row in Fig. 2) and the reaction equations (the bottom row in Fig. 2) could illustrate that dehydration occurred within the PAZ precursors. As a result, the Zr–O–Zr networks act as cross-linking points inducing the gelation of PAZ as shown in the formation scheme (the top row in Fig. 2). The IR spectra of the as-received NH3-1, NH3-2, and NH3-1 aerogel were measured and are shown in Fig. 1b. As indicated in the figure, the peaks located at 3431 and 1632 cm−1 belong to the surface absorbed –OH and H2O. The peaks located at 2982 and 1396 cm−1 are ascribed to –CH2– and CH3. Due to silylation treatment as shown in Fig. 2, the peak of Si–O–Si is located at 1066 cm−1.27 The peaks at 794 and 455 cm−1 come from the Zr–O–Zr network which formed during the gelation process as we analyzed. The absorption peaks ascribed to the acac group in the PAZ precursor were not observed since they were removed during SCD, either being dissolved in ethanol or from pyrolysis at high temperatures. After drying, only ZrO2 networks and some surface groups remained (Fig. 2).
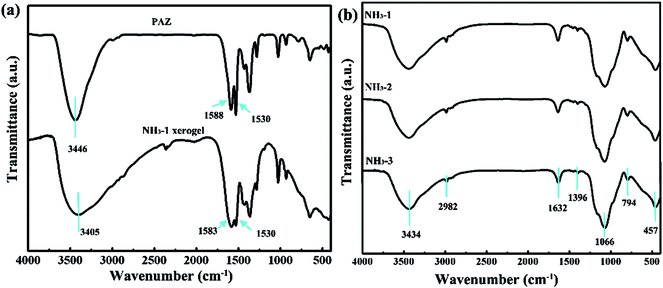 |
| Fig. 1 (a) IR spectra of PAZ and the NH3-1 xerogel. (b) IR spectra of the NH3-1, NH3-2, and NH3-3 aerogels. | |
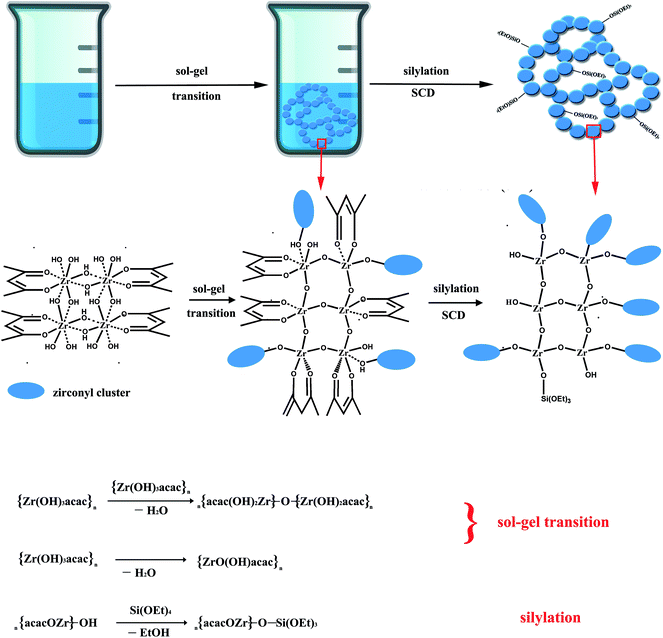 |
| Fig. 2 Schematic of the formation mechanism of the ZrO2 aerogel. | |
After drying, as shown in Fig. 3a, enough ZrO2 aerogels cylinders with diameters of ∼1 cm for the study of the physical properties were obtained, indicating that our method has good reproducibility. The molded ZrO2 aerogel cylinders obtained after SCD had a meniscus (shown in Fig. S1†) on the surfaces, making the accurate determination of the physical properties difficult. The meniscus was eliminated by polishing on sand paper, producing a cylinder with a regular shape, indicating the excellent processability.28 Fig. 3b shows the photograph of a large sized NH3-1 aerogel monolith with a large diameter size of 4.4 cm. The aerogel has high optical transmittance so that the quadrille paper is distinguished through the aerogel monolith. We synthesized a ZrO2 aerogel by using PAZ as the zirconium precursors and PO as the gel initiator for comparison. The aerogel gelled by PO has better optical transmittance than that of NH3-1 as shown in Fig. 3b. We considered that the optical transmittance of the ZrO2 aerogel synthesized by the present method could be further improved via careful control of the gelation rate,29 such as further decreasing the ammonia hydroxide amount, lowering the gel temperature, etc. Fig. 3c and d show the typical bluish color in the reflection mode and yellowish-orange color in the transmission mode of the ZrO2 aerogel monolith induced by Rayleigh scattering, indicating the homogeneous microstructure and high optical transmittance of the aerogel.30
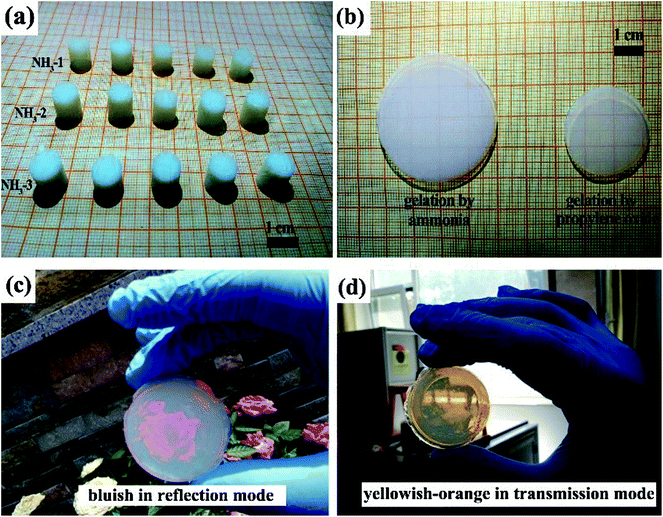 |
| Fig. 3 (a) Photographs of the NH3-1, NH3-2, and NH3-3 aerogel cylinders with a diameter of ∼1 cm. (b) Photograph comparison of the ZrO2 aerogel gelled by ammonia hydroxide and propylene oxide. Photograph of the bluish appearance in the reflection mode (c) and the yellowish-orange appearance in the transmission mode (d) of the NH3-1 ZrO2 aerogel monolith. | |
