Efficient hypochlorous acid (HClO) production via photoelectrochemical solar energy conversion using a BiVO4-based photoanode†
Received
16th September 2017
, Accepted 11th October 2017
First published on 11th October 2017
Abstract
A BiVO4/WO3/FTO (FTO: fluorine doped tin oxide coated glass) multilayer photoelectrode was found to be suitable for photoelectrochemical oxidative hypochlorous acid (HClO) production from chloride ions (Cl−). HClO was efficiently produced in the electrolyte solution by irradiating the BiVO4/WO3/FTO photoanode with simulated solar light. It was expected that Cl− would be oxidized to chlorine (Cl2) by the photogenerated holes on the BiVO4, and then converted to HClO via the disproportionation reaction in an aqueous electrolyte solution. For the first 100 s, the current efficiency for HClO production was 97%, indicating that the photogenerated hole oxidized Cl− with high selectivity in preference to H2O. Moreover, the BiVO4/WO3/FTO multilayer photoanode enabled us to achieve significant diminution of the external bias required for HClO production under solar light irradiation.
Introduction
Hypochlorous acid (HClO) is commonly used worldwide for the disinfection of drinking water, bleaching, cleaning, deodorizing, treatment of dye wastewater, and sterilization of food, because of its strong oxidation power in aqueous solutions.1–3 According to World Health Organization (WHO) guidelines,4 drinking water should contain at least 2.0 mg L−1 of free chlorine. It is well-known that Cl2 is easily dissolved in an aqueous solution, and that a disproportionation reaction of Cl2 in aqueous media leads to the formation of hypochlorous acid. The molar fractions of Cl2, HClO, and hypochlorite ion (ClO−) are altered by the pH value of the aqueous solution in accordance with a thermodynamic equilibrium5,6 (eqn (1) and (2); see also Fig. S1† for molar fractions plotted against pH). | Cl2 + H2O → HClO + H+ + Cl−, K1 = 3.94 × 10−4 M2 | (1) |
| HClO → H+ + ClO−, K2 = 2.62 × 10−8 M | (2) |
From an electrochemical perspective, the formation of one mole of Cl2 can be considered equivalent to the production of a mole of either HClO or ClO−, all of which are two-electron oxidation products of Cl−. In the present study, Cl2, HClO, and ClO− are unified as HClO for convenience. The electrochemical HClO production process, also known as the chlor-alkali process, is adequate for practical use, because sufficiently high current efficiencies (above 95%) had already been achieved using RuO2 and Ti-based stable anodes.5,6 The chlor-alkali process generally involves Cl− oxidation at the anode (eqn (3)) and H2 production (eqn (4)) or O2 reduction at the cathode (eqn (5)).5,6
| 2Cl− → Cl2 + 2e−, E° = +1.39 V vs. NHE | (3) |
| 2H+ + 2e− → H2, E° = 0.0 V vs. NHE | (4) |
| O2 + 4e− + 4H+ → 2H2O, E° = +1.23 V vs. NHE | (5) |
where the values of the standard oxidation potential are given in volts relative to the normal hydrogen electrode (NHE). The total cell reaction in the chlor-alkali process is described by
eqn (6) and
(7).
5,6 | 2NaCl + 2H+ → Cl2 + H2 + 2Na+, ΔG = 347 kJ mol−1 (at pH = 7.0) | (6) |
| 2NaCl + 0.5O2 + 2H+ → Cl2 + H2O + 2Na+, ΔG = 110 kJ mol−1 (at pH = 7.0) | (7) |
According to the literature,6,7 the chlor-alkali process (for industrial HClO production) requires a huge supply of electrical energy. Therefore, there are environmental and economic benefits to reducing energy consumption in industrial HClO production, for example, by utilizing renewable energy sources such as solar light.
