DOI:
10.1039/C8SC01804A
(Edge Article)
Chem. Sci., 2018,
9, 6969-6974
Bifunctional iminophosphorane catalysed enantioselective sulfa-Michael addition of alkyl thiols to alkenyl benzimidazoles†
Received
20th April 2018
, Accepted 12th July 2018
First published on 23rd July 2018
Abstract
The first enantioselective sulfa-Michael addition of alkyl thiols to alkenyl benzimidazoles, enabled by a bifunctional iminophosphorane (BIMP) organocatalyst, is described. The iminophosphorane moiety of the catalyst provides the required basicity to deprotonate the thiol nucleophile while the chiral scaffold and H-bond donor control facial selectivity. The reaction is broad in scope with respect to the thiol and benzimidazole reaction partners with the reaction proceeding in up to 98% yield and 96
:
4 er.
N-Containing heterocycles are ubiquitous motifs in both biologically active molecules and natural products. Their functionalization, especially when performed in an enantioselective manner, is therefore of particular interest in the field of organic synthesis. Alkenyl azaarenes have been used extensively as synthetic precursors for the functionalization of N-containing heterocycles.1 The electron deficiency of the aromatic ring, part-activates the conjugated alkene towards Michael-type additions,2 allowing for the rapid generation of molecular complexity. Most recently, the groups of Harutyunyan, Terada and Meng reported elegant, highly enantioselective Michael additions to alkenyl N-heterocycles employing organocuprates,3 pyrazoles4 and B2(pin)2
5 respectively.
Our research has focused on developing enantioselective methods utilizing novel bifunctional iminophosphorane (BIMP) organocatalysts,6 which combine a chiral H-bond donor scaffold7 with an organo-superbase.8 More specifically, BIMP catalysis has been employed in the enantioselective addition of thiols9,10 to unactivated esters.6c,g This encouraged us to consider replacing the enoate electrophile with isoelectronic alkenyl benzimidazoles in order to access complex, chiral drug-like scaffolds with perfect atom economy and potential applications to medicinal chemistry (Fig. 1).11 To the best of our knowledge, there have been no reports to date of the enantioselective base catalysed Michael additions to alkenyl benzimidazoles12 and herein we wish to report our work leading to the first example, under BIMP catalysis.
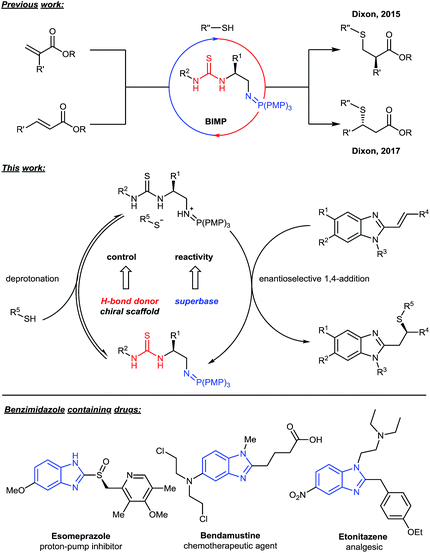 |
| Fig. 1 (Top) Previous BIMP catalysed sulfa-Michael additions to unactivated esters and current application to conjugated alkenyl benzimidazoles with a plausible catalytic cycle. (Bottom) Selected relevant benzimidazole containing drugs. PMP = para-methoxyphenyl. | |
We chose the readily prepared4 (E)-2-propenyl-1-tosyl-benzimidazole 1 and commercially available 1-propanethiol as model coupling partners to investigate reactivity and selectivity with a selection of bifunctional Brønsted base/H-bond donor catalysts using 3 eq. of thiol at 0.5 M concentration in THF at 22 °C for 24 hours (Fig. 2, Table 1). Quinidine derived catalyst A (entry 1) only provided 2 in 12% yield and a negligible 53
