DOI:
10.1039/C8SC02276C
(Edge Article)
Chem. Sci., 2018,
9, 8228-8233
New self-assembling peptide nanotubes of large diameter using δ-amino acids†
Received
23rd May 2018
, Accepted 26th August 2018
First published on 27th August 2018
Abstract
Here we show that 4-aminocyclohexanecarboxylic acid is a rigid stretcher building block for the preparation of cyclic peptides that self-assemble to form peptide nanotubes with large diameter and hydrophobic pores. The hydrophobic properties of the resulting nanotubes provided by the two methylene groups per δ-residue allow the encapsulation of C60 moieties forming a new type of bionanopeapod structure.
1 Introduction
Self-assembling cyclic peptide nanotubes (SCPNs) are hollow cylindrical-shaped supramolecular structures formed by the stacking of cyclic peptides (CPs) in a flat conformation.1 Their simple synthesis, precise control of the internal pore dimensions, the biocompatibility and easy modification make them especially suitable for the development of new nanobiomaterials.2 Recent studies have shown that these supramolecular polymers are one of the known most robust nanofibers derived from proteinaceous materials.3 In fact, the same authors have made composites with polymers as a structural filler to reinforce their mechanical strength.4 All these properties have given rise to search for applications in fields ranging from biology (antimicrobials, drug delivery…) to materials science (biomaterials, molecular electronics, sensing and so on).5,6 Among the pioneering SCPNs in which CPs made of α-amino acids of alternating opposite chirality (D,L-α-CP)7 were used, other amino acids (β-, γ-, δ- or ε-residues) have been also reported for the preparation of nanotubes.1,8 Especially attractive are the CPs that contain γ-amino acids (α,γ-CPs) because they form nanotubes with partially hydrophobic or functionalized cavities.9,10
In this sense, nanotubes with hydrophobic cavities are especially demanded for their potential implementation in the design of new drug or diagnostic agent carrier systems.11 We envisioned that 4-aminocyclohexanecarboxylic acid (δ-Ach) would be a promising residue in the preparation of nanotubes with hydrophobic properties because of the two methylene groups of each residue that would be projected towards the inner cavity of the nanotube. In this article, new hybrid α,δ-cyclic peptides (D,L-α,δ-CPs) with self-assembling properties are described. These peptides allow the formation of nanotubes with large internal diameters and hydrophobic properties. The resulting ensemble is able to dissolve and encapsulate fullerene in aqueous media inside of the tubular pore.
2 Results and discussion
2.1 Synthetic studies and dimer characterization
For the design of these new nanotube forming cyclic peptides we used D,L-α-CPs as the basic component and incorporated one δ-Ach residue after each α-amino acid (Scheme 1) to ensure the adoption of a flat conformation. In addition, this design would also assure that the hydrogen-bond donors and acceptors were complementary in each face of the CP, facilitating the nanotube formation. To carry out these studies we started with dimers forming D,L-α-CPs of different lengths,12 tetramers (n = 1), hexamers (n = 2) and octamers (n = 3). In these models (CP1, CP2 and CP3) the amide protons in one face of the β-strand are substituted by methyl groups through the alkylation of all the amino acids of the same chirality to prevent hydrogen bond formation through this face. Therefore, the assembling process is restricted to dimers that are soluble in organic solvents. To ensure that all the N-alkyl groups are pointing in the same direction, in these new hybrids the N-methylated δ-Ach residues should be coupled to the N-methylated α-residue (Scheme 1). Consequently, the non-methylated δ-amino acids should be attached to the α-residues of opposite chirality (D-residue) through a secondary amide connection. With these design principles, cyclic peptides CP4, CP5 and CP6 were synthetized following the general scheme illustrated in Scheme 1S in the ESI.† The resulting dimers D4, D5 and D6 (Scheme 1) should be formed by the hydrogen-bonding interactions between the amide groups of D-amino acids and the NH group of δ-Ach with the carbonyl group of MeN-δ-Ach.
