DOI:
10.1039/C8RA08358D
(Paper)
RSC Adv., 2018,
8, 39854-39864
A colorimetric and fluorescent chemosensor for Hg2+ based on a photochromic diarylethene with a quinoline unit
Received
9th October 2018
, Accepted 20th November 2018
First published on 28th November 2018
Abstract
A new colorimetric and fluorescent ‘on–off’ chemosensor, 1O, based on a photochromic diarylethene with a quinoline unit was designed and synthesized. The chemosensor 1O demonstrated selective and sensitive detection of Hg2+ ions in the presence of other competitive metal ions in acetonitrile. The stoichiometric ratio of the sensor 1O for Hg2+ was determined to be 1
:
1, and the limit of detection of the probe 1O was calculated to be 56.3 nM for Hg2+. In addition, a molecular logic circuit with four inputs and one output was successfully constructed with UV/vis light and metal-responsive behavior. ESI-MS spectroscopy, Job's plot analysis, and 1H NMR titration experiments confirm the binding behavior between 1O and Hg2+.
1. Introduction
Nearly all heavy metals are toxic to human health and dangerous for the environment. In particular, Hg2+ is one of the major and most deadly heavy metal ions,1–3 and is widely distributed in the water, air, and soil of everyday living environments.4,5 The accumulation of mercury even at low concentrations can cause many deleterious effects,6,7 including DNA damage, movement disorders, mitosis impairment, and serious damage to the nervous and endocrine organ systems.8–15 Many methods to measure Hg2+ have been reported, including atomic absorption spectrometry (AAS), electrochemical sensing, inductively coupled plasma optical emission spectrometry (ICP-OES), voltammetry, and more.16–20 However, these traditional methods have some common disadvantages, such as expensive specialized equipment, complicated sample preparation, and long assay times.21–23 Thus, it is important to develop a facile, rapid, sensitive, and easy to use method for sensing mercury ions even at low concentrations.
Optical methods, colorimetric or fluorometric, are preferred methods owing to their operational simplicity, real time monitoring, high selectivity and sensitivity, and low detection limits.24–31 In addition, optical probes for the determination of transition metal cations are popular because they can facilitate analyte detection in solutions by the naked eye32,33 as well as in living cells by microscopy.34–36 Actually, a lot of fluorescent sensors have been designed for the detection of Hg2+, such as rhodamine,37 oligothiophene,38 pyrazoline,39 coumarin.40 Unfortunately, most of these sensors for the detection of Hg2+ still have weak points, such as synthetic difficulty, complex molecular structure, poor selectivity and high detection limit. Therefore, it is necessary to design and develop efficient and convenient Hg2+ sensors for their promising application potential in the environment.
Diarylethene derivatives, one of the most promising photo-switchable dyes have attracted considerable research interest due to their excellent photo reactivity, thermal stability, and fatigue resistance.41–44 They can be used to construct photoswitches, logic circuits, optical memories, and chemosensors.45–49 So far many diarylethene derivatives have been reported as optical sensors, such as diarylethene attach to 1-butyl-2-oxo-1,2-dihydroquinoline,50 thieno-imidazole,51 chromone,52 2-hydrazinopyridine,53 1,8-naphthyridin,54 rhodamine 6G.55 However, most of these diarylethene derivatives are designed for detection of Zn2+, Al3+, Cd2+ with Schiff base structure, and the reported sensing methods combining the colorimetric and fluorometric are relatively sparsely, especially with a quinoline group. Meanwhile, to our knowledge, Schiff base is unstable.56 Thus, it is still highly desirable to design sensitive and stable sensors for Hg2+ detection, which taking both advantages of colorimetric and fluorometric methods based on diarylethene with quinoline group.
Toward this goal, we describe herein a colorimetric and fluorescent chemosensor for Hg2+ based on photochromic diarylethene with a quinoline unit. The selectivity and sensitivity for Hg2+ were systematically investigated, and the results indicated that 1O exhibited changes in both absorbance and fluorescence upon exposure to Hg2+ ions over other competing metal ions. In addition, 1O could detect Hg2+ ions with a low detection limit (56.3 nM). Scheme 1 shows the synthetic route and photochromic process of 1O.
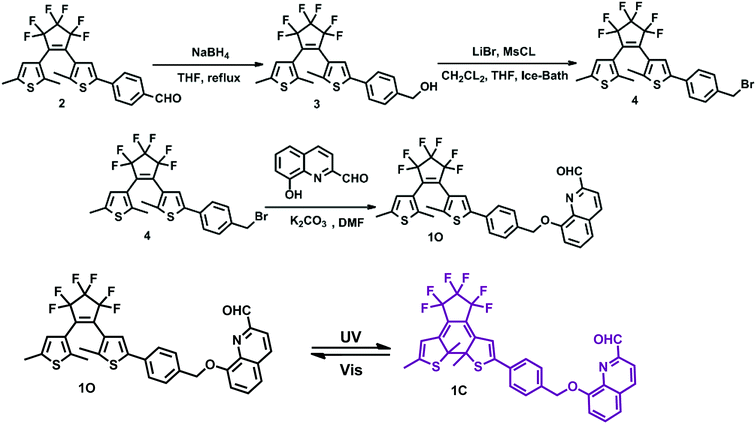 |
| Scheme 1 Scheme of the synthesis of diarylethene 1O and its photochromism. | |
2. Experiments
2.1. General methods
All solvents were of analytical grade and distilled before use. 8-Hydroxyquinoline was obtained from J & K Scientific Ltd and other chemical reagents were used as received. All metal ions expect for Ba2+ and K+ that were prepared with their chloride salts. Hg2+ was prepared with its high chloride salts, and the remainings were obtained by dissolving the corresponding metal nitrates (0.1 mmol) in distilled water (10 mL). An EDTA solution was prepared with ethylenediaminetetraacetic acid disodium salt (Na2EDTA) (1.0 mmol) in distilled water (10 mL).
