DOI:
10.1039/C8SC00688A
(Edge Article)
Chem. Sci., 2018,
9, 4444-4450
9,10-Azaboraphenanthrene-containing small molecules and conjugated polymers: synthesis and their application in chemodosimeters for the ratiometric detection of fluoride ions†
Received
10th February 2018
, Accepted 6th April 2018
First published on 9th April 2018
Abstract
The introduction of main group elements into conjugated scaffolds is emerging as a key route to novel optoelectronic materials. Herein, an efficient and versatile way to synthesize polymerizable 9,10-azaboraphenanthrene (BNP)-containing monomers by aromaticity-driven ring expansion reactions between highly antiaromatic borafluorene and azides is reported, and the corresponding conjugated small molecules and polymers are developed as well. The BNP-containing small molecules and conjugated polymers showed good air/moisture stability and notable fluorescence properties. Addition of fluoride ions to the BNP-based small molecules and polymers induced a rapid change in the emission color from blue to green/yellow, respectively, accompanied by strong intensity changes. The conjugated polymers showed better ratiometric sensing performance than small molecules due to the exciton migration along the conjugated chains. Further experiments showed that the sensing process is fully reversible. The films prepared by solution-deposition of BNP-based compounds in the presence of polycaprolactone also showed good ratiometric sensing for fluoride ions.
1 Introduction
The fluoride anion (F−) has recently received well-deserved attention from the scientific community not only because of its importance in many fields of human activities, including toothpaste, vitamins, and dietary supplements but also because of its effect on the environment.1–4 As a result, many optical sensors have been developed for detecting F−. The construction strategies are generally based on the strong molecular interactions between sensors and F−, including H-bonding, F−-mediated chemical reactions and Lewis acid–base interactions. However, sensors based on hydrogen bonding5–10 and chemical reactions11,12 suffer from several drawbacks, such as a false positive signal or low sensitivity and long response time. In contrast, sensors based on the interactions between fluoride ions and Lewis acids have been regularly employed for the sensing of fluoride ions due to their superior sensitivity, simplicity, and reversibility.
As a class of typical Lewis acids, organoboranes with intrinsic Lewis acidity can react with fluoride to afford fluoroborate anions efficiently.13–17 This reaction is traditionally described as an addition reaction, which takes advantage of the extended π-conjugation in tricoordinate organoboranes with the p-orbital of the boron center that can accept an electron pair from F−. However, a large number of organoboranes are air- and moisture-sensitive; thus, improving their air stability is a very important research topic in this field. A common strategy to construct stable organoboranes involves introducing a bulky aryl group onto the boron atom, but this method is challenging because of the tough synthesis, especially for boroles.18–22
Replacing the boron atom by a boron–nitrogen (BN) unit in aromatics has been demonstrated to be an alternative way to stabilize organoboranes (boroles). The BN derivatives not only display high stability and excellent luminescence properties23–26 but also show Lewis acidity on the boron center, which can easily react with Lewis bases, such as F−. This property is explained by the existence of the p-orbital on boron atoms in BN-containing conjugated heterocycles. Since Dewar and co-workers pioneered the synthesis of BN isosteres of simple polycyclic aromatic hydrocarbons (PAHs) and their derivatives in the 1960s, significant attention has been focused on this type of chemistry27,28 and fascinating BN derivatives have recently been developed by Liu,29,30 Jäkle,31,32 Braunschweig33,34 and other groups.35–37 Very recently, five-membered, unsaturated and highly antiaromatic boroles38 have been proven to react via 1,1- and 1,2-insertion reactions with a variety of substrates, such as carbon monoxide, alkynes and isocyanides, as well as with a ketone or an aldehyde to generate a number of aromatic six- or seven-membered heterocycles, which are not available by other methods.39–42 Interestingly, the efficient aromaticity-driven ring-opening strategy, first developed by Braunschweig's group, was also used by Martin's group to synthesize the desired BN derivatives with high yield through the reaction between boroles and azides.43–45 However, synthesis of novel BN-containing conjugated heterocycles through the ring-opening reaction is still rare. Evidently, exploring the scope of the ring-opening reaction to obtain new BN-containing molecules and extending the family of BN moiety-containing conjugated polymers is not only important for BN and polymer chemistry, but also meaningful for F− sensing and other optoelectronic applications.
Based on these considerations, herein, for the first time, we report a new strategy to synthesize polymerizable 9,10-azaboraphenanthrene (BNP)-based monomers by the aromaticity-driven ring-opening reaction between a borafluorene precursor and an azide, as well as the respective BNP-containing conjugated polymers (Scheme 1). Because of the existence of the p-orbital on boron atoms, these small molecules and polymers were successfully used as chemodosimeters for the ratiometric detection of F− with high selectivity and sensitivity.