:
47 er. We therefore chose to investigate the more basic and more active BIMP catalysts in this reaction and were very pleased to find that known BIMP catalyst B6a bearing one stereocenter provided desired product 2 in 80% yield and 83
:
17 er (entry 2). With significant catalyst-enabled reactivity and stereocontrol identified we then proceeded to investigate second generation catalyst C6g which provided 2 in improved yield and er at 92% and 86
:
14 respectively (entry 3). Shifting the thiourea moiety further away from the iminophosphorane (D–E)6d showed no improvement in er over B (entries 4 and 5). We therefore focused on exploring catalysts built around the same chiral scaffold as C. Catalyst F6g bearing tBu groups at both stereocenters in the (S,S) configuration afforded 2 in 90% yield and 90
:
10 er (entry 6). Interestingly, a control reaction without any catalyst was found to go to completion (entry 7), indicating that an uncatalysed background reaction13 pathway was leading to an erosion in the enantiomeric ratio of the product. To suppress this background reactivity, the reaction was diluted to [0.06 M], cooled to 0 °C and only 1.2 eq. of thiol were used. The new set of conditions, combined with a solvent switch from THF to Et2O, provided 2 in 93% yield and 94
:
6 er using catalyst F (entry 8). Surprisingly a further decrease of the temperature to −40 °C led to an erosion of the enantiomeric ratio (entry 9). To further boost the enantiomeric ratio, diastereoisomeric catalyst G6g was screened. Pleasingly, catalyst G outperformed corresponding diastereomer F affording the desired product in 98% yield and 95
:
5 er (entry 10).
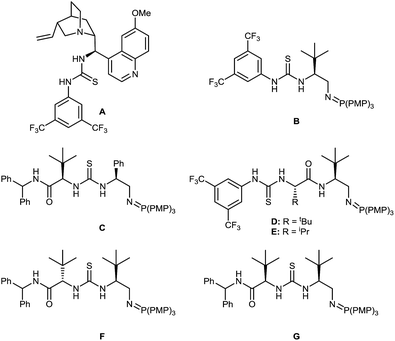 |
| Fig. 2 Selected BIMP catalysts investigated for the optimization of the sulfa-Michael addition. PMP = para-methoxyphenyl. | |
Table 1 Reaction optimization. Full optimization data available in the ESI
With optimal conditions established, we proceeded to explore the scope and limitations of this transformation (Scheme 1). Initially the steric and electronic properties of the thiol nucleophile were varied. Higher order linear, branched and cyclic alkyl substituents on the thiol all provided the corresponding Michael adducts (3–5) with high yields and enantioselectivities. The introduction of a phenyl ring was well tolerated providing 6 in outstanding yield and good er. Appending a silyl group to the thiol nucleophile showed no detrimental effect providing 7 in excellent yield and er. Benzyl thiols provided corresponding Michael adducts 8–10 in high yields in all cases and good enantioselectivity, albeit slightly diminished when compared to simpler alkyl thiols.15,16
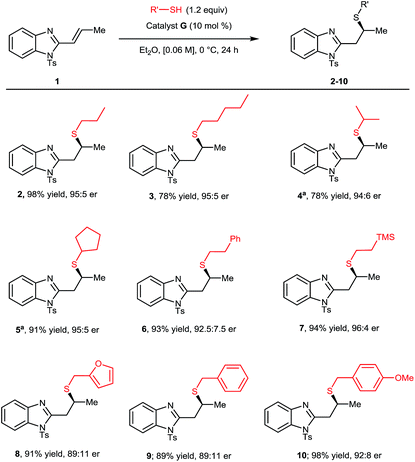 |