1H NMR and 13C NMR spectra were recorded on a Bruker AV400 spectrometer (400 MHz) using CD3CL and CD3CN as solvents and tetramethylsilane (TMS) as the internal standard. Mass spectra were obtained using an Agilent 1100 Ion Trap LC/MS MSD system. Melting points were measured using a WRS-1B melting point apparatus. Infrared spectra (IR) were collected on a BrukerVertex-70 spectrometer. UV-vis absorption spectra were recorded on an Agilent 8453 UV/vis spectrophotometer equipped with an MUA-165 UV lamp and MVL-210 visible lamp for photoirradiation. Fluorescence spectra were measured with a Hitachi F-4600 fluorescence spectrophotometer. Fluorescence quantum yield was measured using a QY C11347-11 absolute PL quantum yield spectrometer. Photoirradiation was performed on a setup consisting of a SHG-200 UV lamp, Cx-21 ultraviolet fluorescence analysis cabinet, and BMH-250 visible lamp. The synthetic route to the target compound 1O is shown in Scheme 1. Intermediate compounds 2 were prepared by the reported method.57
2.1.1 Synthesis of 1-(2,5-dimethyl-3-thienyl)-2-[2-methyl-5-(4-hydroxymethylphenyl)-3-thienyl]perfluorocyclopentene (3). 1.95 g, 4 mmol of compound 2 and 0.19 g, 4.8 mmol of NaBH4 were taken in 50 mL of THF in a 100 mL round bottom flask. The mixture was stirred and refluxed for 2 h. After the reaction completed, the reaction product was cooled to RT and quenched with 20 mL water. The product was extracted with dichloromethane, the organic phase was washed with water, dried over anhydrous Na2SO4, filtered, and rotary evaporated. Finally, the compound was purified by column chromatography (petroleum ether
:
ethyl acetate = 1
:
1) to obtain a white solid 1.84 g (3.76 mmol, 94%). Mp: 353–354 K; 1H NMR (DMSO, 400 MHz), δ (ppm): 1.843 (s, 3H, –CH3), 1.902 (s, 3H, –CH3), 2.293 (s, 3H, –CH3), 4.495 (t, 2H, –CH2–), 5.25 (s, 1H, –OH), 6.818 (s, 1H, Ar–H), 7.35 (d, 2H, Ar–H), 7.413 (s, 1H, Ar–H), 7.560 (d, 2H, Ar–H).
2.1.2 Synthesis of 1-(2,5-dimethyl-3-thienyl)-2-[2-methyl-5-(4-bromomethylphenyl)-3-thienyl]perfluorocyclopentene (4). To a stirred solution of 40 mL anhydrous CH2Cl2 in ice bath, compound 3 (1.47 g, 3.00 mmol), MsCl (0.39 mL, 4.80 mmol) and TEA (0.75 mL, 5.40 mmol) were added. After rapid stirring for ten minutes at 273 K, added 10 mL of anhydrous THF containing LiBr (1.03 g, 12.00 mmol) to the reaction flask. Keep this temperature and continue to stir for 30 minutes. Then, the solution was warmed to room temperature and stirred for 12 h. After reaction, the product was quenched with 20 mL water and evaporated of organic solvents. Extracted with dichloromethane, dried over anhydrous Na2SO4, filtered, and rotary evaporated. Finally, the product was purified by column chromatography (petroleum ether
:
ethyl acetate = 8
:
1) to obtain a white solid 1.48 g (2.68 mmol, 90%). Mp: 345–346 K; 1H NMR (DMSO, 400 MHz), δ (ppm): 1.556 (s, 3H, –CH3), 1.927 (s, 3H, –CH3), 2.412 (s, 3H, –CH3), 4.763 (d, 2H, –CH2–), 6.837 (s, 1H, Ar–H), 7.497 (d, 3H, Ar–H), 7.631 (t, 2H, Ar–H).
2.1.3 Synthesis of 1-(2,5-dimethyl-3-thienyl)-2-{2-methyl-5-phenyl-4-(2-formylquinoline-8-methoxyl)-3-thienyl}perfluorocyclopentene (1O). Compound 4 (0.55 g, 1 mmol) and 8-hydroxyquinoline-2-carboxaldehyde (0.19 g, 1.10 mmol) were added to anhydrous CH3CN (30 mL) containing K2CO3 (0.33 g, 2 mmol). The solution was stirred at 283 K for 48 h. The reaction mixture was filtered, and the solvent was removed under vacuum. The crude product was purified by column chromatography on silica gel using dichloromethane/ethyl acetate (v/v = 6/1) as the eluent to give the target compound 1O as a purple solid (0.42 g, 0.67 mmol) in 67% yield. Mp: 339–341 K. 1H NMR (400 MHz, CDCl3), δ (ppm): 1.88 (s, 3H, –CH3), 1.94 (s, 3H, –CH3), 2.45 (s, 3H, –CH3), 5.53 (s, 2H, –CH2–), 6.76 (s, 1H, Ar–H), 7.18 (d, 1H, Ar–H), 7.53 (t, 2H, Ar–H), 5.9 (t, 5H, Ar–H), 8.12 (d, 1H, Ar–H), 8.32 (d, 1H, Ar–H), 10.36 (s, 1H, –CHO); 13C NMR (400 MHz, CDCl3) δ (ppm): 14.34 (–CH3), 15.07 (–CH3), 29.69 (–CH3), 70.81 (–CH2–), 111.20, 117.92, 120.15, 122.76, 124.68, 125.86, 127.75, 129.56, 131.43, 133.21, 135.31, 136.26, 137.28, 137.78, 139.78, 140.38, 141.26, 141.54, 151.70, 155.13, 193.82; IR (KBr, v, cm−1): 1272 (–C–O), 1704 (–CHO). Anal. calcd for C33H23F6NO2S2: C, 61.58; H, 3.60; F, 17.71; N, 2.18; O, 4.97; S, 9.96; found: C, 61.53; H, 3.65; F, 17.74; N, 2.23; O, 4.91; S, 9.93. MS (m/z): Calculated for C33H23F6NO2S2 [M]+: 643.11, found: 666.0972 for [1O + Na]+ and 698.1233 for [1O + 3H2O + H]+.