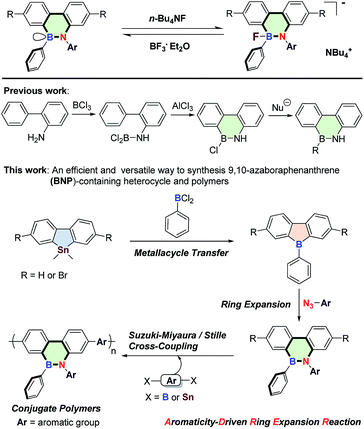 |
| Scheme 1 Aromaticity-driven ring expansion route to 9,10-azaboraphenanthrene-containing conjugated small molecules and polymers. | |
2 Results and discussion
2.1. Synthesis of BNP-containing small molecules and polymers
The highly antiaromatic borafluorene precursors 3 and 4 (NICS(1)zz: +26.0 for 3 and +24.8 for 4, Table S3†) were synthesized through boron–tin exchange reactions and were directly used for the ring-opening reactions without further separation and purification. The ring-opening reaction between borafluorene precursors (3 and 4) and 2-azido-1,3,5-trimethyl-benzene gave the desired aromatic BNP derivatives 5 and 6 (NICS(1)zz: −6.9 for 5 and −6.6 for 6, Table S3†) with 50% and 71% yield, respectively (Scheme 2). The successful synthesis of 5/6 was confirmed by multinuclear NMR, high-resolution mass spectrometry (HRMS), and elemental analysis (EA) (ESI†). The white crystals of 6 were obtained from saturated hexanes/CH2Cl2 mixtures, and were further analyzed by single-crystal X-ray crystallography. As shown in Fig. 1, the core rings of compound 6 had an almost planar conformation, and the B–N distance was 1.414(5) Å (i.e. this distance is shorter than the reported BN bond distances of (E)-2-mesityl-1-(2,6-dibromo-4-methylphenyldiazenyl)-3,4,5,6-tetraphenyl-1,2-azaborinine (1.443 Å)44,45 and (E)-6-phenyl-5-(phenyldiazenyl)-5,6-dihydrodibenzo[c,e]-[1,2]azaborinine (1.426 Å),46 Table S2†). The bond angles (deg) of N(1)–B(1)–C(1), N(1)–B(1)–C(14) and C(1)–B(1)–C(14) were 116.0(3)°, 121.8(3)° and 122.2(3)°, respectively, indicating a distorted trigonal planar environment around the boron center as expected for a B–N stable system. The mechanism of borole ring expansion by azides was revealed carefully by using experimental and theoretical methods by Martin and co-workers.43 According to this mechanism, the α-nitrogen of azide coordinates with the empty p orbital of the boron atom, followed by rearrangement to a bicyclic intermediate and generation of a kinetically favoured eight-membered heterocycle; alternatively molecular N2 is released to form the thermodynamically favoured BNP derivatives (Fig. S1†).
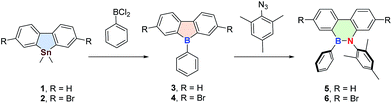 |
| Scheme 2 Synthesis of BNP derivatives 5 and 6. | |
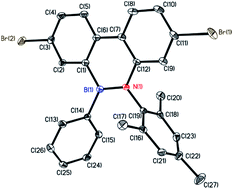 |
| Fig. 1 X-ray crystal structure of 6. Selected bond lengths (Å): N(1)–C(12), 1.412(4); N(1)–B(1), 1.414(5); N(1)–C(19), 1.452(5); B(1)–C(1), 1.551(6); and B(1)–C(14), 1.576(5). Bond angles (deg): C(12)–N(1)–B(1), 123.2(3); C(12)–N(1)–C(19), 116.1(3); B(1)–N(1)–C(19), 120.7(3); N(1)–B(1)–C(1), 116.0(3); N(1)–B(1)–C(14), 121.8(3); and C(1)–B(1)–C(14), 122.2(3). | |
Given the successful synthesis of 5 and 6, we were eager to explore Suzuki–Miyaura and Stille cross-coupling methodologies to extend the π-conjugation of these systems (Scheme 3). Firstly, model compound 8 was synthesized as a white solid in 52% yield by a Suzuki–Miyaura coupling reaction between monomer 6 and 4-methylphenylboronic acid (7), using Pd(PPh3)4 as a catalyst and Aliquat-336 as a phase-transfer agent. Similarly, the conjugated polymer P1 with a number-average molecular weight (Mn) of 6.17 kg mol−1 (PDI = 1.52, Fig. S3†) was successfully prepared by a Suzuki–Miyaura coupling reaction between 6 and 2,2′-(9,9-didecyl-9H-fluorene-2,7-diyl)bis(4,4,5,5-tetramethyl-1,3,2-dioxaborolane) (9). Moreover, monomer 6 was also tolerated under the reaction conditions of the Stille-coupling, and regiorandom polymer P2 (Mn of 6.08 kg mol−1 and PDI = 1.32, Fig. S4†) was successfully obtained through a reaction between 6 and (3-hexylthiophene-2,5-diyl)bis(tributylstannane) (10). The integrity of the 9,10-azaboraphenanthrene azaborine moieties in P1 and P2 was confirmed by broad signals in the 11B NMR spectra at δ = 34.0 ppm for P1 and δ = 33.7 ppm for P2, which were close to those of the monomers. Polymers P1 and P2 are soluble in typical organic solvents of medium polarity, such as DMF, THF, toluene, CH2Cl2 and CHCl3, which can be readily processed into thin films via a spin-coating method for future applications.