3.2 Microstructures
N2 adsorption/desorption was used to characterize the pore structure of the ZrO2 aerogels and the results are shown in Fig. 4. All isotherm curves are type IV. The flat stages of relative pressure between 0 to 0.8 are attributed to the multilayer adsorption on the external surface of the pores. At the relative pressure of 0.8–1.0, the abrupt increase of adsorption and the following desorption hysteresis ring result from the capillary condensation of N2 molecules in the mesopores with diameters of 2 nm to 50 nm. When the relative pressure was closed to 1, the adsorption was still unsaturated, indicating macropores with diameters larger than 50 nm. The pore size distribution (PSD) was analyzed from the desorption isotherm branch via the BJH method. As shown in the inset, all ZrO2 aerogels exhibit a mesoporous structure. The peak values of the PSD are located near 20 nm. It is obvious that the PSD curves broaden with the increase of ammonia hydroxide. This is because the increased gel initiator speeds the gelation reaction up, decreasing the homogeneity of the porous structure. According to the N2 adsorption/desorption results, the specific surface area (SSA) and pore volume (PV) were calculated by the BET and BJH method. As shown in Table 1, NH3-2 has the largest SSA and PV which reach up to as high as 630.72 m2 g−1 and 5.12 cm3 g−1, respectively. The SSA and PV of the ZrO2 aerogel are larger than those of most previously reported research and are only lower than the ZrO2 aerogel synthesized by zirconium alkoxide as the precursor and PO as the gel initiator.31
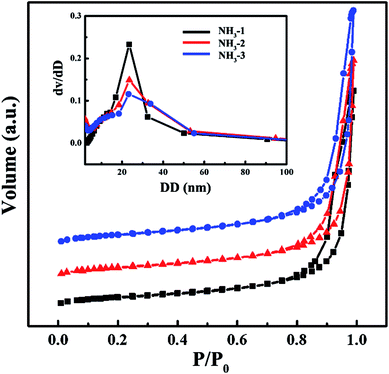 |
| Fig. 4 N2 isotherms of the NH3-1, NH3-2, and NH3-3 aerogels, the inset is the diameter distribution curves. | |
Table 1 The SSA and PV of the as-received ZrO2 aerogel after SCD
|
SSA (m2 g−1) |
PV (cm3 g−1) |
NH3-1 |
576.37 |
4.78 |
NH3-2 |
630.72 |
5.12 |
NH3-3 |
589.10 |
4.78 |
SEM images of the ZrO2 aerogels are shown in Fig. 5. As Fig. 5a shows, NH3-1 has a typical aerogel porous structure. The inter-connected secondary particles assemble into a three-dimensional nanoporous structure with most of the pore diameter sizes located at the mesopores scales. As can be seen from Fig. 5b and c, the porous ZrO2 aerogels gradually turn into dense structures with the increase of the ammonia hydroxide gel initiator. Large cracks were detected on NH3-3 as shown in Fig. 5c which was due to the fast gelation rate, leading to interior stress and the emergence of cracks. It is consistent with the facts that the gel time decreases with the increase of the ammonia hydroxide gel initiator. We also found in the experiments that the optical transmittance decreased with the increase of the ammonia hydroxide gel initiator which is caused by inhomogeneities such as cracks. TEM was used to further understand the microstructure of these ZrO2 aerogels. As shown in Fig. 5d–f, all aerogels exhibit a porous structure. The particles compactly connect to each other, forming the aerogel skeleton. From Fig. 5d–f, it could be observed that the aerogel skeleton became dense with the increase of the ammonia hydroxide gel initiator which is consistent with the SEM results.