Photoelectrochemical reactions currently attract considerable attention in the research field of energy conversion, as they represent a promising technique for converting solar (light) energy directly into chemical energy.8–16 In particular, there have been substantial developments in the photoelectrochemical H2 production process (conversion of solar energy into H2) over the past decade, including significant improvements in energy conversion efficiency.17–25 A WO3 thin film coated on fluorine doped tin oxide conductive glass (FTO) exhibits the activity for photoelectrochemical splitting of water into H2 and O2 when an external bias is applied.21,26 In addition to solar-driven H2 evolution, the development of the oxidation reaction is necessary to utilize solar energy effectively. In 1987, Grätzel et al. found that the photocurrent–potential curve of a WO3 photoanode was altered by the presence of various ions, including Cl−, Br−, HSO4−, Ce3+, and Fe2+, which are capable of being oxidized instead of H2O.27 Our research group recently reported that a WO3/FTO photoanode could produce high added-value chemicals such as persulfate (S2O82−), tetravalent cerium (Ce4+), and periodide (IO4−) in addition to O2. Notably, S2O82− was formed from sulfate (SO42−) over the WO3 photoanode at a current efficiency of almost 100%, accompanied by the production of a stoichiometric amount of H2 on the cathode.28 High added-value oxidation products, including S2O82−, Ce4+, IO4−, and HClO, which are the desired products in the present study, are known as strong oxidizing reagents.29 It has been widely reported that a BiVO4-based photoelectrode can respond to visible light below approximately 520 nm, which is a longer wavelength than the absorption edge of WO3 corresponding to its band-gap excitation.30 In fact, we reported previously that a BiVO4/WO3/FTO multilayer photoelectrode exhibited high activity for the photoelectrochemical splitting of water containing bicarbonate ions (HCO3−) under solar light irradiation with low external bias,31,32 indicating that an improvement in photoelectrochemical properties corresponds to an expansion of the light absorption range. Therefore, applying the BiVO4/WO3/FTO photoelectrode to the production of high added-value chemicals by utilizing solar energy conversion should result in significant improvements in the amounts of the desired chemicals produced, while suppressing external energy input.33,34 Moreover, solar-driven HClO production from NaCl aqueous solution in accordance with eqn (6) or (7), which is an uphill reaction (ΔG > 0), can be thought of as a type of artificial photosynthesis.
Improving the selectivity for HClO production is one of the major issues in the development of the chlor-alkali process, as the oxidation of H2O to O2 (eqn (8)) takes place competitively at the anode.6
| 2H2O → O2 + 4H+ + 4e−, E° = +1.23 V vs. NHE | (8) |
It is known that the decomposition of HClO (eqn (9)) and the formation of further oxidation products (eqn (10)–(13)), such as chlorate (ClO3−) or perchlorate (ClO4−), decrease the selectivity for HClO production.
| 2HClO → 2H+ + 2Cl− + O2 | (9) |
| 0.5Cl2 + 3H2O → ClO3− + 6H+ + 5e−, E° = +1.47 V vs. NHE | (10) |
| Cl− + 3H2O → ClO3− + 6H+ + 6e−, E° = +1.45 V vs. NHE | (11) |
| 0.5Cl2 + 4H2O → ClO4− + 8H+ + 7e−, E° = +1.39 V vs. NHE | (12) |
| Cl− + 4H2O → ClO4− + 8H+ + 8e−, E° = +1.39 V vs. NHE | (13) |
Moreover, the formation of ClO3− or ClO4−, which is toxic, is undesirable in photoelectrochemical reactions. Zou et al. reported solar H2 evolution from sea water using a BiVO4-based photoelectrode, and found that Cl2 (HClO) was produced as an oxidation product at a current efficiency of less than 30%, indicating that the majority of photogenerated holes oxidized not Cl− but H2O; O2 was produced as a major oxidation product.35 In the present study, photoelectrochemical oxidative HClO production from a Cl− electrolyte under solar light irradiation was investigated using a BiVO4/WO3/FTO photoelectrode, with the aim of achieving high current efficiency (high selectivity) and low external bias.