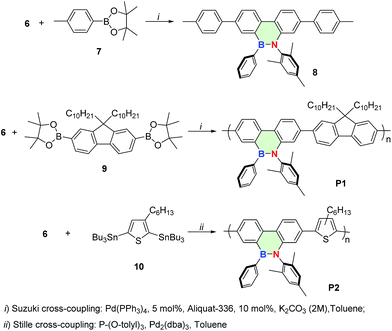 |
| Scheme 3 Synthesis of the BNP-containing molecule 8 and polymers P1 and P2 by the Suzuki–Miyaura or Stille cross-coupling. | |
2.2. Photophysical properties of the BNP-containing small molecules and polymers
Phenanthrene is a well-known fluorophore and is widely used as a conjugated component in luminescent organic materials.47–50 In comparison with the photophysical and electrochemical properties of phenanthrene, a BN–phenanthrene-containing heterocycle provided a clear-cut view of the characteristic properties.51,52 The lowest-energy absorption peaks of 5 showed major absorption bands at λmax = 317 and 329 nm, while its emission was observed at λmax,em = 355 nm (Fig. S5†). Due to its extended π-conjugation, the absorption and emission spectra of 8 showed maxima at 340 nm and 391 nm, respectively, which were slightly bathochromically shifted compared to 5 (Fig. S7†). The lowest energy absorption bands in 5 and 8 were attributed to lower symmetry in the heterocycle vs. the phenyl ring.53,54 The fluorescence spectra of 5 and 8 exhibited emission features with similar lifetimes (τ = 3.1 ns for 5 and 2.2 ns for 8) and high quantum yields (Φ = 0.52 for 5 and 0.77 for 8). In comparison to 5 and 8, polymers P1 and P2 exhibited a notable red-shift in UV-vis absorption and fluorescence spectra as a result of their increased aromatic chain linkage. While P1 showed the maximum absorption at λmax = 377 nm, P2 absorbed at λmax = 399 nm, the latter being ca. 20 nm red-shifted compared with P1. When excited at λex = 350 nm, polymers P1 and P2 displayed blue fluorescence at λmax,em = 414 (P1) and 458 nm (P2). However, the quantum yield of P1 (Φ = 0.88) is about 1.5-fold higher than that of P2 (Φ = 0.57).
2.3. Redox properties of the 9,10-azaboraphenanthrene-containing small molecules and polymers
The electron-acceptor properties of the small molecules and polymers were studied by cyclic voltammetry in DCM solution in a glovebox. As shown in Fig. S17,† an irreversible oxidation at around +0.4 to +1.0 V vs. Fc/Fc+ was found for the BNP-containing small molecules and polymers (5, E1/2 = 0.95 V; 8, E1/2 = 0.84 V; P1, E1/2 = 0.47 V; and P2, E1/2 = 0.44 V). Accordingly, the highest occupied molecular orbital (HOMO) energy levels of 5, 8, P1 and P2 were determined to be −5.75 eV, −5.64 eV, −5.27 eV and −5.24 eV, respectively; these data are consistent with the relationship between the extension of the π-conjugation and the increase in HOMO energy levels. The LUMO levels, calculated from the HOMO levels and the optical band gaps (5, 3.69 eV; 8, 3.39 eV; P1, 3.04 eV; and P2, 2.85 eV), were ca. −2.06, −2.25, −2.23 and −2.39 eV (Fig. 2), which are in good accordance with the calculation results (Table S4 and Fig. S18–S21,† calculated in the gas phase; Fig. S22–S25,† calculated in DCM). Compared to boroles,22,55 the redox waves of boron–nitrogen (BN) units showed significant differences, implying that BN substitution changed the electronic structure of the compound significantly. Notably, both B and N atoms contribute to the molecular orbitals to a large extent, thus being responsible for the altered electronic properties.