3. Results and discussion
3.1. Photochromic and fluorescent properties of diarylethene 1O
The photochromic behavior of diarylethene 1O induced by UV/vis light was monitored in acetonitrile (C = 2.0 × 10−5 mol L−1) at room temperature. As shown in Fig. 1a, diarylethene 1O displayed a sharp absorption band at 261 nm (εmax = 4.9 × 104 mol−1 L cm−1), which is assigned to the π–π* transition. Upon irradiation with 297 nm light, the absorption band at 279 nm was decreased significantly. A new absorption band centered at 557 nm (εmax = 0.9 × 104 mol−1 L cm−1) appeared and the color of the solution changed from colorless to purple due to the formation of ring-closed isomer 1C.58,59
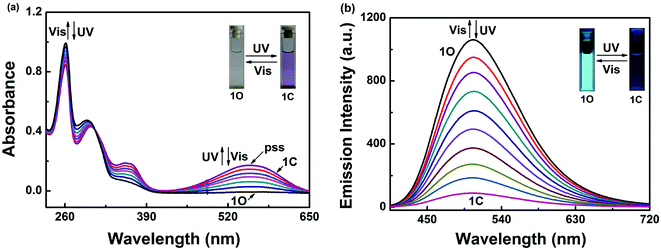 |
| Fig. 1 Upon irradiation with UV-vis light: (a) absorption spectra changes of 1O in acetonitrile (2.0 × 10−5 mol L−1); (b) fluorescence spectra changes of 1O in acetonitrile (2.0 × 10−5 mol L−1). Excitation: 370 nm. | |
This photocyclization reaction reached the photostationary state (PSS) after 3 min of irradiation with UV light, where a clear isosbestic point was observed at 308 nm. These observations suggest that the process is a two-component photochromic reaction. In addition, the purple solution of 1C could be completely bleached upon irradiation with visible light (λ > 500 nm) and the absorption spectrum returned back to the initial state 1O. The cyclization and cycloreversion quantum yields were calculated to be 0.533 and 0.023, respectively, with 1,2-bis(2-methyl-5-phenyl-3-thienyl)-perfluorocyclopentene as the reference.
Fig. 1b shows the fluorescence emission spectra of 1O in acetonitrile (2.0 × 10−5 mol L−1) under photoirradiation at room temperature. A strong fluorescence peak of 1O centered at 506 nm was observed under excitation at 370 nm. Upon irradiation with 297 nm UV light, the compound 1O showed notable fluorescent switching properties, where the emission peak of 1O decreased significantly and the solution color changed from bright cyan to dark. In the photostationary state, the fluorescence was 92% quenched due to the formation of the non-fluorescent ring closed isomer 1C. The weak fluorescence may be due to incomplete cyclization. The fluorescence quantum yield of 1O to 1C was determined to be 0.245 to 0.021. Back irradiation with appropriate visible light (λ > 500 nm) regenerated the open-ring isomer 1O and restored the initial fluorescence.
3.2. Absorption response of 1O to Hg2+
Colorimetric sensing behavior of 1O was examined by UV-vis in acetonitrile (2.0 × 10−5 mol L−1) at room temperature. As shown in Fig. 2, compound 1O was investigated in the presence of various metal ions, including Cu2+, Hg2+, Ni2+, Co2+, Fe3+, Cr3+, Al3+, Zn2+, Cd2+, Mg2+, Sr2+, K+, Ba2+, Mn2+, Ca2+, Pb2+ and Ag+. Of all of these ions, only Hg2+ caused a modest color change from colorless to yellow (Fig. 2c). The UV-vis spectra showed that Hg2+ addition to the sensor 1O resulted in a 15 nm bathochromic shift from 261 nm to 275 nm, at the same time, a broad absorption band appeared in the visible region. These changes were ascribed to ligand-to-metal charge transfer (LMCT).60–62 However, the other metal ions did not cause the absorption peak red shift and no new absorption band could be produced in the visible range (Fig. 2a). Fig. 2b shows the absorbance ratio (A275 nm/A261 nm) of 1O upon addition of each ion, which also exhibited high selectivity of 1O for Hg2+ ions. To explore the practical applications of 1O in Hg2+ detection and to estimate the selectivity of 1O for Hg2+, competitive experiments were conducted with the metal ions listed above. As depicted in Fig. 2d, other competitive metal ions did not interfere with the recognition of Hg2+ ions, confirming the selectivity of the sensor 1O for Hg2+ ions over other metal ions.
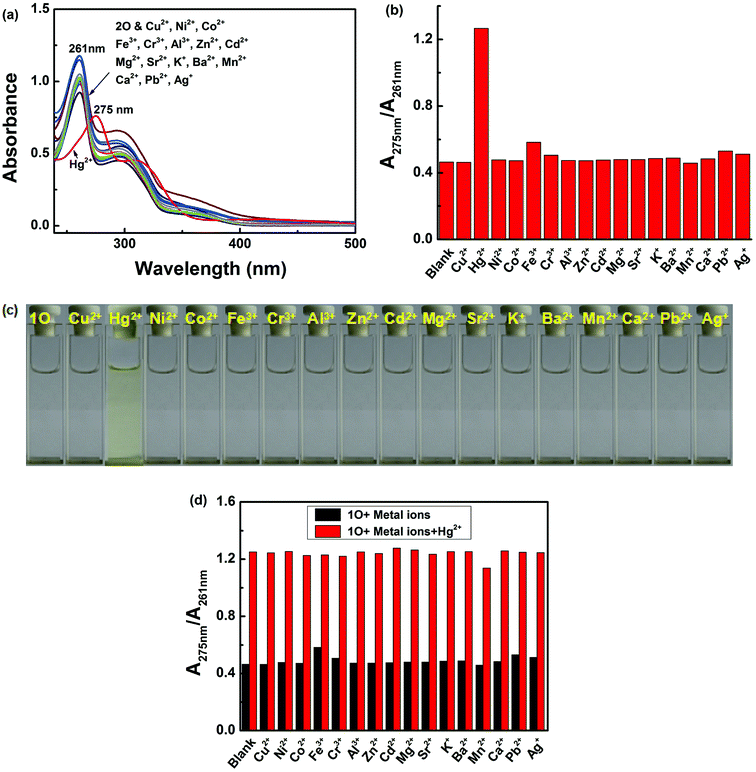 |
| Fig. 2 Changes in the absorption of 1O induced by various metal ions (1.0 equiv.) in acetonitrile (2.0 × 10−5 mol L−1). (a) UV/vis absorption spectra of 1O in the presence of different metal ions; (b) corresponding absorbance ratios (A275 nm/A261 nm) of 1O in the presence of different metal ions; (c) photos demonstrating the color of 1O solutions containing different metal ions; (d) competitive tests for the absorption response of 1O in the presence of Hg2+ and other competing metal ions in acetonitrile. Black bars represent the absorption of 1O solution in the presence of 1.1 equiv. metal ions and red bars represent the absorptions of 1O solutions containing the metal ions and Hg2+ (1.0 equiv.). | |
Fig. 3 shows the absorption spectral and color changes of 1O in acetonitrile (2.0 × 10−5 moL L−1) induced by the stimulation of Hg2+/EDTA and UV/vis. As depicted in Fig. 3a, the first absorption band around 341 nm gradually reduced and a new absorption band at 275 nm obviously increased with the sequential addition of Hg2+ amount. It reached the maximum at 1.0 equiv. of Hg2+, and a notable color change from colorless to yellow was apparent from bare eye. Further addition of Hg2+ did not change the absorption spectrum, suggesting that the 1O + Hg2+ complex was formed. The absorbance intensity at 261 and at 275 nm exhibited a good linear relationship with the Hg2+ in the range of 0–1.0 equiv. (Fig. 3b).