Experimental section
Preparation of the photoelectrode
The BiVO4/WO3/FTO multilayer photoelectrode was prepared by a step-by-step spin-coating method. Prior to photoelectrode fabrication, three kinds of precursor solutions were obtained in accordance with our previous reports.33,34,36 Solution A (1.0 g tungsten hexachloride [WCl6, Kojundo Chemical Laboratory Co., Ltd] dissolved in 5.0 mL of dimethyl formamide [DMF, Wako Pure Chemical Industries, Ltd.]) and Solution B (0.5 g WCl6 dissolved in 5.0 mL DMF) were prepared under an Ar atmosphere. Solution C was prepared by dissolving bismuth oxide (BiO1.5) solution [Symetrix Co., USA], vanadium oxide (VO2.5) solution [Symetrix Co., USA], and ethyl cellulose [Sigma-Aldrich Co. LLC] in ethyl acetate [Wako Pure Chemical Industries, Ltd.] with a Bi/V molar ratio of 1.0. First, a WO3/FTO electrode was fabricated via two-step spin coating. 200 μL of Solution A was coated on FTO (Nippon Sheet Glass Co., Ltd., 24 × 60 mm) at a rotation rate of 1000 rpm for 15 s, and calcined at 773 K under an ambient air atmosphere for 30 min. Subsequently, Solution B was coated on the same electrode using the same procedure, to obtain a WO3/FTO electrode with a double layer of WO3 on the FTO (referred to subsequently as the ‘under layer electrode’). The BiVO4 photocatalyst layer was prepared as follows: 400 μL of Solution C was coated on the under layer electrode at a rotation rate of 500 rpm for 15 s, followed by calcination at 823 K under an air atmosphere for 30 min, which yielded a BiVO4/WO3/FTO multilayer photoelectrode. A BiVO4 single-layer electrode (without the WO3 under layer) was fabricated on the FTO substrate using the same procedure, but omitting the WO3 coating steps. Prior to the above fabrication procedure, the FTO substrate was rinsed with pure water, ethanol, and ethyl acetate, and then polished in an ultraviolet (UV)-ozone cleaning system (Filgen Inc., PL1101N-14) for 30 min. The prepared BiVO4/WO3/FTO multilayer photoelectrode was characterized by X-ray diffraction (XRD) using an X-ray diffractometer (Empyrean, PANalytical) with Cu Kα radiation.
Photoelectrochemical HClO production
Oxidative photoelectrochemical HClO production was performed under solar light irradiation using the prepared BiVO4/WO3/FTO multilayer photoelectrode (irradiation area: ca. 4.0 cm2) as a photoanode in a two-electrode photoelectrochemical cell (Fig. S2†). The BiVO4/WO3/FTO photoanode was immersed in an aqueous NaCl solution (35 mL, various concentrations) and illuminated under simulated solar light (SAN-EI Electric Co., XES-151S) through the required cut-off filter. The intensity of the solar simulator was calibrated to AM-1.5 (1 SUN, 100 mW cm−2) using a spectroradiometer (SOMA Optics, Ltd., S2440) prior to use. The anodic photocurrent value and the external bias versus the cathode (Pt wire) were controlled and monitored using a potentiostat (BAS. Inc., ALS660B). The anode chamber of the Pyrex-divided cell contained a non-conditioned air atmosphere, and an aqueous anolyte solution was gently stirred by magnetic stirring at less than 100 rpm. Gaseous O2 was bubbled into the cathode chamber at a low flow rate (less than a few mL min−1) to enhance O2 reduction on the Pt wire cathode in accordance with eqn (5). The total cell reaction could be expressed as eqn (7). The concentration of HClO produced in the anolyte solution (CHClO) was determined by the N,N-diethyl-p-phenylenediamine (DPD) method.37 It is known that DPD is immediately transformed into the oxidized form, which is red in colour, in the presence of a strong oxidizing reagent such as HClO (Fig. S3a and b†). DPD reagent powder (chlorine comparator, SIBATA Scientific Technology Ltd., Japan) was added to the sample solution (10 mL), which was diluted to a suitable concentration in advance, and the absorbance at 552 nm was measured using a UV-Vis spectrometer (JASCO, V-730) with a quartz cell of path length 10 mm. CHClO was obtained in accordance with eqn (14). | CHClO = Abs552/(2.1 × 10−4) | (14) |
where Abs552 is the absorbance at 552 nm. The absorption coefficient (2.1 × 10−4 M−1 cm−1), which was determined using a standard aqueous KMnO4 solution, was consistent with the previous literature.38 Moreover, the current efficiency of HClO production (ηHClO) in 0.035 L (35 mL) of the electrolyte solution was calculated using eqn (15). | ηHClO (%) = 100 × (2 × CHClO × 0.035)/C/F | (15) |
where C and F denote the electric charge and Faraday constant, respectively. The formation of HClO from Cl− was considered to occur via two-electron oxidation, based on eqn (1) and (3).