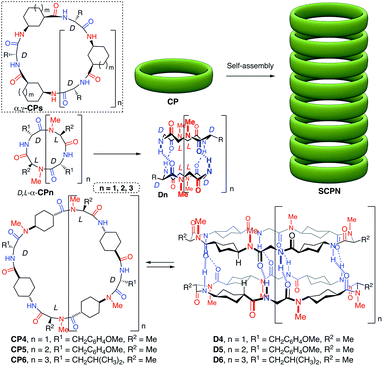 |
| Scheme 1 Top: Peptide nanotube models formed by stacking of cyclic peptides (SCPN) and structure of α,γ-cyclic peptide hybrids. Bottom: Structure of the cyclic peptides and dimers of D,L-α-CP (centre) and D,L-α,δ-CP (bottom) in which CP4 corresponds to n = 1 (octamer), CP5 to n = 2 (dodecamer) and CP6 to n = 3 (hexadecamer). | |
The synthesis (Scheme 2, see the ESI and Scheme 1S† for more details) started from commercially available trans-N-Boc-4-aminocyclohexanecarboxylic acid (Boc-δ-Ach-OH) that was transformed into Boc-MeN-δ-Ach-OH by treatment with sodium hydride and iodomethane in DMF followed by hydrolysis with lithium hydroxide of the resulting methyl ester. Finally, Boc-δ-Ach-OH was also transformed into Boc-δ-Ach-OFm under simple esterification conditions. This compound was converted into the corresponding tetrapeptides (Tp1a or Tp1b, Scheme 1S†) through the sequential coupling/deprotection steps of the corresponding Boc-protected amino acids (MeN-Ala, MeN-δ-Ach and D-Tyr for Tp1a, and MeN-Ala, MeN-δ-Ach and D-Leu for Tp1b). The resulting tetrapeptides, through successive deprotection and coupling processes, were transformed into the corresponding linear octa-, dodeca- and hexadecapeptides (Op1, Dd1 and Hd1a and/or b) that were finally cyclized to provide CP4, CP5 and CP6, respectively, in yields ranging from 34 to 64%.
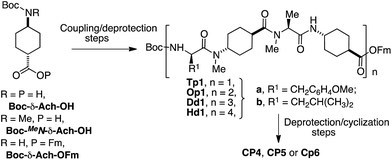 |
| Scheme 2 Synthetic strategy used in the synthesis of cyclic peptides CP4, CP5 and CP6. For more details, see Scheme 1S in the ESI.† | |
The CPs were soluble in nonpolar solvents, i.e. deuterochloroform, allowing the analysis of the self-assembling properties by NMR experiments. The 1H NMR spectra in deuterochloroform of the three compounds are well defined and highly symmetrical with JNH,γH coupling constants larger than 7.0 Hz. This indicates that the peptides must exist in an all-trans conformation characteristic of the flat-ring-shaped structure (Fig. 1). The main difference is the chemical shift of the amide protons. While in this solvent the signals of these protons of CP4 appear at δ 6.48 (NHTyr) and 6.23 (NH∂-Ach) ppm, independently of peptide concentration, the signals of amide protons are shifted downfield, δ 8.68 (NHTyr) and 7.78 (NH∂-Ach) ppm for CP5 and 8.32 (NHLeu) and 7.91 (NH∂-Ach) ppm for CP6. These results suggest that the small CPs remain as monomers in solution with the amide protons not involved in a hydrogen bonding interaction. This was also confirmed by solution and solid state FTIR (Fig. 1aS†) that show an amide A band at 3400 cm−1 that corresponds to the stretching of amide protons not involved in hydrogen bonding.13
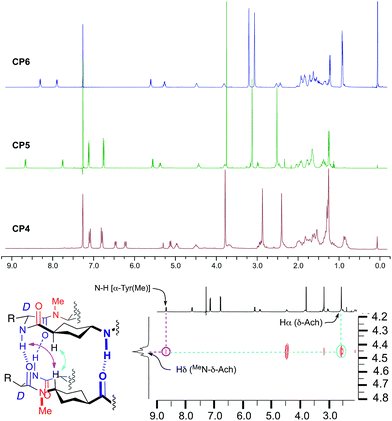 |
| Fig. 1 Top: 1H NMR spectra of CP4 (bottom, red), CP5 (media, green) and CP6 (top, blue). Bottom: Selected NOESY spectra of D5 (5 mM in CDCl3); on the left the coloured arrows denote the main characteristic cross peaks, magenta for NH (Tyr) and cyan for Hδ(δ-MeN-Ach), Hδ(δ-MeN-Ach) and Hα(δ-Ach). | |
On the other hand, the other two CPs must form in this solvent (CDCl3) the corresponding dimers, D5 and D6, with a very large association constant (Ka > 105 M−1), as deduced from the location of the amide signals (8.68 and 7.78 ppm for CP5 and 8.32 and 7.91 ppm for CP6) that remains constant at concentrations as low as 1 × 10−4 M (Fig. 2S†). NOE cross peaks (Fig. 1) between Tyr amide protons (8.68 ppm) and the Hδ of δ-MeN-Ach (4.45 ppm) and between the latter and Hα of δ-MeN-Ach (2.54 ppm) for CP5 are consistent with the proposed antiparallel dimeric structure. Similar cross peaks were also observed for CP6 (see ESI, Fig. 3S†). Dimer formation was also verified by electrospray ionisation-time-of-flight (ESI-TOF) mass spectroscopy that showed peaks corresponding to the dimeric forms with two (1580.9 for CP5 and 1851.3 for CP6) and three charges (1054.3 for CP5 and 1234.5 for CP6), as deduced from the isotopic distribution, see Fig. 4S in the ESI.† The FTIR spectra (Fig. 1bS and cS†) also confirm the formation of the dimer assemblies with an amide A band near 3315 cm−1 that suggests an inter-monomer distance of around 4.85–4.90 Å.13 These experiments confirm that the intercalation of the rigid δ-Ach residues into dimers forming self-assembling D,L-α-CPs provides a new class of CPs that adopt a flat conformation and stack to form torus-shaped supramolecular ensembles; see Fig. 5S in the ESI† for a computer generated model of CP5. The rigidity of this residue allows the formation of a large pore structure with diameters as big as 30 Å, the largest published for this type of tubular assembly.