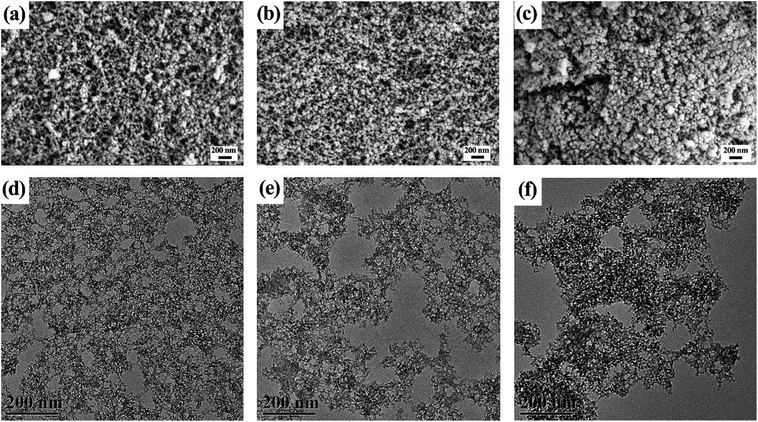 |
| Fig. 5 SEM images of the (a) NH3-1, (b) NH3-2, and (c) NH3-3 aerogels. TEM images of the (d) NH3-1, (e) NH3-2, and (f) NH3-3 aerogels. | |
3.3 Mechanical strength
The mechanical properties of the ZrO2 aerogels were determined via a uniaxial compression test. Before the test, the densities of the ZrO2 aerogel cylinders were calculated from the measured weight divided by the volume of the cylinders. Five cylinders were tested for one ZrO2 aerogel sample. The results are shown in Fig. 6. As shown in the figure, the compressive strength (Young's modulus) of NH3-2 is 0.21 ± 0.05 MPa (1.9 ± 0.3 MPa), slightly larger than that of NH3-1 which is 0.20 ± 0.04 MPa (1.8 ± 0.2 MPa). The slight increase in the strength of NH3-2 as compared to that of NH3-1 is likely to be caused by measuring errors since the increase is inconspicuous. However, as shown in the above-mentioned SEM results, the inter-connections between the secondary particles are improved for NH3-2 with the reduced large sized pores as compared to that of NH3-1, which theoretically allows the aerogels skeleton of NH3-2 to sustain higher loads.32 For NH3-3, the compressive stress (1.8 ± 0.2 MPa) is unambiguously lower than both NH3-2 and NH3-1. The modulus of NH3-3 is also the lowest among the three aerogels, indicating its poor mechanical properties. But, from the SEM results, the connections between the secondary particles continue to improve for NH3-3. We suggested that the macroscopic cracks in NH3-3 shown in the SEM images lead to the poor mechanical properties. In the compression test, we found that the ZrO2 aerogels showed a typical brittle nature. The stress–strain curves, shown in Fig. S2,† exhibit sudden decreases in stress when they reach critical values which are identified as fracture points. Herein, the mechanical properties could be explained by a fracture mechanism that shows that the material failure is induced by crack growth.33 At the fracture point, a bursting apart of the brittle ZrO2 aerogel was observed during the compression test, shown in Movie S1,† confirming that the failure of the aerogel is induced by the crack growth. As we observed in the SEM images, before the compression test, the intrinsic cracks had penetrated through the NH3-3 body. They are more likely to grow into catastrophic avulsion under external loads, inducing the fracture of the aerogel. As a result, NH3-3 has the lowest compressive stress.