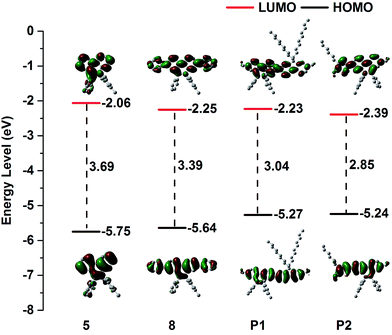 |
| Fig. 2 Experimental orbital energy levels and HOMO/LUMO orbital plots of 5, 8, P1 and P2. | |
2.4. Ratiometric fluoride-ion sensing properties of the BNP-containing small molecules and polymers
Owing to the presence of the empty p-orbital, tricoordinate organoboranes are known to accommodate a lone pair of electrons from a Lewis base, which will strongly change the optoelectronic properties. Based on this mechanism, many optical sensors for F− ions were developed. Considering the existence of the p-orbital on boron in the BNP-containing small molecules and polymers, we decided to investigate the response of these compounds to fluoride anions by UV-vis and fluorescence titration experiments, which were carried out using a solution of n-tetrabutylammonium fluoride (TBAF). Changes in the absorptions and fluorescence spectra of compounds 5, 8, P1 and P2 were compared in THF (Fig. 3). Upon addition of aliquots of fluoride to a solution of 5, a gradual decrease in intensity of the absorption bands at λ = 317 nm and 329 nm was observed, and a gradually increasing red-shift at λ = 372 nm was observed as a result of the binding of fluoride to the boron atom of the BN moiety (Fig. 3a). Similarly, fluoride addition to 8 led to a new absorption band at λ = 411 nm, which was a red-shifted by ca. 39 nm relative to the maximum absorption observed in 5 (Fig. 3c). These phenomena were well supported by the calculation results (Fig. S6 and S8†). Titration of 5 and 8 with F− was monitored by fluorescence spectroscopy. As shown in Fig. 3b, compound 5 exhibits an emission maximum at 355 nm, while the emission maximum gradually decreased and the new emission peak at around 448 nm increased in intensity after the addition of F− and a well-defined isoemission point at 410 nm was observed, which revealed the formation of new species. Compound 8 showed similar results (Fig. 3d), but the emission color changed from blue to green after the titration (λem = 391 nm to 498 nm) with an isoemission point at λ = 452 nm. The polymers also exhibited almost identical titration features; P1 and P2 were titrated with TBAF in THF and monitored by UV-vis absorption (Fig. 3e and g) and fluorescence spectroscopy (Fig. 3f and h). Upon binding of F−, the bands in the UV-vis spectra of P1 (λmax = 379 nm) and P2 (λmax = 399 nm) were gradually quenched and new absorption peaks emerged at λ = 436 nm and λ = 446 nm, respectively. For the fluorescence spectra, a similar phenomenon was observed; the emission of P1 (λem = 414 nm) and P2 (λem = 458 nm) was gradually quenched and a more red-shifted emission appeared at λem = 516 and 541 nm, respectively. We calculated the F− binding constant of 5, 8, P1 and P2 using the Benesi–Hilderbrand plots shown in Fig. S14 (ESI†). The binding constants were determined to be 1.38 × 104 M−1 (5), 1.41 × 104 M−1 (8), 1.76 × 105 M−1 (P1) and 1.60 × 105 M−1 (P2). The emission intensity ratio of 5 (I448/I354), 8 (I498/I391), P1 (I516/I414) and P2 (I541/I458) increased with increasing the concentration of F− (Fig. S13†), which means that the BNP-containing small molecules and polymers can be used as colorimetric F− sensors.