2.2 Nanotube characterization
After confirming the assembling properties of the D,L-α,δ-CPs with dimeric models, we extend the studies to the formation of SCPNs. For that purpose, cyclic dodecapeptide CP7 (Scheme 3) was designed. The incorporation of Glu and Lys residues should provide CPs with improved solubility in aqueous media and also provide some control over cyclic peptide stacking by salt bridge formation. His, in addition to the participation in hydrogen bonding interactions, should give some chemical control (pH modification) over the assembling process under neutral conditions.14 In the resulting nanotube, the more favourable arrangement (pairwise C, inset Scheme 3) should align all the Arg and Leu residues on top of each other but in opposite sides. This linear arrangement of guanidinium groups in the nanotube should facilitate the deposition of the nanotube on an anionic mica surface. The peptide was synthetized by solid phase synthesis on a trityl resin and cyclized prior to its cleavage by attaching the first dipeptide Fmoc-Lys-δ-Ach-OAllyl through the Lys side-chain (Scheme 2S†).15,16 After cleavage with the TFA cocktail,17 the peptide was precipitated, washed and purified. NMR and MS confirmed the formation of CP7 (Fig. 6S†). IR spectra showed intense bands at 1537 and 1627 cm−1 identified as amide I and amide II, respectively, corresponding to amide bonds involved in hydrogen bonded antiparallel β-sheet structures (Fig. 7S†).13 The amide A stretching band at 3270 cm−1 confirmed the tight ring-to-ring stacked tubular structure with an average inter-subunit distance of 4.75–4.80 Å.18 Nanotube formation in solution was confirmed by using thioflavin T (ThT), a probe used to study amyloid diseases such as Alzheimer, diabetes or prion diseases, that is known to fluoresce upon binding to β-sheet structures.19,20 Addition of increasing amounts of CP7 to an aqueous acidic solution containing ThT (20 μM) did not produce any change in the emission band (excitation 420 nm) at 485 nm (Fig. 8aS†). On the other hand, similar additions of CP7 to a solution at pH 8.1 (in water or TRIS buffer) gave rise to a clear increase in dye emission that confirms the interaction of ThT with cyclic peptide assemblies (Fig. 8bS†). This was observed at CP7 concentrations higher than 50 μM. In any case nanotube formation was confirmed above this concentration under slightly basic conditions in which His residues are mainly deprotonated.
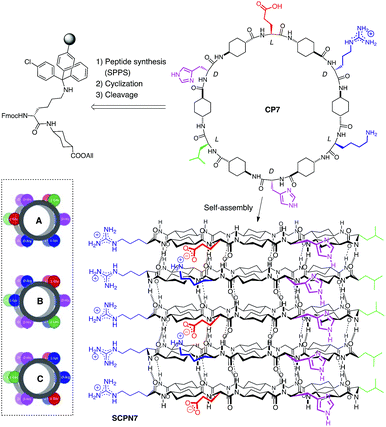 |
| Scheme 3 Structures of cyclic peptide CP7, synthetized on a solid support (trityl resin), and the corresponding SCPN model, in which the CPs are stacked in a antiparallel-like β-sheet structure. The inset shows the top view of the three inter-cyclic peptides pairwise that can be formed between each pair of CPs considering the antiparallel β-sheet structure. | |
Drop casting of an aqueous solution of CP7 in TRIS buffer (2.0 mM, pH ∼ 8.1) on mica afforded fibrous structures with lengths of a few μm and average heights of 3.3–3.9 ± 0.30 nm (Fig. 2a, b and 9S†). Similar results were obtained from aqueous solutions of CP7 at the same pH. These fibrous structures are in keeping with those expected for a nanotube composed of the corresponding dodecapeptide rings.