| Scheme 1 Scope of the thiol coupling partner. aReaction carried out at 22 °C. | |
Having investigated the thiol component, we then focused on substituent effects on the benzimidazole core (Scheme 2). Variations to the phenyl backbone did not affect reactivity, disubstitution at C5 and C6 with methyl groups afforded corresponding adduct 11 in 81% yield and 86
:
14 er. Alternating monosubstitution between C5 and C6 did not have a large effect, with bromine containing substrates affording the corresponding Michael adducts (12, 13) in greater than 75% yield and 86
:
14 er allowing for potential further functionalization at both positions.17
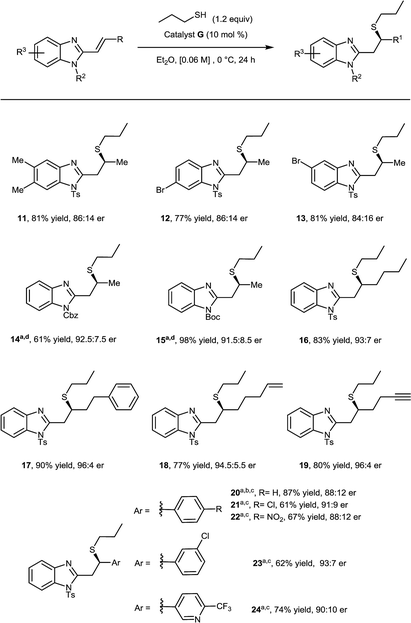 |
| Scheme 2 Scope of the alkenyl benzimidazole coupling partner. aReaction carried out at 22 °C. bReaction carried out using catalyst F. cReaction carried out in THF. dReaction carried out using 3 eq. of thiol. | |
We were pleased to find that the high enantioselectivity of the reaction was largely maintained when the nitrogen protecting group was changed from N-tosyl to N-Cbz (14) or N-Boc (15), however in these cases reactivity was found to diminish. This was easily circumvented by running the reaction at 22 °C using 3 equivalents of 1-propanethiol.18
Having varied the substitution pattern on the benzimidazole, we proceeded to investigate the scope with respect to substituents on the alkenyl moiety. The introduction of higher order linear alkyl chains, bearing aromatic, alkene and alkyne substituents, was well-tolerated with all n-propyl thiol Michael additions providing the corresponding products (16–19) in excellent yield and enantioselectivity. When substituting the alkene moiety with an aromatic group, the solvent was switched to THF and reactions were run at 22 °C due to decreased solubility and reactivity of the substrates. When a phenyl substituent was introduced on the alkenyl moiety, catalyst G only provided a moderate Michael adduct 20 in 77
:
23 er, however this was boosted to 88
:
12 when using diastereomeric catalyst F. Introducing electron withdrawing groups at either the para or meta positions of the phenyl ring afforded the corresponding products 21–23 in good yield and enantioselectivity; in these cases, however, catalyst G proved superior to F. Finally, when the phenyl ring was exchanged with a 3-pyridyl moiety, it smoothly afforded the corresponding adduct 24 in 74% yield and 90
:
10 er.
Increasing the reaction scale 10-fold (1 mmol) afforded 2 in equal yield and er, which upon treatment with HCl (5 M aq.) gave corresponding deprotected product 25 in quantitative yield. Single crystal X-ray analysis of 25 allowed the absolute configuration of sulfa-Michael product 2 to be determined as S when using catalyst G. We were also pleased to find that, upon treatment of 2 with m-CPBA, sulfone 26 was obtained in 95% yield with no loss of optical purity (Scheme 3).