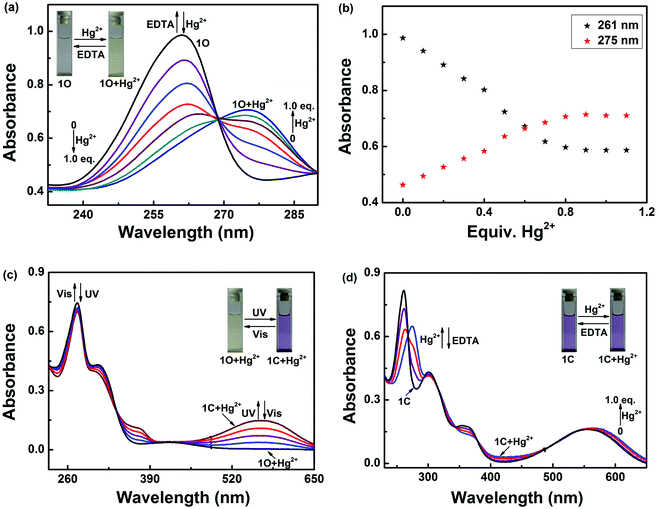 |
| Fig. 3 Changes in the absorption spectrum and color of 1O (2.0 × 10−5 moL L−1) in acetonitrile induced by Hg2+/EDTA and light stimuli: (a) UV/vis spectra of 1O induced by Hg2+/EDTA; (b) absorbance at 261 and 275 nm versus the concentration of Hg2+; (c) UV/vis spectra of 1O + Hg2+ induced by UV/vis light; (d) UV/vis spectra of 1C induced by Hg2+/EDTA. | |
Compound 1O + Hg2+ could also undergo photoisomerization upon alternating irradiation with UV and visible light. Upon irradiation with 297 nm UV light, the yellow solution of 1O + Hg2+ turned purple and a new absorption band centered at 532 nm (εmax = 7.4 × 103 moL−1 L cm−1) emerged due to the formation of the closed-ring isomer 1C + Hg2+ (Fig. 3c). In addition, 1C + Hg2+ also could be formed by reacting 1C with Hg2+ (Fig. 3d). It was noticed that the absorption properties of 1O and 1C could be restored by adding excess EDTA to the solutions containing 1O + Hg2+ and 1C + Hg2+, respectively.
3.3. Fluorescence response of 1O to Hg2+
As shown in Fig. 4, after the addition of 2.1 equiv. of different metal ions Cu2+, Hg2+, Ni2+, Co2+, Fe3+, Cr3+, Al3+, Zn2+, Cd2+, Mg2+, Sr2+, K+, Ba2+, Mn2+, Ca2+, Pb2+ and Ag+, no significant changes in fluorescence intensity were observed except for in case of Hg2+, where an emission band peak at 506 nm exhibited quenching (Fig. 4a). Compared with that of 1O, the fluorescence intensity of the complex 1O + Hg2+ was reduced to 97% at 506 nm (Fig. 4b). Simultaneously, Hg2+ could make the color of the 1O solution “switched-off” from a fluorescent bright cyan to a non-fluorescent color, no obvious color changes could be observed in the presence of other metal ions(Fig. 4c). These results suggest that 1O is highly selective towards Hg2+. To explore practical applicability of 1O in Hg2+ detection, competitive experiments were performed in the presence of the above-mentioned metal ions (Fig. 4d). It was observed that the fluorescence quenching of 1O + Hg2+ was not affected by the presence of other metal ions (2.1 equiv.) in acetonitrile, indicating the high binding affinity of 1O for Hg2+.