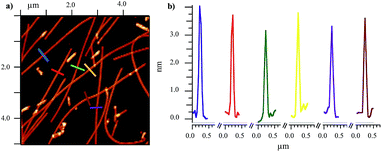 |
| Fig. 2 (a) AFM topography micrographs of SCPN7 deposited over mica (grade V-I muscovite) from aqueous solutions (250 μM, pH ∼ 8.1). (b) AFM height profiles along the transects in different colors shown in (a). | |
2.3 Fullerene encapsulation and peptide molecular peapods
Computational models suggest that the hydrophobic properties and diameter size of the α,δ-SCPN made of twelve residues, such as CP7, would be suitable for the encapsulation of fullerene to form peptide nanopeapod-type structures (Fig. 3c).21–23 Therefore, 1.5 mL of a water solution of CP7 (1.0 mM) at pH 8.1 was added to an Eppendorf tube containing C60 (0.5 mg) and then sonicated for 40 min. The resulting solution was centrifuged (7500 rpm) for 10 min to afford a brownish aqueous solution that was stable for few weeks. The UV/vis spectrum shows the absorption band at 345 nm characteristic of buckminsterfullerene dispersed in water as almost individual molecules (Fig. 10S†).24 Considering the proposed UV-vis molar extinction coefficients in aqueous media [30.000–60.000 M−1 cm−1 for the λmax (360)], we estimated a C60 concentration of 70 μM.25 In addition to this band, long wavelength absorption bands between 400 and 480 nm were also observed. They have been assigned to the interaction between different C60. This suggests that the entrapped fullerenes must be aligned inside the SCPN7. The UV absorption spectrum characteristic of the C60 moiety was essentially the same with time. It is worth mentioning that acidic solutions (pH 3.5) containing similar CP concentration (1.5 mM) or acidic (pH 3.5) or basic aqueous (pH 8.1) solutions did not dissolve C60 under similar conditions. This confirms the requirement of SCPN7 formation for dissolving the fullerene. In addition, the disassembly of peptide nanotubes by acidification of the basic solution containing CP7 and C60 produces the precipitation of the encapsulated fullerene. This suggests, considering the CP concentration used in the solubilization (1.0 mM), that one molecule of C60 was dissolved per each twenty molecules of CP7.
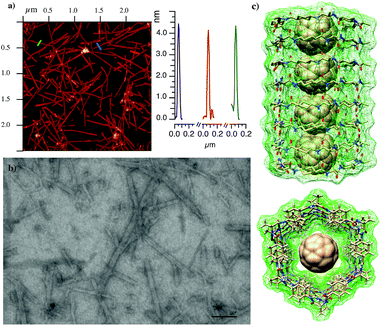 |
| Fig. 3 (a) AFM topography micrographs from aqueous solutions of CP7 and C60 deposited over mica (100 μM, pH ∼ 8.1) and AFM height profiles along the transects in different colors. (b) STEM image of an aqueous solution of CP7 and C60 after deposition on a carbon holey grid (scale bar 100 nm). (c) Side and top views of a computer assisted model of C60 encapsulated in the inner cavity of SCPN7. | |
Additions of the basic solution containing CP7 and C60 to a TRIS solution containing ThT (20 μM) showed the characteristic emission at 485 nm that confirms the β-sheet structure characteristic of peptide nanotubes (Fig. 11S†). Finally, FTIR spectra showed intense bands at 1542, 1620 and 3277 cm−1 that, once again, confirmed the SCPN formation (Fig. 12S†).13
The AFM images of the basic solution of CP7 and C60 also showed a few μm long horizontal fibrous structures with heights of 3.9–4.5 ± 0.41 nm slightly higher to those observed for the solutions of the CP alone (Fig. 3a and 13S†), perhaps as a consequence of the higher rigidity of the cyclic peptide peapod structure.26 No spherical particles of 1 nm in height deposited on the nanotube were observed in any case, confirming that C60 must be encapsulated on the nanotube cavity. These results confirm the ability of the cyclic peptide to assemble into a tubular structure after fullerene encapsulation. Interestingly, the SCPN7 solution was not capable of dissolving C70, perhaps due to its larger size that must not fit in the nanotube cavity following the best Rebek's packing coefficient.27 The solution containing CP7 and C60 was also deposited on a carbon holey grid that appeared in scanning transmission electron microscopy (STEM) images as long fibers of a few μm long that correspond to individual nanotubes (Fig. 3b). Interestingly the deposition of more than one-week aged solutions showed the formation of small nanotube bundles (Fig. 16S†). Similar results were also observed by AFM (Fig. 14S†).