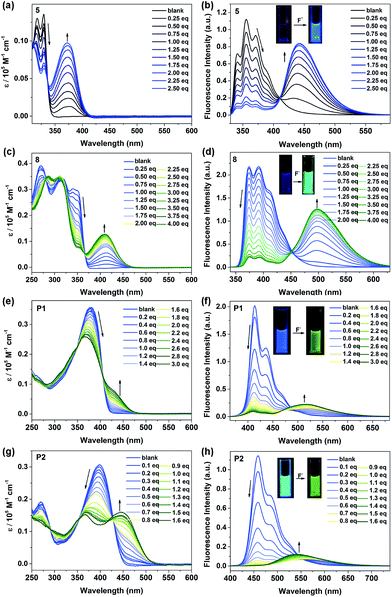 |
| Fig. 3 Spectral changes in the UV/vis absorption (left) and fluorescence (right) after the addition of TBAF. (a and b) 5, λex = 300 nm, [5] = 10 μM; (c and d) 8, λex = 300 nm, [8] = 10 μM; (e and f) P1, λex = 350 nm, [P1] = 10 μM; and (g and h) P2, λex = 365 nm, [P2] = 10 μM. [F−] = 1 mM. | |
In contrast to 5, the Stern–Volmer plots of polymer P2 showed more efficient fluorescence quenching (Fig. 4). The enhanced quenching efficiency of the polymer is likely due to the exciton migration along the polymer chain to lower energy quenching sites that are generated upon F− binding.56 This phenomenon is also known as the “molecular wire effect” or “super-quenching effect” for conjugated polymer-based sensors.57,58 In this case, however, the Stern–Volmer plots of P2 displayed an upward curvature in the intensity change, which could be a result of the combination of dynamic quenching and static quenching. In this system, the main reason for the upward curvature is the formation of weakly or non-emissive lower-energy aggregates upon fluoride titration, which might contribute to the observed enhancement in fluorescence quenching.56 Moreover, the fluorescence lifetimes and intensities of 5, 8, P1 and P2 were determined in the presence of different concentrations of F−, and the results are shown in Fig. 4a and b, S15 and S16.† We observed that the lifetime curves were close to a straight line after adding F−, indicating the static quenching nature of the sensing process.
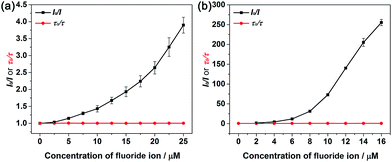 |
| Fig. 4 Stern–Volmer plots of fluorescence intensity (I0/I) and lifetime change (τ0/τ) as a function of [F−] of 5 (λem = 355 nm) (a) and P2 (λem = 458 nm) (b) in THF upon addition of n-Bu4NF. | |
The anion sensing properties of the BNP-containing small molecules and polymers were investigated upon simultaneous addition of a certain number of different anions. Fig. 5 shows the changes in the fluorescence spectra of 5, 8, P1 and P2 (1 × 10−5 M in THF) with 11 different anions, including F−, Cl−, Br−, I−, NO3−, ClO4−, BF4−, PF6−, AcO−, H2PO4− and SO42−. Specifically, upon the addition of F− to the solution of 5 and 8, the fluorescence spectra showed distinct changes both in intensity and emission wavelength, while other anions showed almost no change in the fluorescence spectra. Similarly, it is evident that P1 and P2 bind F− selectively, whereas other anions do not show any affinity (except for AcO− and SO42− anions, which show a slight affinity for P2). The dramatic anion-specific response suggests that compounds 5, 8, P1 and P2 are highly selective colorimetric sensors for the detection of fluoride anions.
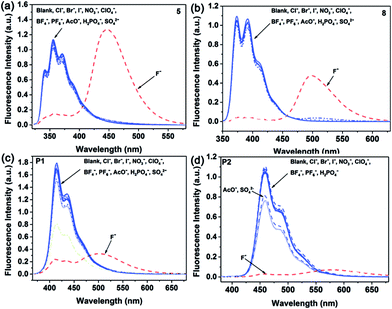 |
| Fig. 5 (a–d) Complexation of 5, 8, P1 and P2 (1 × 10−5 M in THF) with different anions (THF, 5 equiv. of Bu4N+ salts, 0.01 M). | |
The electron-deficient boron atoms of BN–phenanthrene units are captured by the added F− leading to a change of geometry at boron centers. In order to gain further insight into the mechanism, the reversibility of the sensing performance of 5 was investigated by UV-vis and fluorescence spectroscopy. Evidently, the new product 5-F can be converted back to the starting material, 5, by treatment with a stronger fluoride scavenger such as BF3·OEt2 (Fig. 6). These results supported our assumption regarding the origin of BN–phenanthrene as a stable conformation without decomposition. To further explore this assumption, DFT calculations (Gaussian 09, B3LYP/6-31G*) were conducted. Geometry optimization of the possible isomers of 5 and 5-F is shown in Fig. 6b. The calculated UV/Vis spectrum for 5-F (Fig. S6†) indicates that the dominant absorption occurring at ca. 365 nm is in good agreement with the experimental results (i.e., a major absorption band at 372 nm). The titrations were further verified by 19F and 11B NMR measurements. Upon addition of different equivalents of TBAF to compound 5, a new signal appeared at −156.6 ppm in 19F NMR (Fig. S37†) and 2.2 ppm in 11B NMR spectra (Fig. S38†), indicating the formation of a fluoroborate complex (5-F). These results also demonstrated the potential of the present boron system as a new type of fluorescent sensor for the detection of the fluoride ion.59
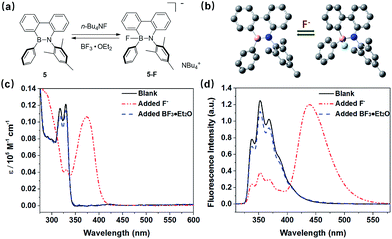 |
| Fig. 6 (a) Proposed interaction of 5 with fluoride anions; (b) illustration of the predicted shape changes for 5 upon fluoride ion binding; and the absorption (c) and emission (d) spectra of 5 in THF (solid line) upon addition of fluoride ions (dashed-dotted line) and BF3·OEt2 (dashed line). | |
2.5. Application of the film sensors for fluoride ions
The BNP-containing small molecules and polymers can also be used to fabricate film sensors for F− (Fig. 7). Compounds 5 and P2 were mixed with polycaprolactone (PCL) to prepare a solution mixture (weight ratio: 1/400), which was further cast on a pre-cleaned glass substrate to fabricate the sensor films. The compound 5-based film showed weak emission when irradiated with a hand-held UV lamp (λex = 365 nm) at room temperature. However, a bright blue luminescence was observed upon writing the characters “XJTU” on the film using TBAF solution. Similarly, a blue-green luminescence was found for P2 (λex = 365 nm), and it immediately transformed into a yellow-green “XJTU” luminescence upon addition of fluoride ions. These findings are promising for advanced optoelectronic applications in solid-state sensing of F− anions.