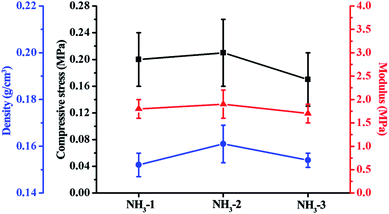 |
| Fig. 6 Densities, compressive stresses and modulus of the ZrO2 aerogels. | |
3.4 Thermal stability upon 1000 °C
In order to investigate the thermal stabilities of the pores in the ZrO2 aerogels in a high temperature environment, the aerogels were heat-treated in a furnace with a temperature program shown in Fig. 7a. After that, the N2 adsorption/desorption behaviors of the ZrO2 aerogels were studied. As shown in Fig. 7b, even when the aerogels were calcined at 1000 °C for 2 h, the isotherm curves still exhibited mesoporous characters as the obvious desorption hysteresis ring was detected during the relative pressure of 0.8–1.0. When the relative pressure was close to 1, the adsorption saturation platforms were not obvious in all isotherm curves, indicating the macropores in the aerogel samples. As shown in the inset, ZrO2 aerogels presented mesoporous PSD. The peak value of PSD for the NH3-1 aerogel is still located near 20 nm. However, those of the NH3-2 and NH3-3 aerogels shifted towards a large pore size of ∼30 nm. The SSA and PV for the ZrO2 aerogels after being heat-treated at 1000 °C for 2 h are listed in Table 2. The NH3-2 has the largest SSA and PV of 188.62 m2 g−1 and 0.93 cm3 g−1, respectively, indicating the best pore thermal stability against high temperatures. The appearances of the aerogel cylinder before and after heat-treatment at 1000 °C for 2 h were recorded by an optical camera. As shown in Fig. 7c, all cylinders were intact but obviously shrunken. The accurate linear shrinkages (Fig. 7d) were calculated via carefully measuring the diameter changes of the aerogel cylinders before and after heat-treatment. Although the shrinkages of the three aerogel cylinders are almost the same direction as deduced from the photograph shown in Fig. 7c, the calculated linear shrinkage values were 49.50%, 47.52% and 44.44% for NH3-1, NH3-2 and NH3-3, respectively, indeed showing a decreased trend for the aerogels with an increased gel initiator of ammonia hydroxide. The weight losses of the three aerogels during heat-treatment under an air atmosphere were investigated by a thermal analyzer (Fig. S3†). The weight losses show a decrease for NH3-1, NH3-2 and NH3-3, in accordance with the trend of the linear shrinkage, which is one of the factors resulting in the decreased linear shrinkages. Furthermore, from the TG curves, it should be noted that the weight losses below 100 °C, which are ascribed to surface absorbed hydroxyls and water molecules,23 show a decrease for NH3-1, NH3-2 and NH3-3, which means the decreased surface absorbed hydroxyls and water molecules. These surface absorbed hydroxyls are manifested to improve the sintering between the nanoparticles within the aerogel, leading to large linear shrinkages.34–36 Herein, the increased linear shrinkages of the aerogel cylinders may result from the increased surface hydroxyls and the increased sintering between nanoparticles. In fact, the shrinkage of the aerogel in a high temperature environment is derived from many factors, such as crystallization, crystal phase changes and sintering between the connected particles, etc. It seems that the linear shrinkages of our ZrO2 aerogels are not satisfactory because an aerogel monolith is considered as thermally stable only when it maintains a linear shrinkage lower than 2% when being subjected to a specific temperature. Further study is needed to determine how to decrease the linear shrinkages of our ZrO2 aerogel.