3 Conclusions
We have showed that 4-aminocyclohexanecarboxylic acid (δ-Ach) is an useful building block for the preparation of self-assembling cyclic peptides with large diameter and hydrophobic internal cavities. δ-Ach is a rigid amino acid in which trans-1,4 disposition makes it a suitable component of cyclic peptides that must adopt a flat conformation. Especially suited for this purpose D,L-α-CPs were transformed into α,δ-hybrids by incorporating δ-Ach residues in tetra-, hexa- and octa-peptides. The resulting peptides can stack through an antiparallel-like interaction. The disposition of two methylene groups of the cyclohexyl moiety towards the lumen of the nanotube cavity provided hydrophobic properties. These hydrophobic properties and the appropriate nanotube internal diameter were efficiently implemented for the encapsulation of fullerene C60. These results provide new supramolecular polymers with tuned assembling properties that might make them suitable for the development of efficient drug delivery platforms. The novel peptide nanopeapod structures described here might find applications in field-effect transistors, magnetic or data storage nanodevices, nanoscale lasers and so on.
Conflicts of interest
There are no conflicts to declare.
Acknowledgements
This work was supported by the Spanish Agencia Estatal de Investigación (AEI) and the ERDF (CTQ2016-78423-R), and by the Xunta de Galicia and the ERDF (EM2014/011, ED431C 2017/25 and Centro singular de investigación de Galicia accreditation 2016-2019, ED431G/09). We also thank the ORFEO-CINCA network and MINECO (CTQ2016-81797-REDC). We thank Dr Carlos Vázquez-Vázquez and Miguel Cuerva (IIT, USC) for their help in the AFM characterization.
Notes and references
- D. Bong, T. Clark, J. R. Granja and M. Ghadiri, Angew. Chem., Int. Ed., 2001, 40, 988–1011 CrossRef PubMed
; R. J. Brea, C. Reiriz and J. R. Granja, Chem. Soc. Rev., 2010, 39, 1448–1456 RSC
; R. Chapman, M. Danial, M. L. Koh, K. A. Jolliffe and S. Perrier, Chem. Soc. Rev., 2012, 41, 6023–6041 RSC
; N. Rodríguez-Vázquez, M. Amorín and J. R. Granja, Org. Biomol. Chem., 2017, 15, 4490–4505 RSC
.
- M. J. Webber, E. A. Appel, E. W. Meijer and R. Langer, Nat. Mater., 2016, 15, 13–26 CrossRef PubMed
; R. Dong, Y. Zhou, X. Huang, X. Zhu, Y. Lu and J. Shen, Adv. Mater., 2014, 27, 498–526 CrossRef PubMed
; S. Koutsopoulos, J. Biomed. Mater. Res., 2016, 104, 1002–1016 CrossRef PubMed
; A. Fuertes, M. Juanes, J. R. Granja and J. Montenegro, Chem. Commun., 2017, 53, 7861–7871 RSC
.
- D. J. Rubin, S. Amini, F. Zhou, H. Su, A. Miserez and N. S. Joshi, ACS Nano, 2015, 9, 3360–3368 CrossRef PubMed
; L. Ruiz, P. VonAchen, T. D. Lazzara, T. Xu and S. Keten, Nanotechnology, 2013, 24, 195103 CrossRef PubMed
.
- D. J. Rubin, H. T. Nia, T. Desire, P. Q. Nguyen, M. Gevelber, C. Ortiz and N. S. Joshi, Biomacromolecules, 2013, 14, 3370–3375 CrossRef PubMed
.
- M. R. Ghadiri, J. R. Granja and L. K. Buehler, Nature, 1994, 369, 301–304 CrossRef PubMed
; J. R. Granja and M. R. Ghadiri, J. Am. Chem. Soc., 1994, 116, 10785–10786 CrossRef
; S. Fernandez-Lopez, H. S. Kim, E. C. Choi, M. Delgado, J. R. Granja, A. Khasanov, K. Kraehenbuehl, G. Long, D. A. Weinberger, K. M. Wilcoxen and M. R. Ghadiri, Nature, 2001, 412, 452–456 CrossRef PubMed
; M. Richman, S. Wilk, M. Chemerovski, S. K. T. S. Wärmländer, A. Wahlström, A. Gräslund and S. Rahimipour, J. Am. Chem. Soc., 2013, 135, 3474–3484 CrossRef PubMed
; Y. Wang, S. Yi, L. Sun, Y. Huang, S. C. Lenaghan and M. Zhang, J. Biomed. Nanotech., 2014, 10, 445–454 CrossRef
; M. Danial, C. M. N. Tran, K. A. Jolliffe and S. Perrier, J. Am. Chem. Soc., 2014, 136, 8018–8026 CrossRef PubMed
; M. Li, M. Ehlers, S. Schlesiger, E. Zellermann, S. K. Knauer and C. Schmuck, Angew. Chem., Int. Ed., 2016, 55, 598–601 CrossRef PubMed
; J. Chen, B. Zhang, F. Xia, Y. Xie, S. Jiang, R. Su, Y. Lu and W. Wu, Nanoscale, 2016, 8, 7127–7136 RSC
; Y. Zhao, L. J. Leman, D. J. Search, R. A. Garcia, D. A. Gordon, B. E. Maryanoff and M. R. Ghadiri, ACS Cent. Sci., 2017, 3, 639–646 CrossRef PubMed
; S. C. Larnaudie, J. C. Brendel, I. Romero-Canelón, C. Sanchez-Cano, S. Catrouillet, J. Sanchis, J. P. C. Coverdale, J.-I. Song, A. Habtemariam, P. J. Sadler, K. A. Jolliffe and S. Perrier, Biomacromolecules, 2017, 19, 239–247 CrossRef PubMed
.