Results and discussion
Fig. 1 shows the XRD patterns of (c) the BiVO4/WO3/FTO multilayer photoelectrode with reference to (a) bare FTO glass, (b) the WO3/FTO under layer electrode, and (d) the BiVO4 powder sample. Distinct diffraction peaks derived from the FTO substrate were found in patterns (a), (b), and (c). A sharp peak at 24.4° in patterns (b) and (c) corresponded to WO3.39 Diffraction peaks at 18.9° and 28.9° in patterns (c) and (d) were assigned to the BiVO4 structure.31,36 The BiVO4/WO3/FTO multilayer electrode applied to the photoelectrochemical reaction in this study was equivalent to those used in previous studies. Additional characterizations can be found in the previous literature.31–34,36,40
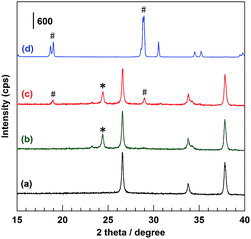 |
| Fig. 1 XRD patterns of the (a) bare FTO substrate, (b) WO3/FTO, (c) BiVO4/WO3/FTO, and (d) BiVO4 powder sample. #: BiVO4 and *: WO3. | |
Fig. 2 displays the time courses of the anodic photocurrent value and CHClO value for the photoelectrochemical Cl− oxidation under solar light irradiation using the BiVO4/WO3/FTO photoanode. By applying +0.50 V of the stable external bias versus the counter electrode, a steady anodic photocurrent at nearly 3.0 mA was observed during solar light irradiation. In the presence of Cl− in an aqueous electrolyte solution, not only H2O but also Cl− is capable of being oxidized by the photogenerated holes on the surface of the photoanode (eqn (3)), and the resulting Cl2 in the aqueous solution should be immediately converted into HClO via the disproportionation reaction (eqn (1)). As shown in Fig. S3c,† the colour of the anolyte solution after adding the DPD reagent gradually became deeper with the photoirradiation. Fig. 2 shows that CHClO linearly increased with the reaction time, and approximately 150 μM of HClO (equivalent to 5.3 mg L−1 as free chlorine) was stored in the anolyte solution after photoirradiation for 900 s. According to the WHO guideline, as discussed in the Introduction section, 5.3 mg L−1 of free chlorine concentration is sufficient for the disinfection of drinking water.
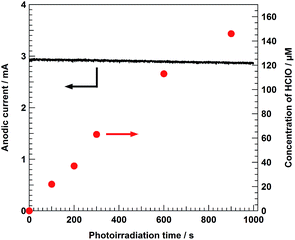 |
| Fig. 2 Time dependence of the anodic current value (left axis) and the concentration of HClO (CHClO, right axis) during the photoelectrochemical Cl− oxidation using the BiVO4/WO3/FTO photoanode. Electrolyte: 5.0 M NaCl aqueous solution, light source: simulated solar light (AM 1.5) with an L-42 cut off filter, external bias: 0.5 V versus the counter electrode, anode chamber: air atmosphere, cathode chamber: O2 atmosphere, and irradiation area: ca. 4.0 cm2. | |
Fig. 3 displays I–V curves of the BiVO4/WO3/FTO photoanode (irradiation area: ca. 4.0 cm2), which were measured under solar light irradiation through an L-42 cut-off filter at various concentrations of NaCl electrolyte solution using a two-electrode photoelectrochemical cell (the same conditions as for the HClO production reaction). No anodic current was obtained under the dark conditions in this external bias range (Fig. 3g). When 5.0 M NaCl electrolyte solution was used, the anodic photocurrent appeared at −0.4 V of the external bias versus the counter electrode; it reached approximately 5.0 mA when +1.4 V was applied (Fig. 3a). The magnitude of the anodic photocurrent was clearly influenced by the concentration of NaCl in an aqueous electrolyte solution, and a large photocurrent value was observed when the concentrated NaCl electrolyte solution was used. It could be considered that the inclination of the I–V curves near the onset potential reflects the solution resistance of electrolyte solutions. As shown in Fig. S4,† the photocurrent value was drastically improved as the ionic strength of the electrolyte solution increased, which is correlated with the solution resistance, although the same concentration of NaCl was contained in both electrolyte solutions (Fig. S4b and d†), whereas there was no difference in the value of ηHClO between the two (approx. 40%). On the other hand, the photocurrent value for the 0.5 M NaCl electrolyte solution (Fig. S4a†) was clearly larger than that for 0.1 M NaCl (+0.4 M NaClO4; Fig. S4b†) and 0.5 M NaClO4 (Fig. S4c†), despite both solutions having the same ionic strength as that of the 0.5 M NaCl. These results indicated that the increment of the photocurrent value was caused by both the decrease in solution resistance that occurred with increasing NaCl concentration and the high affinity for the oxidation of Cl− (two-electron oxidation), compared with the oxidation of H2O (four-electron oxidation), over the BiVO4/WO3/FTO photoanode.