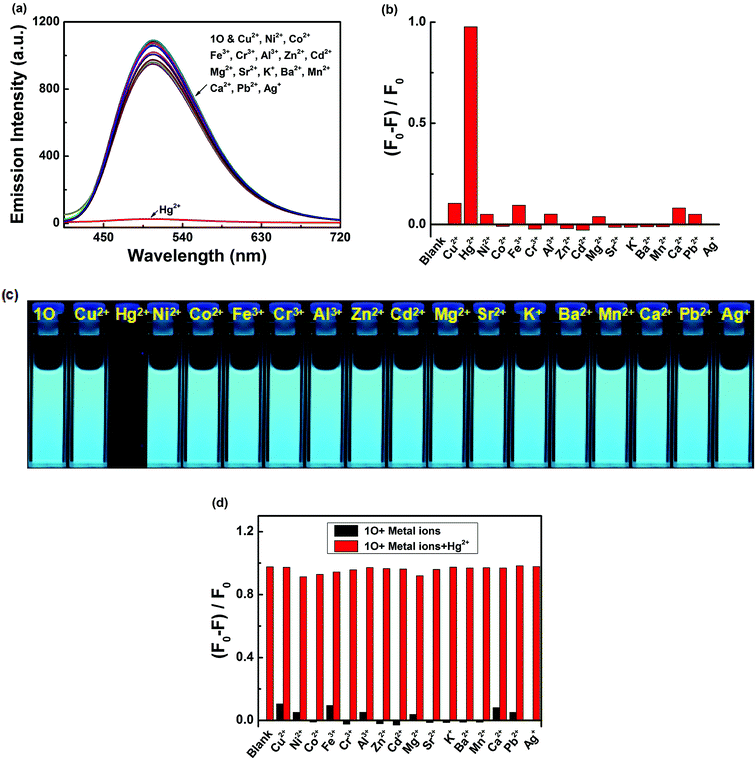 |
| Fig. 4 Fluorescence responses of 1O (2.0 × 10−5 mol L−1) to various metal ions (2.1 equiv.) in acetonitrile. (a) Emission spectra of 1O in the presence of different metal ions; (b) corresponding maximum emission intensities of 1O in the presence of the metal ions; (c) photos demonstrating the colors of 1O solutions containing different metal ions; (d) competitive tests for the fluorescent response of 1O in the presence of Hg2+ and other competing metal ions in acetonitrile. Black bars represent the fluorescence of 1O solution in the presence of 2.1 equiv. metal ions and red bars represent the fluorescence of 1O solutions containing the metal ions and Hg2+ (2.1 equiv.). | |
Meanwhile, changes in fluorescence of 1O induced by Hg2+/EDTA were performed in acetonitrile (2.0 × 10−5 mol L−1) at room temperature, and the results are shown in Fig. 5. A decline in emission intensity at 506 nm was noted for 1O when the Hg2+ concentration increased from 0 to 2.1 equiv., accompanied by a notable fluorescent color change from bright cyan to dark and followed by a plateau upon further titration. Notably, the fluorescence of 1O was successfully restored with the addition of 2.1 equiv. of EDTA. In addition, the fluorescence quantum yield of 1O + Hg2+ was determined to be 0.008, 29.6 folds lower than that of 1O (Φf, 1O = 0.245), suggesting that the diarylethene 1O could also be potentially used as a fluorescent probe of Hg2+.
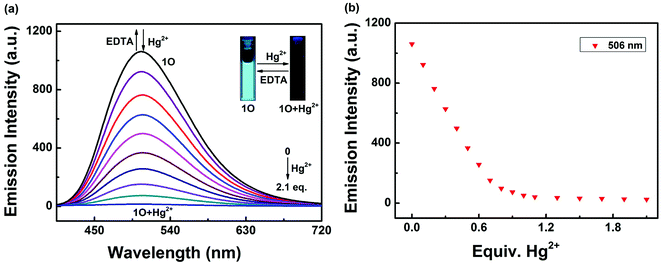 |
| Fig. 5 Changes in the fluorescence of 1O in acetonitrile (2.0 × 10−5 mol L−1) induced by Hg2+/EDTA, excited at 370 nm: (a) fluorescence emission spectra of 1O titrated with different amounts of Hg2+; (b) the fluorescence intensities of 1O at 506 nm in the presence of different equiv. Hg2+. | |
The binding stoichiometry of 1O and Hg2+ was calculated from the modified Hildebrand–Benesi equation. As shown in Fig. 6a, the lowest concentration of 1O + Hg2+ was achieved at [Hg2+]/([Hg2+] + [1O]) = 0.5, indicating that 1O was bound to Hg2+ in a binding stoichiometry of 1
:
1. The association constant (Ka) of 1O with Hg2+ was calculated to be 4.011 × 104 L mol−1 (R = −0.98696) (Fig. 6b). The limit of detection of 1O for Hg2+ was measured to be 56.3 nM (Fig. 6c), lower than those of other Hg2+ sensors reported in literature, suggesting that 1O could be used as a highly sensitive fluorescence sensor of Hg2+. The 1
:
1 coordination stoichiometry of 1O with Hg2+ was further confirmed by the ESI mass spectrometry (ESI-MS) analysis. 1O exhibited a characteristic peak at 666.0972 m/z for [1O + Na]+ (calcd 666.11) and 698.1233 m/z for [1O + 3H2O + H]+ (calcd 698.11), which disappeared as 2.1 equiv. of Hg2+ were added, accompanied by the appearance of a new peak at 876.0972 m/z for [1O + Hg2+ + CH3OH–H]+ (calcd 876.10) (Fig. 6d).
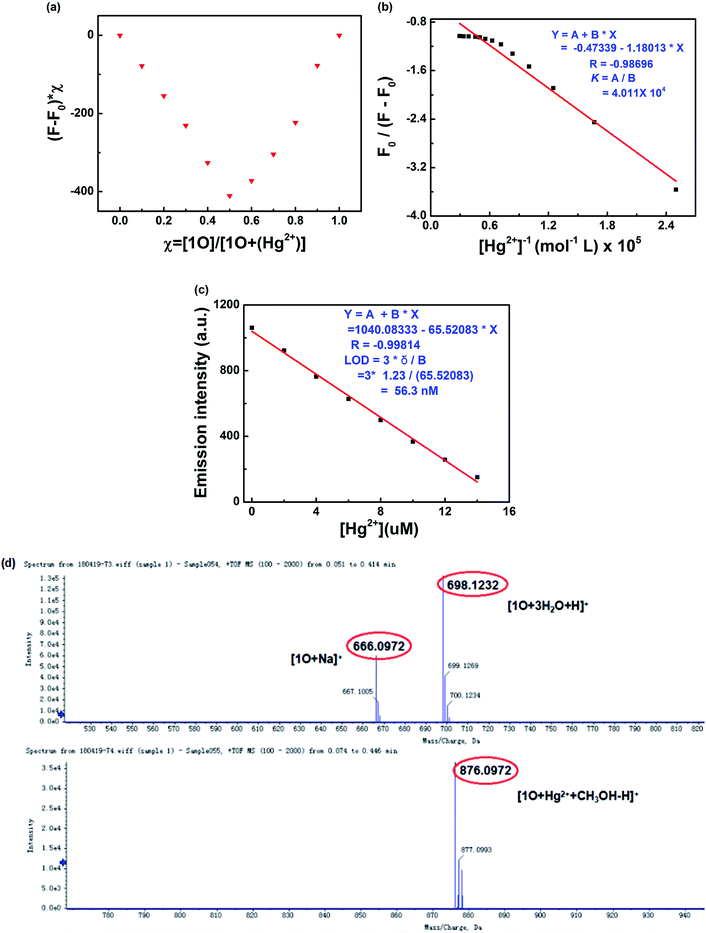 |
| Fig. 6 (a) Job's plot showing the 1 : 1 complex of 1O with Hg2+; (b) Hildebrand–Benesi plot based on the 1 : 1 binding stoichiometry. The binding constant of 1O with Hg2+ was calculated to be 4.011 × 104 L mol−1; (c) the limit of detection (LOD was estimated to be 18.5 nM); (d) ESI-MS spectrum of 1O and 1O + Hg2+. | |
Furthermore, 1H NMR titrations verified the binding mode of 1O with Hg2+. As shown in Fig. 7, the double peaks at 7.353, 7.371 ppm belonging to Ha gradually shifted to 7.367 and 7.385 ppm, respectively. In addition, the proton signal of Hb at 7.617 ppm and Hc at 7.680 ppm reduced with the addition of Hg2+. Therefore, we deduced that one oxygen atom close to methylene, and the nitrogen atom of quinolone together participated in binding with a Hg2+ ion, supporting that 1O was an “O–N” type ligand. Similar phenomena also observed in other reported probes.63 Based on the UV-vis and fluorescence titration experiments, Job's plot, ESI-MS, 1H NMR titrations analysis, the proposed structure of 1O + Hg2+ is shown in Scheme 2.