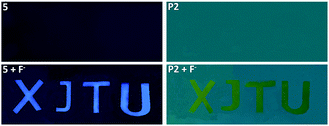 |
| Fig. 7 The 5 and P2-based film sensors for fluoride anions under UV irradiation (λex = 365 nm). | |
3 Conclusions
In summary, we have reported a highly efficient synthetic route for conjugated small molecules and polymers featuring 9,10-azaboraphenanthrene (BNP) units through aromaticity-driven ring-opening reactions. The BNP-containing conjugated small molecules and polymers showed good air and moisture stability as well as bright fluorescence properties; these compounds were used as effective and selective ratiometric sensors for F− in THF solution among 11 anions (F−, Cl−, Br−, I−, NO3−, ClO4−, BF4−, PF6−, AcO−, H2PO4−, and SO42−), resulting in dissimilar spectral changes from blue to green/yellow with different intensities, respectively. The large red shift in the absorption and the ratiometric fluorescence response are extremely desirable for the detection of F−. Further experiments and DFT calculation results showed that the sensing process is fully reversible. Future work will involve extending this synthetic concept to incorporate hydrophilic moieties into BNP units to obtain a new sensing platform for the detection of fluoride anions in aqueous medium.
Conflicts of interest
There are no conflicts to declare.
Acknowledgements
We gratefully acknowledge financial support from the Natural Science Foundation of China (21603016 and 21704081), the Natural Science Foundation of Shaanxi Province (2017JQ2023), the Cyrus Chung Ying Tang Foundation and the National 1000-Plan program. We also thank Prof. Yu Fang for helpful discussions. We are especially grateful to Prof. Yanzhen Zheng for his help with X-ray crystallographic analysis. We thank Dr. Gang Chang and Yu Wang of the Instrument Analysis Center of Xi'an Jiaotong University for their assistance with acquiring photoluminescence (PL) spectra.
Notes and references
- B. D. Gessner, M. Beller, J. P. Middaugh and G. M. Whitford, N. Engl. J. Med., 1994, 330, 95–99 CrossRef CAS PubMed
.
- P. Singh, M. Barjatiya, S. Dhing, R. Bhatnagar, S. Kothari and V. Dhar, Urol. Res., 2001, 29, 238–244 CrossRef CAS PubMed
.
- X. Zheng, W. Zhu, D. Liu, H. Ai, Y. Huang and Z. Lu, ACS Appl. Mater. Interfaces, 2014, 6, 7996–8000 CAS
.
- P. A. Gale, Acc. Chem. Res., 2006, 39, 465–475 CrossRef CAS PubMed
.
- P. A. Gale and C. Caltagirone, Chem. Soc. Rev., 2015, 44, 4212–4227 RSC
.
- Y. Zhou, J. F. Zhang and J. Yoon, Chem. Rev., 2014, 114, 5511–5571 CrossRef CAS PubMed
.
- S. Ayoob and A. K. Gupta, Crit. Rev. Environ. Sci. Technol., 2006, 36, 433–487 CrossRef CAS
.
- C. R. Wade, A. E. J. Broomsgrove, S. Aldridge and F. P. Gabbaï, Chem. Rev., 2010, 110, 3958–3984 CrossRef CAS PubMed
.
- M. Cametti and K. Rissanen, Chem. Soc. Rev., 2013, 42, 2016–2038 RSC
.
- M. Cametti and K. Rissanen, Chem. Commun., 2009, 2809–2829 RSC
.