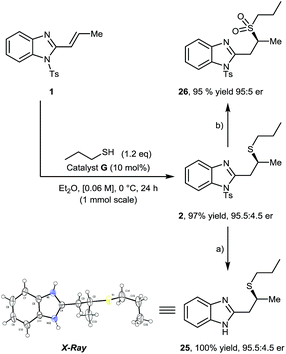 |
| Scheme 3 Scale up (to 1 mmol) and derivatization of compound 2 and determination of absolute configuration of 25 by single crystal X-ray analysis. (a) 5 M aq. HCl, 40 °C, THF, 10 h. (b) m-CPBA, CH2Cl2, 22 °C, 4 h. | |
We used density functional theory (DFT) to investigate the origins of enantioselectivity, performing calculations at the wB97XD/6-31G(d) level of theory (Fig. 3).19 Calculations considered PPh3-derived catalyst G* with the PMP-groups of G modelled by Ph-groups. The most stable conformation of (most enantioselective) catalyst G* has substituents either side of the urea oriented with a hydrogen atom towards sulfur: other rotamers are disfavoured. This creates a pocket with the iminophosphorane positioned above the thiourea (from the perspective of Fig. 3). Two substrate activation modes are possible (Avs.B) and either could in principle lead to the formation of the major observed enantiomer. Computationally, we find that the interaction of the thiolate nucleophile with the protonated iminophoshorane and the benzimidazole with the thiourea (mode A) is energetically favored by 4–5 kcal mol−1 over the alternative (mode B) in which the thiourea binds the nucleophile and the benzimidazole to the protonated iminophoshorane. This mode of activation is consistent with the observed sense of enantioselectivity, and with earlier mechanistic proposals of Takemoto. Recent theoretical studies of Grayson and Houk have emphasized the importance of activation mode B in sulfa-Michael reactions promoted by Cinchona-derived catalysts.20 Our present results suggest that both activation modes may be operative, depending on catalyst and substrate, as originally hypothesized by Soós and I. Pápai.21
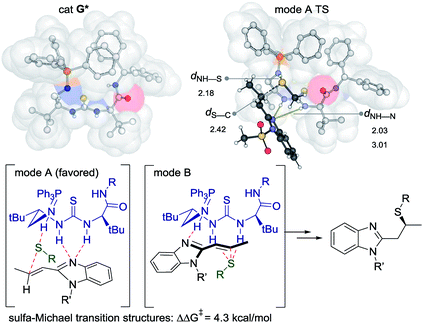 |
| Fig. 3 SMD-wB97XD/6-31G(d) computed structure of catalyst G* and the most favourable transition structure leading to the major enantiomer. | |
Conclusions
In summary, the first enantioselective sulfa-Michael addition of alkyl thiols to alkenyl benzimidazoles has been described. Excellent yields and good enantioselectivities were achieved across a broad range of alkyl thiol and alkenyl benzimidazole reaction partners using a second generation BIMP organocatalyst. This work further demonstrates the versatility and high activity of the BIMP catalyst family, as well as expanding its use in methodology for the synthesis of biologically relevant chiral benzimidazole derivatives. Further investigations into new catalyst designs and applications for BIMP promoted reactivity are underway in our laboratories.
Conflicts of interest
There are no conflicts to declare.
Acknowledgements
M. F. is grateful to the EPSRC Centre for Doctoral Training in Synthesis for Biology and Medicine (EP/L015838/1) for a studentship, generously supported by AstraZeneca, Diamond Light Source, Defence Science and Technology Laboratory, Evotec, GlaxoSmithKline, Janssen, Novartis, Pfizer, Syngenta, Takeda, UCB and Vertex. G. S. is grateful to the Université Paris Descartes for CRCT funding. J. D. thanks the Spanish Ministry of Education, Culture and Sports for a Jose Castillejo grant. We also thank Heyao Shi for X-ray structure determination and Dr Amber L. Thompson and Dr Kirsten E. Christensen (Oxford Chemical Crystallography Service) for X-ray mentoring and help. R. S. P. acknowledges the RMACC Summit supercomputer supported by the National Science Foundation (ACI-1532235 and ACI-1532236), the University of Colorado Boulder and Colorado State University and the Extreme Science and Engineering Discovery Environment (XSEDE) through allocation TG-CHE180006.
Notes and references
- For reviews on conjugate addition to N-containing heterocycles, see:
(a) D. A. Klumpp, Synlett, 2012, 23, 1590 CrossRef;
(b) D. Best and H. W. Lam, J. Org. Chem., 2014, 79, 831 CrossRef PubMed.