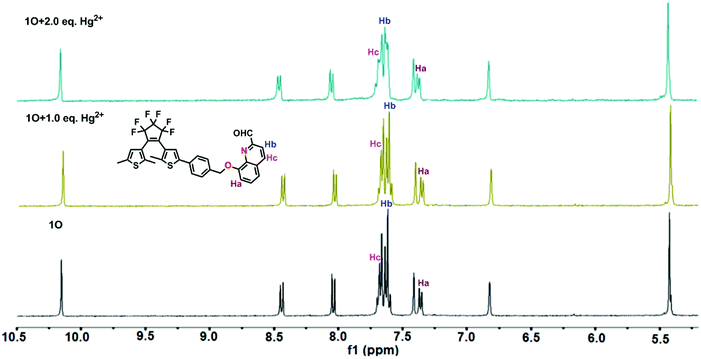 |
| Fig. 7 1H NMR spectral changes in deuterated acetonitrile with the increasingly addition of Hg2+. | |
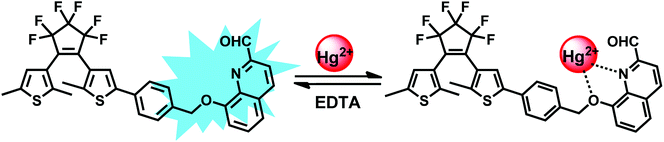 |
| Scheme 2 The binding mode between 1O and Hg2+. | |
3.4. pH effects
In order to study practical applicability, the pH effect on the fluorescence intensity changes of the 1O solution in the absence and presence of 2.1 equiv. of Hg2+ was evaluated. As shown in Fig. 8, when the pH value was lower than 4, it showed the fluorescence emission was significantly reduced, owing to the protonation of quinoline unit.64 When the pH value range from 4 to 10, no apparent changes of the fluorescence intensity were observed for 1O. Coincidentally, the 1O + Hg2+ complex was also not sensitive to pH in the range of 4–10, and at the same time, we can see the big gaps between the fluorescence intensity of 1O and 1O + Hg2+ within this pH range. Therefore, suggesting that sensor 1O was stable at biological conditions and could detect Hg2+ in a relatively wide pH range (4 to 10).
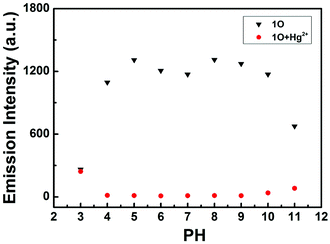 |
| Fig. 8 Changes in the fluorescence intensity of sensor 1O at 506 nm with and without Hg2+ at different pH values (λex = 370 nm). | |
3.5. Application in logic circuits
As mentioned above, the fluorescence of 1O can be independently modulated by exposure to UV/vis light and to Hg2+/EDTA. Based on these properties, a combinatorial logic circuit can be constructed using the stimulation of light irradiation (In1: 297 nm light and In2: λ > 500 nm visible light) and chemical compounds (In3: Hg2+ and In4: EDTA) as four input signals, and the fluorescence intensity at 506 nm as the output signal (Fig. 9). The output signal could serve as ‘on’ with a Boolean value of ‘1’ when fluorescence intensity was quenched to ca. 97%, otherwise defined as ‘off’ with a Boolean value of ‘0’. For example, inputs of ‘0, 0, 1 and 0’, corresponding to In1, In2, In3 and In4, result in an output of ‘off, off, on and off’. Under these conditions, 1O was converted to 1O + Hg2+ by stimulation with Hg2+, and its emission intensity was quenched dramatically. As a result, the output signal is ‘on’ and the output digit is ‘1’. All possible logical combinations of the four inputs and their corresponding outputs are listed in Table 1.
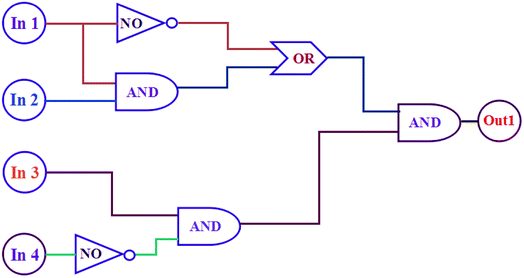 |
| Fig. 9 The combinatorial logic circuits to the truth table given in Table 1: In1 (297 nm light), In2 (λ > 500 nm visible light), In3 (Hg2+), In4 (EDTA) and output1 (fluorescence at 506 nm). | |
Table 1 Truth table for all possible strings of four binary-input data and the corresponding outputa
Input |
Output |
In1 (UV) |
In2 (Vis) |
In3 (Hg2+) |
In4 (EDTA) |
λem = 506 nm |
At 506 nm, when fluorescence is quenched to ca. 97%, is defined as 1. Otherwise, it is 0. |
0 |
0 |
0 |
0 |
0 |
1 |
0 |
0 |
0 |
0 |
0 |
1 |
0 |
0 |
0 |
0 |
0 |
1 |
0 |
1 |
0 |
0 |
0 |
1 |
0 |
1 |
1 |
0 |
0 |
0 |
1 |
0 |
1 |
0 |
0 |
1 |
0 |
0 |
1 |
0 |
0 |
1 |
1 |
0 |
1 |
0 |
1 |
0 |
1 |
0 |
0 |
0 |
1 |
1 |
0 |
1 |
1 |
1 |
0 |
1 |
1 |
1 |
0 |
1 |
0 |
1 |
0 |
1 |
1 |
0 |
0 |
1 |
1 |
1 |
0 |
1 |
1 |
1 |
1 |
0 |
4. Conclusion
In conclusion, we have developed a novel diarylethene-based colorimetric and on–off type fluorescent chemosensor 1O for the selective and sensitive sensing of Hg2+ in acetonitrile. The complexation of 1O with various metal ions was studied using UV-vis absorption and fluorescent emission spectra. Importantly, competition experiments suggested that the selective colorimetric and fluorescence quenching of Hg2+ is not affected by other metal ions. The detection limit of 1O towards Hg2+ was 56.3 nM. These results showed that probe 1O is an efficient sensor for detecting Hg2+. In addition, the absorption and fluorescence emission of 1O were efficiently adjusted by stimuli with light and chemical species. Based on these characteristics, an integrated logic circuit with multiple control switches was successfully designed and constructed.