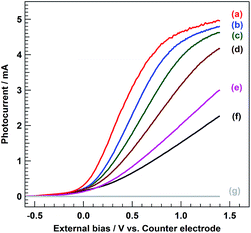 |
| Fig. 3
I–V curves of the BiVO4/WO3/FTO photoelectrode measured in (a) 5.0 M, (b) 3.0 M, (c) 1.0 M, (d) 0.5 M, (e) 0.15 M, and (f) 0.1 M NaCl aqueous solution. (g) Dark conditions (without photoirradiation). Light source: simulated solar light (AM 1.5) with an L-42 cut off filter, anode chamber: air atmosphere, cathode chamber: O2 atmosphere, and irradiation area: ca. 4.0 cm2. | |
Table 1 summarizes the current efficiency for HClO production (ηHClO), the external bias versus the counter electrode required to obtain 1.0 mA of the anodic current, and the concentration of HClO (CHClO) produced after 500 s of photoirradiation, in the photoelectrochemical HClO production using various photoelectrodes and electrodes: BiVO4/WO3/FTO, BiVO4/FTO (without the WO3 under layer), WO3/FTO (without the BiVO4 layer), bare FTO, and Pt wire. The BiVO4/WO3/FTO multilayer photoelectrode showed high activity for HClO production under solar light irradiation, and 60 μM of HClO aqueous solution was obtained at an ηHClO of 81.4% after photoirradiation for 500 s (0.5 coulomb of charge was passed). The external bias was only 0.22 V versus the counter electrode, which was consistent with the I–V curve in the case of the 5.0 M NaCl electrolyte solution (Fig. 3a). When the aqueous electrolyte solution contained concentrated NaCl (5.0 M for Table 1), the value of ηHClO in the (photo)electrochemical Cl− oxidation was hardly influenced by the sort of anodes. By contrast, 1.9 V of the external bias was required to obtain 1.0 mA of the anodic current in the case of the BiVO4/FTO (without the WO3 under layer), indicating that a simple BiVO4 photoelectrode was not active for Cl− oxidation by photo-to-current conversion under solar light irradiation. Furthermore, the values for WO3/FTO and the bare FTO were 1.9 and 2.0 V, respectively, whereas a measurable amount of HClO was produced at ηHClO values of 78.4% and 78.5%, respectively. The lack of response to solar light irradiation in the case of the WO3/FTO was considered to be because the WO3 under layer loaded on FTO was too thin to enable the photocatalytic reaction, as evidenced by its absorption spectrum (Fig. S5b†). HClO production under solar light irradiation over the BiVO4/FTO, the WO3/FTO, and the bare FTO, which required large external bias, was deduced to occur not photoelectrochemically but electrochemically. It was also confirmed that the magnitude of external bias has no significant influence on ηHClO in the electrochemical HClO production using these three types of electrodes. In addition, the BiVO4/WO3/FTO electrode required 1.9 V of the external bias to exhibit 1.0 mA anodic current under the dark conditions, which is a similar external bias to that of the bare FTO electrode. Therefore, it was estimated that Cl− oxidation under dark conditions occurred on the FTO substrate. As we reported in previous literature, the electrolyte solution could contact the FTO substrate of the BiVO4/WO3/FTO electrode through its pore structure.33 These results revealed that the multilayer structure consisting of the BiVO4 photocatalyst and the WO3 under layer was necessary to reduce external bias for photoelectrochemical HClO production under solar light irradiation. As discussed in previous reports by our research group, the photo-induced electrons generated on the conduction band of the BiVO4 photocatalyst appear to be transferred to the FTO conductive substrate through the WO3 under layer, which can function as an effective charge-transporting layer.33,34 Therefore, the onset potential for the anodic photocurrent of the BiVO4/WO3/FTO photoelectrode in an NaCl electrolyte solution was clearly lower (more negative) than that of the BiVO4/FTO (without the WO3 under layer). It can be estimated that an increase in roughness of the electrode surface corresponded with loading BiVO4/WO3 layers on the FTO substrate improves the adsorption site of Cl− on the photoelectrode. Unfortunately, the correlation between ηHClO and the surface properties of photoelectrodes could not be discussed in the present study due to the lack of experimental evidence. Currently, we conclude that the BiVO4/WO3/FTO photoanode is essential for achieving a high ηHClO value and low external bias in photoelectrochemical oxidative HClO production under solar light irradiation.