- C. Steinem, A. Janshoff, M. S. Vollmer and M. R. Ghadiri, Langmuir, 1999, 15, 3956–3964 CrossRef
; M. S. Vollmer, T. D. Clark, C. Steinem and M. R. Ghadiri, Angew. Chem., Int. Ed., 1999, 38, 1598–1601 CrossRef PubMed
; W. S. Horne, N. Ashkenasy and M. R. Ghadiri, Chem.–Eur. J., 2005, 11, 1137–1144 CrossRef PubMed
; N. Ashkenasy, W. S. Horne and M. R. Ghadiri, Small, 2006, 2, 99–102 CrossRef PubMed
; J. Couet, J. D. J. S. Samuel, A. Kopyshev, S. Santer and M. Biesalski, Angew. Chem., Int. Ed., 2005, 44, 3297–3301 CrossRef PubMed
; R. de la Rica, C. Pejoux and H. Matsui, Adv. Funct. Mater., 2011, 21, 1018–1026 CrossRef PubMed
; T. Xu, N. Zhao, F. Ren, R. Hourani, M. Tsang Lee, J. Y. Shu, S. Mao and B. A. Helms, ACS Nano, 2011, 2, 1376–1384 CrossRef PubMed
; M. Mizrahi, A. Zakrassov, J. Lerner-Yardeni and N. Ashkenasy, Nanoscale, 2012, 4, 518–524 RSC
; M. Danial, C. M.-N. Tran, P. G. Young, S. E. B. Perrier and K. A. Jolliffe, Nat. Commun., 2013, 4, 2780 CrossRef PubMed
; M. Potnuru and N. Madhavan, Polym. Chem., 2016, 7, 31–35 RSC
.
- M. R. Ghadiri, J. R. Granja, R. A. Milligan, D. E. McRee and N. Khazanovich, Nature, 1993, 366, 324–327 CrossRef PubMed
; J. D. Hartgerink, J. R. Granja, R. A. Milligan and M. R. Ghadiri, J. Am. Chem. Soc., 1996, 118, 43–50 CrossRef
; N. Khazanovich, J. R. Granja, D. E. McRee, R. A. Milligan and M. R. Ghadiri, J. Am. Chem. Soc., 1994, 116, 6011–6012 CrossRef
; M. R. Silk, J. Newman, J. C. Ratcliffe, J. F. White, T. Caradoc-Davies, J. R. Price, S. X. B. Perrier, P. E. Thompson and D. K. Chalmers, Chem. Commun., 2017, 53, 6613–6616 RSC
.
- D. Seebach, J. L. Matthews, A. Meden, T. Wessels, C. Baerlocher and L. B. McCusker, Helv. Chim. Acta, 1997, 80, 173–182 CrossRef
; S. Leclair, P. Baillargeon, R. Skouta, D. Gauthier, Y. Zhao and Y. L. Dory, Angew. Chem., Int. Ed., 2004, 43, 349–353 CrossRef PubMed
; W. S. Horne, C. D. Stout and M. R. Ghadiri, J. Am. Chem. Soc., 2003, 125, 9372–9376 CrossRef PubMed
; A. Ghorai, S. K. Reddy, B. Achari and P. Chattopadhyay, Org. Lett., 2014, 16, 3196–3199 CrossRef PubMed
.
- M. Amorin, L. Castedo and J. R. Granja, J. Am. Chem. Soc., 2003, 125, 2844–2845 CrossRef PubMed
; J. Montenegro, C. Vázquez-Vázquez, A. Kalinin, K. E. Geckeler and J. R. Granja, J. Am. Chem. Soc., 2014, 136, 2484–2491 CrossRef PubMed
; M. Cuerva, R. García-Fandiño, C. Vázquez-Vázquez, M. A. López-Quintela, J. Montenegro and J. R. Granja, ACS Nano, 2015, 9, 10834–10843 CrossRef PubMed
; A. Fuertes, H. L. Ozores, M. Amorín and J. R. Granja, Nanoscale, 2017, 9, 748–753 RSC
.