-
(a) L. Rupnicki, A. Saxena and H. W. Lam, J. Am. Chem. Soc., 2009, 131, 10386 CrossRef PubMed;
(b) G. Pattison, G. Piraux and H. W. Lam, J. Am. Chem. Soc., 2010, 132, 14373 CrossRef PubMed;
(c) A. Saxena and H. W. Lam, Chem. Sci., 2011, 2, 2326 RSC;
(d) A. A. Friedman, J. Panteleev, J. Tsoung, V. Huynh and M. Lautens, Angew. Chem., Int. Ed., 2013, 52, 9755 CrossRef PubMed;
(e) S. Wang, X. Li, H. Liu, L. Xu, J. Zhuang, J. Li, H. Li and W. Wang, J. Am. Chem. Soc., 2015, 137, 2303 CrossRef PubMed.
-
(a) R. P. Jumde, F. Lanza, M. J. Veenstra and S. R. Harutyunyan, Science, 2016, 352, 433 CrossRef PubMed;
(b) R. P. Jumde, F. Lanza, T. Pellegrini and S. R. Harutyunyan, Nat. Commun., 2017, 8, 2058 CrossRef PubMed.
- Y. Y. Wang, K. Kanomata, T. Korenaga and M. Terada, Angew. Chem., Int. Ed., 2016, 55, 927 CrossRef PubMed.
- L. Wen, Z. Yue, H. Zhang, Q. Chong and F. Meng, Org. Lett., 2017, 19, 6610 CrossRef PubMed.
- For reports on bifunctional iminophosphorane catalysis, see:
(a) M. G. Núñez, A. J. M. Farley and D. J. Dixon, J. Am. Chem. Soc., 2013, 135, 16348 CrossRef PubMed;
(b) A. M. Goldys, M. G. Núñez and D. J. Dixon, Org. Lett., 2014, 16, 6294 CrossRef PubMed;
(c) A. J. M. Farley, C. Sandford and D. J. Dixon, J. Am. Chem. Soc., 2015, 137, 15992 CrossRef PubMed;
(d) G. P. Robertson, A. J. M. Farley and D. J. Dixon, Synlett, 2016, 27, 21 Search PubMed;
(e) M. A. Horwitz, B. P. Zavesky, J. I. Martinez-Alvarado and J. S. Johnson, Org. Lett., 2016, 18, 36 CrossRef PubMed;
(f) M. A. Horwitz, J. L. Fulton and J. S. Johnson, Org. Lett., 2017, 19, 5783 CrossRef PubMed;
(g) J. Yang, A. J. M. Farley and D. J. Dixon, Chem. Sci., 2017, 8, 606 RSC;
(h) H. Shi, I. M. Michaelides, B. Darses, P. Jakubec, Q. N. N. Nguyen, R. S. Paton and D. J. Dixon, J. Am. Chem. Soc., 2017, 139, 17755 CrossRef PubMed;
(i) J. L. Fulton, M. A. Horwitz, E. L. Bruske and J. S. Johnson, J. Org. Chem., 2018, 83, 3385 CrossRef PubMed.
- For selected reviews on (thio)urea bifunctional organocatalysis, see:
(a) Y. Takemoto, Org. Biomol. Chem., 2005, 3, 4299 RSC;
(b) S. J. Connon, Chem. Commun., 2008, 0, 2499 RSC;
(c) X. Yu and W. Wang, Chem.–Asian J., 2008, 3, 516 CrossRef PubMed;
(d) F. E. Held and S. B. Tsogoeva, Catal. Sci. Technol., 2016, 6, 645 RSC.