Table 1 Summary of the (photo)electrochemical HClO production using various (photo)electrodes. Electrolyte: 5.0 M NaCl aqueous solution, light source: simulated solar light (AM 1.5) with using L-42 cut off filter, stable current: 1.0 mA, reaction time: 500 s, anode chamber: air atmosphere, cathode chamber: O2 atmosphere, and irradiation area: ca. 4.0 cm2. ηHClO: current efficiency for HClO production, bias: external bias versus the counter electrode required to get 1.0 mA anodic current, and CHClO: concentration of HClO produced in the anolyte
Electrode |
Irradiation |
η
HClO (%) |
Bias/V |
C
HClO/μM |
BiVO4/WO3/FTO |
Solar light |
81.4 |
0.22 |
60 |
BiVO4/WO3/FTO |
Dark |
75.1 |
1.9 |
56 |
BiVO4/FTO |
Solar light |
72.6 |
1.8 |
54 |
WO3/FTO |
Solar light |
78.4 |
2.0 |
58 |
FTO |
Solar light |
78.5 |
1.9 |
58 |
Pt wire |
Solar light |
77.4 |
1.2 |
57 |
The ηHClO value for photoelectrochemical Cl− oxidation using the BiVO4/WO3/FTO photoelectrode was not influenced by the presence of other cations in the anolyte solution (Fig. S6†). Moreover, the formation of HClO was totally suppressed in the absence of Cl− in the electrolyte solution, indicating that the source of produced HClO was Cl− in the photoelectrochemical reaction over the BiVO4/WO3/FTO photoanode. As shown in Fig. S7,†ηHClO for photoelectrochemical Cl− oxidation was not influenced by the magnitude of the external bias. Fig. 4 displays the time dependence of CHClO and ηHClO in photoelectrochemical HClO production using the BiVO4/WO3/FTO photoanode under solar light irradiation with applying 0.5 V versus the counter electrode. The value of ηHClO gradually decreased with photoirradiation time, and finally reached stability (ηHClO = ca. 80%). After photoirradiation for 1800 s, 280 μM of HClO was stored in the electrolyte solution by applying 0.5 V of external bias versus the counter electrode. No other oxyanions, such as chlorate (ClO3−), or perchlorate (ClO4−), were observed in the electrolyte solution by ion chromatography analysis (940 Professional IC Vario, Metrohm AG). Moreover, none of these oxyanions were known to be detected by the DPD test, suggesting that the formation of these oxyanions from Cl− or by further oxidation of HClO rarely occurred under the reaction conditions. Surprisingly, after photoirradiation for 100 s, almost all of the anodic current was derived from Cl− oxidation (ηHClO: 97%), indicating that the oxidation of H2O to O2 was totally suppressed by the presence of Cl−, although a large excess of H2O molecules existed in the electrolyte solution. To our knowledge, this value of 97% current efficiency is the highest reported so far for photoelectrochemical Cl− oxidation. Li et al. reported that photocatalytic splitting of sea water using a TiO2-based photocatalyst produced stoichiometric amounts of H2 and O2, and that the photocatalytic activity in sea water was better than that in pure water.41 They also claimed that the O2 was evolved via the decomposition of HClO, which was produced by the oxidation of Cl− in sea water; however, no mechanistic studies were shown to support this. In fact, photo- or thermal-decomposition of HClO results in the formation of Cl− and O2, in accordance with eqn (9). As shown in Fig. 5, HClO in the aqueous NaCl solution (approximately 600 μM HClO) was gradually decomposed under solar light irradiation even in the absence of the BiVO4/WO3/FTO photoelectrode. Hence, it appears that the decrease in ηHClO during the photoelectrochemical reaction (Fig. 4) is mainly caused by the decomposition of the produced