- C. Reiriz, C. Amorín, R. García-Fandiño, L. Castedo and J. R. Granja, Org. Biomol. Chem., 2009, 7, 4358–4361 RSC
; R. Hourani, C. Zhang, R. van der Weegen, L. Ruiz, C. Li, S. Keten, B. A. Helms and T. Xu, J. Am. Chem. Soc., 2011, 133, 15296–15299 CrossRef PubMed
; N. Rodríguez-Vázquez, R. García-Fandiño, M. Amorín and J. R. Granja, Chem. Sci., 2016, 7, 183–187 RSC
; N. Rodríguez-Vázquez, M. Amorín, I. Alfonso and J. R. Granja, Angew. Chem., Int. Ed., 2016, 55, 4504–4508 CrossRef PubMed
; N. Rodríguez-Vázquez, R. García-Fandiño, M. J. Aldegunde, J. Brea, M. I. Loza, M. Amorin and J. R. Granja, Org. Lett., 2017, 19, 2560–2563 CrossRef PubMed
.
- W. B. Liechty, D. R. Kryscio, B. V. Slaughter and N. A. Peppas, Annu. Rev. Chem. Biomol. Eng., 2010, 1, 149–173 CrossRef PubMed
; M. McKenzie, D. Betts, A. Suh, K. Bui, L. D. Kim and H. Cho, Molecules, 2015, 20, 20397–20408 CrossRef PubMed
;
D. Douroumis and A. Fahr, Drug Delivery Strategies for Poorly Water-Soluble Drugs, John Wiley & Sons, Ltd., Chichester, West Sussex, 2012 Search PubMed
.
- M. R. Ghadiri, K. Kobayashi, J. R. Granja, R. K. Chadha and D. E. McRee, Angew. Chem., Int. Ed., 1995, 34, 93–95 CrossRef
; T. D. Clark, J. M. Buriak, K. Kobayashi, M. P. Isler, D. E. McRee and M. R. Ghadiri, J. Am. Chem. Soc., 1998, 120, 8949–8962 CrossRef
; X. C. Sun and G. P. Lorenzi, Helv. Chim. Acta, 1994, 77, 1520–1526 CrossRef
.
- P. I. Haris and D. Chapman, Biopolymers, 1995, 37, 251–263 CrossRef PubMed
; S. Krimm and J. Bandekar, Adv. Protein Chem., 1986, 38, 181–364 CrossRef PubMed
; J. Bandekar, Biochim. Biophys. Acta, 1992, 1120, 123–143 CrossRef
.
- Y. Le Duc, M. Michau, A. Gilles, V. Gence, Y. M. Legrand, A. van der Lee, S. Tingry and M. Barboiu, Angew. Chem., Int. Ed., 2011, 50, 11366–11372 CrossRef PubMed
; A. Méndez-Ardoy, J. R. Granja and J. Montenegro, Nanoscale Horiz., 2018, 3, 391–396 RSC
.
- F. Albericio and F. García-Martín, Chem. Today, 2008, 26, 29–34 Search PubMed
.
- C. J. White and A. K. Yudin, Nat. Chem., 2011, 3, 509–524 CrossRef PubMed
; J. Alsina, F. Rabanal, E. Giralt and F. Albericio, Tetrahedron Lett., 1994, 35, 9633–9636 CrossRef
; P. Romanovskis and A. F. Spatola, J. Peptide Res., 1998, 52, 356–374 CrossRef PubMed
.
- C. Reiriz, R. J. Brea, R. Arranz, J. L. Carrascosa, A. Garibotti, B. Manning, J. M. Valpuesta, R. Eritja, L. Castedo and J. R. Granja, J. Am. Chem. Soc., 2009, 131, 11335–11337 CrossRef PubMed
.
- H. S. Kim, J. D. Hartgerink and M. R. Ghadiri, J. Am. Chem. Soc., 1998, 120, 4417–4424 CrossRef
.
- M. Krebs, E. Bromley and A. Donald, J. Struct. Biol., 2005, 149, 30–37 CrossRef PubMed
; N. Byrne, D. Menzies, N. Goujon and M. Forsyth, Chem. Commun., 2013, 49, 7729–7732 RSC
; H. Levine III, Arch. Biochem. Biophys., 1997, 342, 306–316 CrossRef PubMed
.