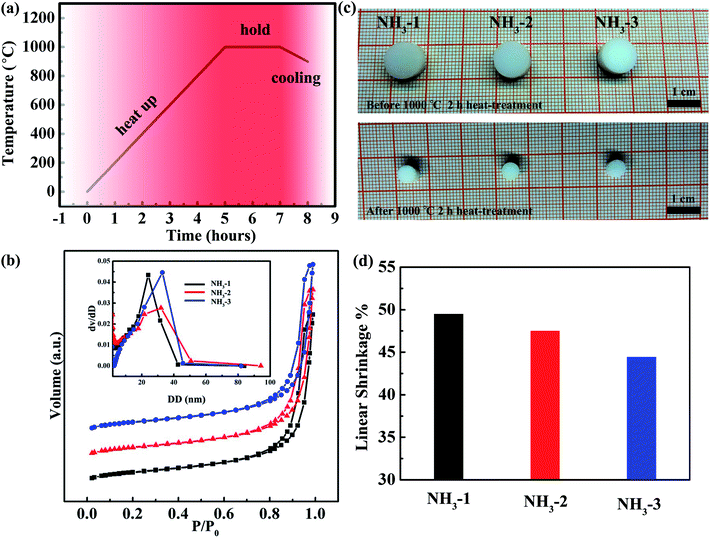 |
| Fig. 7 (a) Heat-treatment temperature programming curve. (b) N2 isotherms of the NH3-1, NH3-2, and NH3-3 aerogels after being heat-treated at 1000 °C for 2 h, the inset is the corresponding diameter distribution. (c) Photographs and (d) linear shrinkages of the ZrO2 aerogel cylinders before and after heat-treatment. | |
Table 2 SSA and PV of the ZrO2 aerogel after being heat treated at 1000 °C for 2 h
|
SSA (m2 g−1) |
PV (cm3 g−1) |
NH3-1 |
169.82 |
0.78 |
NH3-2 |
188.62 |
0.93 |
NH3-3 |
163.93 |
0.91 |
3.5 Thermal insulating properties
Microstructure studies demonstrate that the as-received ZrO2 aerogels have tremendous nano-pores with diameter sizes similar to the mean free path of air molecules. This makes ZrO2 aerogels capable of restraining the thermal convection of air molecules37 efficiently. Because ZrO2 has the lowest skeleton thermal conductivity among metal oxides, ZrO2 aerogels are considered as potential candidates for thermal insulating materials applied in high temperature environments. Herein, the determination of thermal conductivity of ZrO2 aerogels is academically valuable. In previous work, the thermal conductivity of ZrO2 aerogels was studied by transient thermal measurements such as hot wire and hot disk methods.38 The steady state thermal measurement of a pure ZrO2 aerogel has been rarely reported due to the need for a large sample size over 10 cm. In our research, in order to study the thermal insulating ability, the NH3-1 aerogel sample with a diameter size of 4.4 cm and a thickness of 3 mm was subjected to an alcohol lamp and the time-dependent temperature of the thermal image on the back side was recorded. At the same time, a ceramic fiber blanket, from Luyang Energy-Saving Materials Co., Ltd., with a thermal conductivity of 0.152 W m−1 K−1 and silica aerogel blankets, from Guangdong Alison Hi-Tech Co., Ltd., with a thermal conductivity of 0.020 W m−1 K−1 were used as the comparisons. Both the ceramic fiber blanket and the silica aerogel blanket have the same diameter and thickness as the NH3-1 ZrO2 aerogel. The alcohol lamp generated a flame of ∼400 °C. As shown in Fig. 8, subjecting the test samples to an alcohol flame for an extended time results in a slow increase of the back-side temperature. For the ceramic fiber blanket, the high temperature penetrated the sample as soon as the ceramic fiber blanket was subjected to the flame as shown in the infrared thermal images. The back-side temperature reached up to 235 °C when the time passed 180 s. As can be seen from Fig. 8c, the back-side temperature increase of the silica aerogel blanket is slower than that of the ceramic fiber blanket. It is due to the lower thermal conductivity of the silica aerogel blanket compared to that of the ceramic fiber blanket, meaning a higher thermal insulating ability. As can be seen in Fig. 8d, it is obvious that the back-side temperature of the ZrO2 aerogel is the lowest among the three test samples. The back-side temperature of the ZrO2 aerogels, when the time passed 180 s, is 196 °C, indicating a better thermal insulating ability than those of the ceramic fiber blanket and the silica aerogel blanket.