- For reviews on the use of organic superbases in organic synthesis, see:
(a) C. Palomo, M. Oiarbide and R. López, Chem. Soc. Rev., 2009, 38, 632 RSC;
(b)
T. Ishikawa, Superbases for Organic Synthesis, John Wiley & Sons, Ltd, Chichester, UK, 2009 CrossRef;
(c) D. Leow and C. H. Tan, Chem.–Asian J., 2009, 4, 488 CrossRef PubMed;
(d) D. Leow and C. H. Tan, Synlett, 2010, 11, 1589 Search PubMed;
(e) T. Ishikawa, Chem. Pharm. Bull., 2010, 58, 1555 CrossRef PubMed;
(f) X. Fu and C. H. Tan, Chem. Commun., 2011, 47, 8210 RSC;
(g) T. Ishikawa and L. M. Harwood, Synlett, 2013, 24, 2507 CrossRef;
(h) H. Krawczyk, M. Dzięgielewski, D. Deredas, A. Albrecht and Ł. Albrecht, Chem.–Eur. J., 2015, 21, 10268 CrossRef PubMed;
(i) B. Teng, W. C. Lim and C. H. Tan, Synlett, 2017, 28, 1272 CrossRef, for selected recent publications, see:
(j) J. S. Bandar and T. H. Lambert, J. Am. Chem. Soc., 2013, 135, 11799 CrossRef PubMed;
(k) X. Gao, J. Han and L. Wang, Org. Lett., 2015, 17, 4596 CrossRef PubMed;
(l) M. Işk, M. Y. Unver and C. Tanyeli, J. Org. Chem., 2015, 80, 828 CrossRef PubMed;
(m) D. Uraguchi, K. Yamada and T. Ooi, Angew. Chem., Int. Ed., 2015, 54, 9954 CrossRef PubMed;
(n) J. S. Bandar, A. Barthelme, A. Y. Mazori and T. H. Lambert, Chem. Sci., 2015, 6, 1537 RSC;
(o) E. D. Nacsa and T. H. Lambert, J. Am. Chem. Soc., 2015, 137, 10246 CrossRef PubMed;
(p) M. A. Horwitz, N. Tanaka, T. Yokosaka, D. Uraguchi, J. S. Johnson and T. Ooi, Chem. Sci., 2015, 6, 6086 RSC;
(q) A. Kondoh, M. Oishi, T. Takeda and M. Terada, Angew. Chem., Int. Ed., 2015, 54, 15836 CrossRef PubMed;
(r) V. H. Lauridsen, L. Ibsen, J. Blom and K. A. Jørgensen, Chem.–Eur. J., 2016, 22, 3259 CrossRef PubMed;
(s) T. Takeda, A. Kondoh and M. Terada, Angew. Chem., Int. Ed., 2016, 55, 4734 CrossRef PubMed;
(t) D. Uraguchi, K. Yoshioka and T. Ooi, Nat. Commun., 2017, 8, 14793 CrossRef PubMed;
(u) K. Yoshioka, K. Yamada, D. Uraguchi and T. Ooi, Chem. Commun., 2017, 53, 5495 RSC;
(v) N. Tanaka, R. Tsutsumi, D. Uraguchi and T. Ooi, Chem. Commun., 2017, 53, 6999 RSC;
(w) D. Uraguchi, R. Shibazaki, N. Tanaka, K. Yamada, K. Yoshioka and T. Ooi, Angew. Chem., Int. Ed., 2018, 57, 4732 CrossRef PubMed;
(x) D. Uraguchi, Y. Kawai, H. Sasaki, K. Yamada and T. Ooi, Chem. Lett., 2018, 47, 594 CrossRef;
(y) A. Kondoh, S. Akahira, M. Oishi and M. Terada, Angew. Chem., Int. Ed., 2018, 57, 6299 CrossRef PubMed;
(z) D. Uraguchi, K. Yamada, M. Sato and T. Ooi, J. Am. Chem. Soc., 2018, 140, 5110 CrossRef PubMed.