- X.-F. Yang, H. Qi, L. Wang, Z. Su and G. Wang, Talanta, 2009, 80, 92–97 CrossRef CAS PubMed
.
- S. Y. Kim and J.-I. Hong, Org. Lett., 2007, 9, 3109–3112 CrossRef CAS PubMed
.
- F. Cheng, E. M. Bonder and F. Jäkle, J. Am. Chem. Soc., 2013, 135, 17286–17289 CrossRef CAS PubMed
.
- Z. Zhou, A. Wakamiya, T. Kushida and S. Yamaguchi, J. Am. Chem. Soc., 2012, 134, 4529–4532 CrossRef CAS PubMed
.
- L. Trembleau, T. A. D. Smith and M. H. Abdelrahman, Chem. Commun., 2013, 49, 5850–5852 RSC
.
- G. Turkoglu, M. E. Cinar and T. Ozturk, Eur. J. Org. Chem., 2017, 4552–4561 CrossRef CAS
.
- Z. Li, H. Li, H. Xia, X. Ding, X. Luo, X. Liu and Y. Mu, Chem.–Eur. J., 2015, 21, 17355–17362 CrossRef CAS PubMed
.
- F. Jäkle, Chem. Rev., 2010, 110, 3985–4022 CrossRef PubMed
.
- C. Fan, L. G. Mercier, W. E. Piers, H. M. Tuononen and M. Parvez, J. Am. Chem. Soc., 2010, 132, 9604–9606 CrossRef CAS PubMed
.
- H. Braunschweig, R. D. Dewhurst and A. Schneider, Chem. Rev., 2010, 110, 3924–3957 CrossRef CAS PubMed
.
- T. Kaese, A. Hübner, M. Bolte, H.-W. Lerner and M. Wagner, J. Am. Chem. Soc., 2016, 138, 6224–6233 CrossRef CAS PubMed
.
- A. Iida and S. Yamaguchi, J. Am. Chem. Soc., 2011, 133, 6952–6955 CrossRef CAS PubMed
.
- X.-Y. Wang, H.-R. Lin, T. Lei, D.-C. Yang, F.-D. Zhuang, J.-Y. Wang, S.-C. Yuan and J. Pei, Angew. Chem., Int. Ed., 2013, 52, 3117–3120 CrossRef CAS PubMed
.
- R. Zhao, C. Dou, Z. Xie, J. Liu and L. Wang, Angew. Chem., Int. Ed., 2016, 55, 5313–5317 CrossRef CAS PubMed
.
- X. Y. Wang, J. Y. Wang and J. Pei, Chem.–Eur. J., 2015, 21, 3528–3539 CrossRef CAS PubMed
.
- G. Li, Y. Zhao, J. Li, J. Cao, J. Zhu, X. W. Sun and Q. Zhang, J. Org. Chem., 2015, 80, 196–203 CrossRef CAS PubMed
.
- M. J. S. Dewar and W. H. Poesche, J. Am. Chem. Soc., 1963, 85, 2253–2256 CrossRef CAS
.
- M. J. S. Dewar and P. M. Maitlis, J. Am. Chem. Soc., 1961, 83, 187–193 CrossRef CAS
.
- A. W. Baggett, M. Vasiliu, B. Li, D. A. Dixon and S.-Y. Liu, J. Am. Chem. Soc., 2015, 137, 5536–5541 CrossRef CAS PubMed
.
- P. G. Campbell, A. J. V. Marwitz and S.-Y. Liu, Angew. Chem., Int. Ed., 2012, 51, 6074–6092 CrossRef CAS PubMed
.
- H. Kuhtz, F. Cheng, S. Schwedler, L. Boehling, A. Brockhinke, L. Weber, K. Parab and F. Jäkle, ACS Macro Lett., 2012, 1, 555–559 CrossRef CAS
.
- K. Liu, R. A. Lalancette and F. Jäkle, J. Am. Chem. Soc., 2017, 139, 18170–18173 CrossRef CAS PubMed
.
- H. Braunschweig, Q. Ye, M. A. Celik, R. D. Dewhurst and K. Radacki, Angew. Chem., Int. Ed., 2015, 54, 5065–5068 CrossRef CAS PubMed
.
- M. Arrowsmith, H. Braunschweig, K. Radacki, T. Thiess and A. Turkin, Chem.–Eur. J., 2017, 23, 2179–2184 CrossRef CAS PubMed
.
- M. Lepeltier, O. Lukoyanova, A. Jacobson, S. Jeeva and D. F. Perepichka, Chem. Commun., 2010, 46, 7007–7009 RSC
.