Conflicts of interest
There are no conflicts of interest to declare.
Acknowledgements
The authors are grateful for the financial support from the National Natural Science Foundation of China (41867052, 21861017, 21662015, 41867053), the “5511” science and technology innovation talent project of Jiangxi, the key project of Natural Science Foundation of Jiangxi Province (20171ACB20025), the Science Funds of Natural Science Foundation of Jiangxi Province (20171BAB203014, 20171BAB203011).
References
- E. M. Nolan and S. J. Lippard, Chem. Rev., 2008, 108, 3443–3480 CrossRef CAS PubMed.
- T. Balaji, S. A. El-Safty, H. Matsunaga, T. Hanaoka and F. Mizukami, Angew. Chem., Int. Ed., 2006, 118, 7360–7366 CrossRef.
- G. Sener, L. Uzun and A. Denizli, Anal. Chem., 2014, 86, 514–520 CrossRef CAS PubMed.
- C. J. Wu, J. B. Wang, J. J. Shen, C. Bi and H. W. Zhou, Sens. Actuators, B, 2017, 243, 678–683 CrossRef CAS.
- Y. Zhang, J. J. Jiang, M. Li, P. F. Gao, L. H. Shi, G. M. Zhang, C. Dong and S. M. Shuang, Sens. Actuators, B, 2017, 238, 683–692 CrossRef CAS.
- G. Q. Chen, Z. Guo, G. M. Zeng and L. Tang, Analyst, 2015, 140, 5400–5443 RSC.
- E. M. Nolan and S. J. Lippard, Chem. Rev., 2008, 108, 3443–3480 CrossRef CAS PubMed.
- Y. Wang, F. Yang and X. R. Yang, Biosens. Bioelectron., 2010, 25, 1994–1998 CrossRef CAS PubMed.
- Y. L. Wang, Y. Y. Cui, R. Liu, F. P. Gao, L. Gao and X. Y. Gao, Sci. China: Chem., 2015, 58, 819–824 CrossRef CAS.
- J. B. S. Fretham, S. Caito, J. E. Martinez-Finley and M. Aschner, Toxicol. Res., 2012, 1, 132–138 RSC.
- P. S. Zhang, J. Li, B. W. Li, J. S. Xu, F. Zeng, J. Lv and S. Z. Wu, Chem. Commun., 2015, 51, 4414–4416 RSC.
- J. S. Lee, M. S. Han and C. A. Mirkin, Angew. Chem., Int. Ed., 2007, 46, 4096 Search PubMed.
- T. Sun, Q. F. Niu, Y. Li, T. D. Li and H. X. Liu, Sens. Actuators, B, 2017, 248, 24–34 CrossRef CAS.
- H. J. Huang, Y. Xu and X. Qian, J. Org. Chem., 2009, 74, 2167–2170 CrossRef PubMed.
- R. Singh and G. Das, Sens. Actuators, B, 2018, 258, 478–483 CrossRef CAS.
- H. Erxleben and J. Ruzicka, Anal. Chem., 2005, 77, 5124–5128 CrossRef CAS PubMed.
- Z. Q. Zhu, Y. Y. Su, J. Li, D. Li, J. Zhang, S. P. Song, Y. Zhao, G. X. Li and C. H. Fan, Anal. Chem., 2009, 81, 7660–7666 CrossRef CAS PubMed.
- M. Ozdemir, Sens. Actuators, B, 2017, 249, 217–228 CrossRef CAS.
- J. M. Gong, T. Zhou, D. D. Song, L. Z. Zhang and X. L. Hu, Anal. Chem., 2010, 82, 567–573 CrossRef CAS PubMed.
- M. P. N. Bui, J. Brockgreitens, S. Ahmed and a. Abbas, Biosens. Bioelectron., 2016, 85, 280–286 CrossRef CAS PubMed.
- S. C. Lopes Pinheiro, I. M. Raimundo Jr, M. C. Moreno-Bondi and G. Orellana, Anal. Bioanal. Chem., 2010, 398, 3127–3138 CrossRef CAS PubMed.
- M. Ozdemir, J. Photochem. Photobiol., A, 2016, 31, 7–13 CrossRef.
- M. G. K. Salmani, G. H. Rounaghi and M. Chamsaz, Sens. Actuators, B, 2018, 256, 968–975 CrossRef.
- G. Q. Chen, Z. Guo, G. M. Zeng and L. Tang, Analyst, 2015, 140, 5400–5443 RSC.
- M. H. Lee, J. S. Kim and J. L. Sessler, Chem. Soc. Rev., 2015, 44, 4185–4191 RSC.
- D. T. Quang and J. S. Kim, Chem. Rev., 2010, 110, 6280–6301 CrossRef CAS PubMed.
- K. P. Carter, A. M. Young and A. E. Palmer, Chem. Rev., 2014, 114, 4564–4601 CrossRef CAS PubMed.
- S. Y. Qin, B. Chen, J. Huang and Y. F. Han, New J. Chem., 2018, 42, 12766–12772 RSC.