- For review on asymmetric sulfa-Michael additions, see:
(a) D. Enders, K. Luttgen and A. A. Narine, Synthesis, 2007, 959 CrossRef. For selected examples using metals, see:
(b) K. Nishimura, M. Ono, Y. Nagaoka and K. J. Tomioka, J. Am. Chem. Soc., 1997, 119, 12974 CrossRef;
(c) S. Kanemasa, Y. Oderaotoshi and E. Wada, J. Am. Chem. Soc., 1999, 121, 8675 CrossRef;
(d) K. Nishimura, M. Ono, Y. Nagaoka and K. Tomioka, Angew. Chem., Int. Ed., 2001, 40, 440 CrossRef;
(e) Y. Hui, J. Jiang, W. Wang, W. Chen, Y. Cai, L. Lin, X. Liu and X. Feng, Angew. Chem., Int. Ed., 2010, 49, 4290 CrossRef PubMed;
(f) S. Bonollo, D. Lanari, F. Pizzo and L. Vaccaro, Org. Lett., 2011, 13, 2150 CrossRef PubMed;
(g) T. Kitanosono, M. Sakai, M. Ueno and S. Kobayashi, Org. Biomol. Chem., 2012, 10, 7134 RSC;
(h) T. Ogawa, N. Kumagai and M. Shibasaki, Angew. Chem., Int. Ed., 2012, 51, 8551 CrossRef PubMed.
- For a review on organocatalytic asymmetric sulfa Michael addition reactions, see:
(a) P. Chauhan, S. Mahajan and D. Enders, Chem. Rev., 2014, 114, 8807 CrossRef PubMed, for seminal contributions, see:
(b) H. Hiemstra and H. Wynberg, J. Am. Chem. Soc., 1981, 103, 417 CrossRef;
(c) A. Kumar, R. V. Salunkhe, R. A. Rane and S. Y. Dike, J. Chem. Soc., Chem. Commun., 1991, 485 RSC, for selected recent examples, see:
(d) C. Palacio and S. Connon, Chem. Commun., 2012, 48, 2849 RSC;
(e) D. Uraguchi, N. Kinoshita, D. Nakashima and T. Ooi, Chem. Sci., 2012, 3, 3161 RSC;
(f) L. Dai, H. Yang, J. Niu and F. Chen, Synlett, 2012, 314 Search PubMed;
(g) A. C. Breman, J. M. M. Smits, R. de Gelder, J. H. van Maarseveen, S. Ingemann and H. Hiemstra, Synlett, 2012, 23, 2195 CrossRef;
(h) X. Fang, J. Li and C.-J. Wang, Org. Lett., 2013, 15, 3448 CrossRef PubMed;
(i) R. A. Unhale, N. K. Rana and V. K. Singh, Tetrahedron Lett., 2013, 54, 1911 CrossRef;
(j) R. Wang, J. Liu and J. Xu, Adv. Synth. Catal., 2014, 357, 159 CrossRef;
(k) J. P. Phelan, E. J. Patel and J. A. Ellman, Angew. Chem., Int. Ed., 2014, 53, 11329 CrossRef PubMed;
(l) N. K. Fu, L. Zhang, S. Z. Luo and J. P. Cheng, Org. Lett., 2014, 16, 4626 CrossRef PubMed;
(m) P. Yuan, S. Meng, J. Chen and Y. Huang, Synlett, 2016, 27, 1068 CrossRef;
(n) G. Wang, Y. Tang, Y. Zhang, X. Liu, L. Lin and X. Feng, Chem.–Eur. J., 2017, 23, 554 CrossRef PubMed;
(o) Q. Wei, W. Hou, N. Liao and Y. Peng, Adv. Synth. Catal., 2017, 359, 2364 CrossRef;
(p) A. Bacsó, M. Szigeti, S. Varga and T. Soós, Synthesis, 2017, 49, 429 Search PubMed;
(q) J. Guo and M. W. Wong, J. Org. Chem., 2017, 82, 4362 CrossRef PubMed;
(r) A. K. Simlandy and S. Mukherjee, J. Org. Chem., 2017, 82, 4851 CrossRef PubMed;
(s) Y. Fukata, K. Yao, R. Miyaji, K. Asano and S. Matsubara, J. Org. Chem., 2017, 82, 12655 CrossRef PubMed;
(t) D. Hasılcıoğulları and C. Tanyeli, Tetrahedron Lett., 2018, 59, 1414 CrossRef.