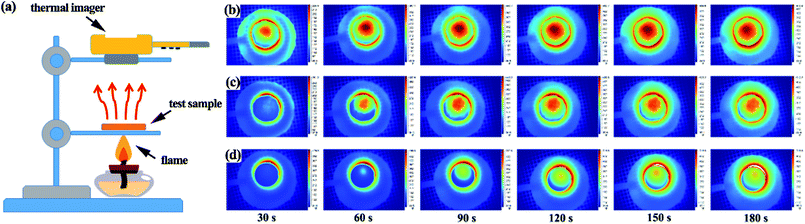 |
| Fig. 8 (a) Schematic of the thermal insulation test. Infrared thermal images of the cold surface of the test samples subjected to an ethanol flame (b) ceramic fiber blanket, (c) silica aerogel blanket, and (d) the ZrO2 aerogel monolith. | |
4 Conclusions
A monolithic ZrO2 aerogel was achieved by using polyacetylacetonatozirconium (PAZ) as the zirconium precursor and ammonia hydroxide as the gel initiator. The ammonia hydroxide gel initiator catalyzes the cross-linking of the PAZ precursor via promotion of the dehydration of hydroxyls intrinsically existing in PAZ and the acetylacetonate in PAZ binds the zirconium ion firmly to avoid over cross-linking which results in a gel precipitate. After drying, the surface area and pore volume of the ZrO2 aerogels were measured to be as high as 630.72 m2 g−1 and 5.12 cm3 g−1, respectively. They remained 188.62 m2 g−1 and 0.93 cm3 g−1 after being heat treated at 1000 °C for 2 hours. The best mechanical performances of the ZrO2 aerogels showed a compressive strength of 0.21 ± 0.05 MPa and a modulus of 1.9 ± 0.3 MPa with a density of 0.161 ± 0.008 g cm−3. Both pore structures and mechanical performance varied with the ammonia hydroxide gel initiator used. The thermal insulating property of the ZrO2 aerogel performed better than a ceramic fiber blanket with a thermal conductivity of 0.152 W m−1 K−1 and a silica aerogel blanket with a thermal conductivity of 0.020 W m−1 K−1. We believe that the robust, thermally stable ZrO2 aerogel with good thermal insulating properties achieved via the present method is a potential candidate to be used as a high temperature thermal insulator, catalyst and adsorbent at temperatures above 1000 °C.
Conflicts of interest
There are no conflicts of interest to declare.
Acknowledgements
National Natural Science Foundations of China (No. 21603125), Shandong Provincial Natural Science Foundation of China (ZR2016EMP04, ZR2017BEM009), Youth Science Funds of Shandong Academy of Sciences (2018QN0031) are acknowledged for financial support.
References
- L. BenHammouda, I. Mejri, M. Kadri Younes and A. Ghorbel, Aerogels Handbook, Spriner Science and Business Media, 2011, pp. 127–143 Search PubMed.
- A. Lecomte, F. Blanchard, A. Dauger, M. C. Silva and R. Guinebretiere, J. Non-Cryst. Solids, 1998, 225, 120–124 CrossRef CAS.
- S. J. Teichner, G. A. Nicolaon, M. A. Vicarini and G. E. Gardes, Adv. Colloid Interface Sci., 1976, 5, 245–273 CrossRef CAS.
- J. He, H. Zhao, X. Li, D. Su and H. Ji, Ceram. Interfaces, 2018, 44, 8742–8748 CrossRef CAS.
- X. Hou, R. Zhang and D. Fang, Scr. Mater., 2018, 143, 113–116 CrossRef CAS.
- G. Zu, J. Shen, L. Zou, W. Zou, D. Guan, Y. Wu and Y. Zhang, Microporous Mesoporous Mater., 2017, 238, 90–96 CrossRef CAS.
- X. Wang, C. Li, Z. Shi, M. Zhi and Z. Hong, RSC Adv., 2018, 8, 8011–8020 RSC.
- H. N. R. Jung, V. G. Parale, T. Kim, H. Hee Cho and H. H. Park, Ceram. Interfaces, 2018, 44, 10579–10584 CrossRef CAS.
- H. Kalies, N. Pinto, G. MarcelPajonk and D. Bianchi, Appl. Catal., A, 2000, 202, 197–205 CrossRef CAS.
- K. Tadanaga, K. Imai, M. Tatsumisage and T. Minami, J. Electrochem. Soc., 2002, 149, A773–A777 CrossRef CAS.
- J. Livage, M. Henry and C. Sanchez, Prog. Solid State Chem., 1988, 18, 259–341 CrossRef CAS.
- Y. W. Zeng, P. Riello, A. Benedetti and G. Fagherazzi, J. Non-Cryst. Solids, 1995, 185, 78–83 CrossRef CAS.
- D. Jin Suh and T. J. Park, Chem. Mater., 1996, 8, 509–513 CrossRef.
- C. Stocker and A. Baiker, J. Non-Cryst. Solids, 1998, 223, 165–178 CrossRef CAS.