- J. F. Zhang, Y. Zhou, J. Yoon and J. S. Kim, Chem. Soc. Rev., 2011, 40, 3416–3429 RSC.
- K. Ponnuvel, V. Padmini and R. Sribalan, Sens. Actuators, B, 2016, 222, 605–611 CrossRef CAS.
- K. Ponnuvel, M. Kumar and V. Padmini, Sens. Actuators, B, 2016, 227, 242–247 CrossRef CAS.
- J. M. Jung, C. Kim and R. G. Harrison, Sens. Actuators, B, 2018, 255, 2756–2763 CrossRef CAS.
- Y. Zhao, L. L. Cui and Z. B. Chen, Sens. Actuators, B, 2017, 241, 262–267 CrossRef CAS.
- H. Wang, P. S. Zhang, J. Chen, Y. Li, M. L. Yu, Y. F. Long and P. G. Yi, Sens. Actuators, B, 2017, 242, 818–824 CrossRef CAS.
- S. M. Xu, Y. Liu, H. Yang, K. Zhao, J. G. Li and A. P. Deng, Anal. Chim. Acta, 2017, 964, 150–160 CrossRef CAS PubMed.
- L. Yang, Y. A. Su, Y. N. Geng, H. Q. Xiong, J. L. Han, Q. Fang and X. Z. Song, Org. Biomol. Chem., 2018, 16, 5036–5042 RSC.
- K. S. Min, R. Manivannan and Y. A. Son, Sens. Actuators, B, 2018, 261, 545–552 CrossRef CAS.
- T. Sun, Q. F. Niu, Y. Li, T. D. Li and H. X. Liu, Sens. Actuators, B, 2017, 248, 24–34 CrossRef CAS.
- E. Bozkurt and H. I. Gul, Sens. Actuators, B, 2018, 255(1), 814–825 CrossRef CAS.
- C. J. Wu, J. B. Wang, J. J. Shen, C. Bi and H. W. Zhou, Sens. Actuators, B, 2017, 243, 678–683 CrossRef CAS.
- M. Irie, T. Fukaminato and K. Matsuda, Chem. Rev., 2014, 114, 12174–12277 CrossRef CAS PubMed.
- W. L. Li, X. Li, Y. S. Xie, Y. Wu, M. Q. Li and X. Y. Wu, Sci. Rep., 2015, 5, 9186 CrossRef CAS PubMed.
- G. T. Xu, B. Li, J. Y. Wang, D. B. Zhang and Z. N. Chen, Chem.–Eur. J., 2015, 21, 3318–3326 CrossRef CAS PubMed.
- B. Li, H. M. Wen, J. Y. Wang, L. X. Shi and Z. N. Chen, Inorg. Chem., 2015, 54, 11511–11519 CrossRef CAS PubMed.
- J. J. He, J. X. He, T. T. Wang and H. P. Zeng, J. Mater. Chem. C, 2014, 2, 7531–7540 RSC.
- Y. L. Fu, C. B. Fan, G. Liu and S. Z. Pu, Sens. Actuators, B, 2017, 239, 295–303 CrossRef CAS.
- S. J. Xia, G. Liu and S. Z. Pu, J. Mater. Chem. C, 2015, 3, 4023–4029 RSC.
- Y. Yamazaki, A. Sekine and H. Uekusa, Cryst. Growth Des., 2017, 17, 19–27 CrossRef CAS.
- R. J. Mart and R. K. Allemann, Chem. Commun., 2016, 52, 12262–12277 RSC.
- E. T. Feng, Y. Y. Tu, C. B. Fan, G. Liu and S. Z. Pu, RSC Adv., 2017, 7, 50188 RSC.
- Y. M. Xue, R. J. Wang, C. H. Zheng, G. Liu and S. Z. Pu, Tetrahedron Lett., 2016, 57, 1877–1881 CrossRef CAS.
- E. T. Feng, C. B. Fan, N. S. Wang, G. Liu and S. Z. Pu, Dyes Pigm., 2018, 151, 22–27 CrossRef CAS.
- S. Y. Li, D. B. Zhang, J. Y. Wang, R. M. Lu and C. H. Zheng, Sens. Actuators, B, 2017, 245, 263–272 CrossRef CAS.
- X. X. Zhang, R. J. Wang, C. B. Fan, G. Liu and S. Z. Pu, Dyes Pigm., 2017, 139, 208–217 CrossRef CAS.
- H. T. Xu, H. C. Ding, G. Li, C. B. Fan, G. Liu and S. Z. Pu, RSC Adv., 2017, 7, 29827 RSC.
- Z. L. Shi, Y. Y. Tu, R. J. Wang, G. Liu and S. Z. Pu, Dyes Pigm., 2018, 149, 764–773 CrossRef CAS.
- R. J. Wang, S. Z. Pu, G. Liu and S. Q. Cui, Beilstein J. Org. Chem., 2012, 8, 1018–1026 CrossRef CAS PubMed.
- C. B. Fan, L. L. Gong, L. Huang, F. Luo, R. Krashina and X. F. Yi, et al., Angew. Chem., Int. Ed., 2017, 56, 7900–7906 CrossRef CAS PubMed.
- S. L. Guo, G. Liu, C. B. Fan and S. Z. Pu, Sens. Actuators, B, 2018, 266, 603–613 CrossRef CAS.
- Y. Y. Fu, H. X. Li and W. P. Hu, Eur. J. Org. Chem., 2007, 2007, 2459–2463 CrossRef.
- K. L. Zhong, X. Zhou, R. B. Hou, P. Zhou, S. H. Hou, Y. J. Bian, G. Zhang, L. J. Tang and X. H. Shang, RSC Adv., 2014, 4, 16612–16617 RSC.
- Q. Li, Y. Guo and S. J. Shao, Sens. Actuators, B, 2012, 171–172, 872–877 CrossRef CAS.
- L. L. Chang, Q. Gao, S. Liu, C. C. Hu, W. J. Zhou and M. M. Zheng, Dyes Pigm., 2018, 153, 117–124 CrossRef CAS.
- M. Amelia, M. Baroncini and A. Credi, Angew. Chem., Int. Ed., 2008, 47, 6240–6243 CrossRef CAS PubMed.
|
This journal is © The Royal Society of Chemistry 2018 |
Click here to see how this site uses Cookies. View our privacy policy here.