- For selected reviews on benzimidazoles in medicinal chemistry, see:
(a) Y. Bansal and O. Silakari, Bioorg. Med. Chem., 2012, 20, 6208 CrossRef PubMed;
(b) M. Gaba, S. Singh and C. Mohan, Eur. J. Med. Chem., 2014, 76, 494 CrossRef PubMed;
(c) R. S. Keri, A. Hiremathad, S. Budagumpi and B. M. Nagaraja, Chem. Biol. Drug Des., 2015, 86, 19 CrossRef PubMed;
(d) O. O. Ajani, D. V. Aderohunmu, C. O. Ikpo, A. E. Adedapo and I. O. Olanrewaju, Arch. Pharm. Chem. Life Sci., 2016, 349, 475 CrossRef PubMed;
(e) P. M. Krishna Prasad, S. A. Kanvinde and S. Raja, Int. J. Pharm. Pharm. Sci., 2016, 8, 22 CrossRef.
- Only one report describes the addition of thiols to alkenyl benzimidazoles promoted by AcOH at 100 °C to give racemic products: P. Dhanalakshmi and S. Shanmugam, Tetrahedron, 2015, 71, 6300 CrossRef.
- The background reaction was found to be catalysed by trace amounts of acid present in the commercial 1-propane thiol; this however was not found to affect the BIMP catalysed reaction under the optimised conditions. Full control experiments are available in the ESI.†.
- Catalyst A gave no conversion when the reaction was carried out at 0 °C, using 1.2 eq. of thiol and 0.06 M concentration for 24 h.
- Michael adduct 10 was deprotected to obtain free thiol 27 upon treatment with anisole in TFA at 65 °C without any loss of optical purity (see ESI†)
.
- When 4-methylbenzene thiol was used as the nucleophile, the corresponding Michael adduct was obtained in 85% yield but only 69
:
31 er.
- When the benzimidazole ring was substituted with a methyl group at the 4-position (28) the sulfa-Michael reaction failed to proceed under the optimised reaction conditions. When the reaction was run at 0.125 M concentration in THF, 22 °C and with 3 equivalents of n-propane thiol, the corresponding sulfa-Michael product 29 was obtained in 60% yield and 50
:
50 er. See ESI† for full details
.
- The sulfa-Michael reaction failed to proceed under the optimised reaction conditions when using the unprotected variant of benzimidazole 2. When the reaction was run at 0.5 M concentration, 22 °C and with 3 equivalents of n-propane thiol, 25 was obtained in 61% yield and 53
:
47 er.
- Calculations were performed with Gaussian 09 rev. D.01: Frisch, M. J. et al. Gaussian, Inc.: Wallingford, CT, 2013. All calculations were performed with an SMD model of diethyl ether. Full details are given in the ESI.†.
-
(a) M. N. Grayson and K. N. Houk, J. Am. Chem. Soc., 2016, 138, 1170 CrossRef PubMed;
(b) M. N. Grayson and K. N. Houk, J. Am. Chem. Soc., 2016, 138, 9041 CrossRef PubMed;
(c) M. N. Grayson, J. Org. Chem., 2017, 82, 4396 CrossRef PubMed.
- A. Hamza, G. Schubert, T. Soós and I. Pápai, J. Am. Chem. Soc., 2006, 128, 13151 CrossRef PubMed.
Footnotes |
† Electronic supplementary information (ESI) available. CCDC 1833189. For ESI and crystallographic data in CIF or other electronic format see DOI: 10.1039/c8sc01804a |
‡ These authors contributed equally. |
|
This journal is © The Royal Society of Chemistry 2018 |
Click here to see how this site uses Cookies. View our privacy policy here.