- J.-S. Lu, S.-B. Ko, N. R. Walters, Y. Kang, F. Sauriol and S. Wang, Angew. Chem., Int. Ed., 2013, 52, 4544–4548 CrossRef CAS PubMed
.
- T. Hatakeyama, S. Hashimoto, S. Seki and M. Nakamura, J. Am. Chem. Soc., 2011, 133, 18614–18617 CrossRef CAS PubMed
.
- W. Zhang, B. Zhang, D. Yu and G. He, Sci. Bull., 2017, 62, 899–900 CrossRef
.
- K. Huang and C. D. Martin, Inorg. Chem., 2015, 54, 1869–1875 CrossRef CAS PubMed
.
- K. Huang, S. A. Couchman, D. J. D. Wilson, J. L. Dutton and C. D. Martin, Inorg. Chem., 2015, 54, 8957–8968 CrossRef CAS PubMed
.
- A. Fukazawa, J. L. Dutton, C. Fan, L. G. Mercier, A. Y. Houghton, Q. Wu, W. E. Piers and M. Parvez, Chem. Sci., 2012, 3, 1814–1818 RSC
.
- C. Fan, W. E. Piers, M. Parvez and R. McDonald, Organometallics, 2010, 29, 5132–5139 CrossRef CAS
.
- S. A. Couchman, T. K. Thompson, D. J. D. Wilson, J. L. Dutton and C. D. Martin, Chem. Commun., 2014, 50, 11724–11726 RSC
.
- H. Braunschweig, C. Hoerl, L. Mailaender, K. Radacki and J. Wahler, Chem.–Eur. J., 2014, 20, 9858–9861 CrossRef CAS PubMed
.
- H. Braunschweig, M. A. Celik, F. Hupp, I. Krummenacher and L. Mailaender, Angew. Chem., Int. Ed., 2015, 54, 6347–6351 CrossRef CAS PubMed
.
- S. Yruegas, J. J. Martinez and C. D. Martin, Chem. Commun., 2018 10.1039/c8cc01529e
.
- H. Tian, J. Shi, S. Dong, D. Yan, L. Wang, Y. Geng and F. Wang, Chem. Commun., 2006, 3498–34500 RSC
.
- H. Tian, J. Wang, J. Shi, D. Yan, L. Wang, Y. Geng and F. Wang, J. Mater. Chem., 2005, 15, 3026 RSC
.
- C. Yang, H. Scheiber, E. J. W. List, J. Jacob and K. Müllen, Macromolecules, 2006, 39, 5213–5221 CrossRef CAS
.
- B. N. Boden, K. J. Jardine, A. C. W. Leung and M. J. MacLachlan, Org. Lett., 2006, 8, 1855–1858 CrossRef CAS PubMed
.
- M. J. D. Bosdet, C. A. Jaska, W. E. Piers, T. S. Sorensen and M. Parvez, Org. Lett., 2007, 9, 1395–1398 CrossRef CAS PubMed
.
- Z. Liu and T. B. Marder, Angew. Chem., Int. Ed., 2008, 47, 242–244 CrossRef CAS PubMed
.
- A. J. V. Marwitz, A. N. Lamm, L. N. Zakharov, M. Vasiliu, D. A. Dixon and S.-Y. Liu, Chem. Sci., 2012, 3, 825–829 RSC
.
- A. J. V. Marwitz, M. H. Matus, L. N. Zakharov, D. A. Dixon and S.-Y. Liu, Angew. Chem., Int. Ed., 2009, 48, 973–977 CrossRef CAS PubMed
.
- A. Iida, A. Sekioka and S. Yamaguchi, Chem. Sci., 2012, 3, 1461–1466 RSC
.
- K. Parab, K. Venkatasubbaiah and F. Jäkle, J. Am. Chem. Soc., 2006, 128, 12879–12885 CrossRef CAS PubMed
.
- G. He, N. Yan, H. Cui, T. Liu, L. Ding and Y. Fang, Macromolecules, 2011, 44, 7096–7099 CrossRef CAS
.
- D. T. McQuade, A. E. Pullen and T. M. Swager, Chem. Rev., 2000, 100, 2537–2574 CrossRef CAS PubMed
.
- S. Yamaguchi, T. Shirasaka, S. Akiyama and K. Tamao, J. Am. Chem. Soc., 2002, 124, 8816–8817 CrossRef CAS PubMed
.
Footnote |
† Electronic supplementary information (ESI) available: Experimental details, NMR spectra of all the new compounds, crystallographic data and results of theoretical calculations. CCDC 1818909. For ESI and crystallographic data in CIF or other electronic format see DOI: 10.1039/c8sc00688a |
|
This journal is © The Royal Society of Chemistry 2018 |
Click here to see how this site uses Cookies. View our privacy policy here.