- A. E. Gash, T. M. Tillotson, J. H. Satcher Jr, L. W. Hrubesh and R. L. Simpson, J. Non-Cryst. Solids, 2001, 285, 22–28 CrossRef CAS.
- A. N. Chervin, B. J. Clapsaddle, H. W. Chiu, A. E. Gash and J. H. Satcher, Chem. Mater., 2005, 17, 3345–3351 CrossRef.
- Z. Zhang, Q. Gao, Y. Liu, C. Zhou and M. Zhi, RSC Adv., 2015, 5, 84280–84283 RSC.
- Q. Wang, X. Li, W. Fen, H. Ji, X. Sun and R. Xiong, J. Porous Mater., 2014, 21, 127–130 CrossRef CAS.
- G. Zu, J. Shen, W. Wang, L. Zou, Y. Lian, Z. Zhang, B. Liu and F. Zhang, Chem. Mater., 2014, 26, 5761–5772 CrossRef CAS.
- H. Gao, Z. Zhang, Z. Shi, M. Zhi and Z. Hong, J. Sol-Gel Sci. Technol., 2018, 85, 567–573 CrossRef CAS.
- H. Y. Liu, X. Q. Hou, X. Q. Wang, Y. L. Wang and D. Xu, J. Am. Ceram. Soc., 2004, 87, 2237–2241 CrossRef CAS.
- M. Pan, J. R. Liu, M. K. Lv, D. Xu and D. R. Yuan, Thermochim. Acta, 2001, 376, 77–82 CrossRef CAS.
- B. Liu, X. Liu, X. Zhao, H. Fan, J. Zhang, X. Yi, M. Gao, L. Zhu and X. Wang, Chem. Phys. Lett., 2019, 715, 109–114 CrossRef CAS.
- Y. Y. Huang, B. Y. Zhao and Y. C. Xie, Appl. Catal., A, 1998, 172, 327–331 CrossRef CAS.
- K. Yuan, X. Gan, X. Wang, L. Zhu, G. Zhang and D. Xu, J. Therm. Anal. Calorim., 2017, 127, 1889–1895 CrossRef CAS.
- D. A. Powers and H. B. Gray, Inorg. Chem., 1973, 12, 2721–2726 CrossRef CAS.
- J. He, X. Li, D. Su, H. Ji and X. Zhang, J. Mater. Chem. A, 2016, 4, 5632–5638 RSC.
- A. Benad, F. Jurries, B. Vetter, B. Klemmed and R. Hubner, Chem. Mater., 2018, 30, 145–152 CrossRef CAS.
- N. Husing and U. Schubert, Angew. Chem., Int. Ed., 1998, 37, 23–45 CrossRef.
- C. Mandal, S. Donthula, R. Soni, M. Bertino, C. Sotiriou-Leventis and N. Leventis, J. Sol-Gel Sci. Technol. DOI:10.1007/s10971-018-4801-0.
- X. Li, Y. Jiao, H. Ji and X. Sun, Integr. Ferroelectr., 2013, 146, 122–126 CrossRef CAS.
- X. Hou, R. Zhang and B. Wang, Ceram. Interfaces, 2018, 44, 15440–15445 CrossRef CAS.
- T. Woignier, A. HafidiAlaoui, J. Primera and J. Phalippou, Key Eng. Mater., 2008, 391, 27–44 Search PubMed.
- R. Xiong, X. Li, H. Ji, X. Sun and J. He, J. Sol-Gel Sci. Technol., 2014, 72, 496–501 CrossRef CAS.
- Z. Hu, J. He, X. Li, H. Ji, D. Su and Y. Qiao, J. Porous Mater., 2017, 24, 657–665 CrossRef CAS.
- H. Zhang, X. Li, J. He, Z. Hu and H. Yu, Solid State Phenom., 2018, 281, 105–110 Search PubMed.
- Y. L. He and T. Xie, Appl. Therm. Eng., 2015, 81, 28–50 CrossRef CAS.
- J. He, X. Li, D. Su, H. Ji and X. J. Wang, J. Eur. Ceram. Soc., 2016, 36, 1487–1493 CrossRef CAS.
Footnote |
† Electronic supplementary information (ESI) available. See DOI: 10.1039/c8ra08263d |
|
This journal is © The Royal Society of Chemistry 2018 |
Click here to see how this site uses Cookies. View our privacy policy here.