- M. Groenning, J. Chem. Biol., 2009, 3, 1–18 CrossRef PubMed
; H. Naiki, K. Higuchi, M. Hosokawa and T. Takeda, Anal. Biochem., 1989, 177, 244–249 CrossRef PubMed
.
- G. D. Pantoş, J.-L. Wietor and J. K. M. Sanders, Angew. Chem., Int. Ed., 2007, 46, 2238–2240 CrossRef PubMed
; D. Canevet, E. M. Pérez and N. Martín, Angew. Chem., Int. Ed., 2011, 50, 9248–9259 CrossRef PubMed
.
- B. W. Smith, M. Monthioux and D. E. Luzzi, Nature, 1998, 396, 323–324 CrossRef
; G. Zhang, R. Zhou and X. C. Zeng, J. Mater. Chem. C, 2013, 1, 4518–4526 RSC
; K. Hirahara, S. Bandow, K. Suenaga, H. Kato, T. Okazaki, H. Shinohara and S. Iijima, Phys. Rev. B: Condens. Matter Mater. Phys., 2001, 64, 115420 CrossRef
; J. Lee, H. Kim, S. J. Kahng, G. Kim, Y. W. Son, J. Ihm, H. Kato, Z. W. Wang, T. Okazaki, H. Shinohara and Y. Kuk, Nature, 2002, 415, 1005–1008 CrossRef PubMed
; D. A. Britz, A. N. Khlobystov, K. Porfyrakis, A. Ardavan and G. A. D. Briggs, Chem. Commun., 2005, 37–39 RSC
.
- T. Kawase, K. Tanaka, N. Fujiwara, H. R. Darabi and M. Oda, Angew. Chem., Int. Ed., 2003, 42, 1624–1628 CrossRef PubMed
; T. Kawase, K. Tanaka, N. Shiono, Y. Seirai and M. Oda, Angew. Chem., Int. Ed., 2004, 43, 17221–11724 Search PubMed
; Y. Shoji, K. Tashiro and T. Aida, J. Am. Chem. Soc., 2006, 128, 10690–10691 CrossRef PubMed
; E.-X. Zhang, D.-X. Wang, Q.-Y. Zheng and M.-X. Wang, Org. Lett., 2008, 10, 2565–2568 CrossRef PubMed
; J. Song, N. Aratani, H. Shinokubo and A. Osuka, J. Am. Chem. Soc., 2010, 132, 16356–16357 CrossRef PubMed
; S. Hitosugi, W. Nakanishi, T. Yamasaki and H. Isobe, Nat. Commun., 2011, 2, 17646 Search PubMed
; T. Iwamoto, Y. Watanabe, T. Sadahiro, T. Haino and S. Yamago, Angew. Chem., Int. Ed., 2011, 50, 8342–8344 CrossRef PubMed
.
- X. Chang and P. J. Vikesland, Environ. Sci. Technol., 2011, 45, 9967–9974 CrossRef PubMed
; F. Diederich, J. Effing, U. Jonas, L. Jullien, T. Plesnivy, H. Ringsdor, C. Thilgen and D. Weinstein, Angew. Chem., Int. Ed., 1992, 31, 1599–1602 CrossRef
; H. Ajie, M. M. Alvarez, S. J. Anz, R. D. Beck, F. Diederich, K. Fostiropoulos, D. R. Huffman, W. Kraetschmer, Y. Rubin, K. E. Schriver, D. Sensharma and R. L. Whetten, J. Phys. Chem., 1990, 94, 8630–8633 CrossRef
; W. Kratschmer, L. D. Lamb, K. Fostiropoulos and D. R. Huffman, Nature, 1990, 347, 354–358 CrossRef
.
- J. A. Sene, M. V. B. Pinheiro, K. Krambrock and P. J. S. Barbeira, Talanta, 2009, 78, 1503–1507 CrossRef PubMed
; S. J. Vance, V. Desai, B. O. Smith, M. W. Kennedy and A. Cooper, Biophys. Chem., 2016, 214–215, 27–32 CrossRef PubMed
.
- AFM image of a control experiment in which a basic solution of CP7 was sonicated for 40 min and then deposited over mica, showing sorter nanotube structures with similar heights to those observed for the solutions of the CP alone without sonication, see Fig. 15S.†.
- S. Mecozzi and J. Rebek Jr, Chem.–Eur. J., 1998, 4, 1016–1022 CrossRef
.
Footnote |
† Electronic supplementary information (ESI) available: Including synthetic experimental details, DFT calculation details and additional figures (relevant NMR data) and schemes (synthetic schemes for peptide and amino acid preparation). See DOI: 10.1039/c8sc02276c |
|
This journal is © The Royal Society of Chemistry 2018 |
Click here to see how this site uses Cookies. View our privacy policy here.