HClO. We can also assume that the final ηHClO value is determined by the equilibrium between the formation and the decomposition rates of HClO during the reaction. Moreover, the decomposition rate of HClO was clearly influenced by the concentration of NaCl in the electrolyte solution; the HClO in the aqueous 0.1 M NaCl solution was decomposed much more quickly than that in the 5.0 M solution. In addition, vigorous stirring of the anolyte solution, which causes quicker decomposition of HClO, also diminished the value of ηHClO. Fig. 6 shows the dependence of NaCl concentration in the electrolyte solution on the value of ηHClO. After photoirradiation for 1000 s, the ηHClO value was less than 40% in the case of 0.1 M NaCl electrolyte solution, whereas it was greater than 80% when 5.0 M NaCl aqueous solution was used. In the case of 5.0 M NaCl electrolyte solution, we can conclude that almost all the photogenerated holes on the valence band of the BiVO4 were consumed to oxidize not H2O but Cl− into Cl2 under solar light irradiation, after which the produced HClO was gradually decomposed. As shown in Fig. 3, the NaCl concentration in the electrolyte solution also alters the I–V characteristics of the BiVO4/WO3/FTO photoelectrode. The solar light-induced photocurrent was significantly improved with the increase in the concentration of NaCl, whereas the onset potential versus the counter electrode for the anodic photocurrent was not influenced. Accordingly, using the concentrated NaCl aqueous solution as an electrolyte solution enabled us to achieve a large value of CHClO, for two reasons. First, the decomposition of HClO during the photoelectrochemical reaction was suppressed in the case of high NaCl concentration. Second, a large photocurrent value results from using the NaCl electrolyte at high concentrations. The total current efficiency for the oxidation reactions (HClO production and O2 evolution) during the photoelectrochemical Cl− oxidation in an aqueous electrolyte solution was found to be approximately 100% for various concentrations of NaCl (Fig. S8†). A trade-off relationship between HClO production and O2 evolution was clearly altered by the NaCl concentration of the electrolyte solution. Moreover, other oxyanions (ClO3− and ClO4−) were not produced at all, as mentioned above. As the ηHClO value for 0.1 M NaCl solution after 100 s of photoirradiation was not sufficiently high (approx. 40%), the oxidation of H2O proceeded with preference for Cl− oxidation in the case of the dilute NaCl electrolyte solution. Improvements in selectivity for Cl− oxidation with increasing NaCl concentration should be one of the most important effects, in addition to the two mechanisms discussed above. However, it should be emphasized that even when 0.5 M NaCl electrolyte solution was used, which is almost the same concentration as sea water, efficient photoelectrochemical HClO production (ηHClO = ca. 80%) was achieved under solar light irradiation by applying a small external bias (Fig. 6).
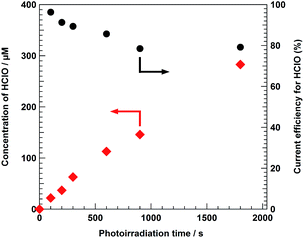 |
| Fig. 4 Time dependence of the current efficiency for HClO production (ηHClO) in the photoelectrochemical Cl− oxidation using the BiVO4/WO3/FTO photoanode. Electrolyte: 5.0 M NaCl aqueous solution, light source: simulated solar light (AM 1.5) with an L-42 cut off filter, external bias: 0.5 V versus the counter electrode, anode chamber: air atmosphere, cathode chamber: O2 atmosphere, and irradiation area: ca. 4.0 cm2. Plots of the concentration of HClO (CHClO, left axis) are same to those shown in Fig. 2. | |
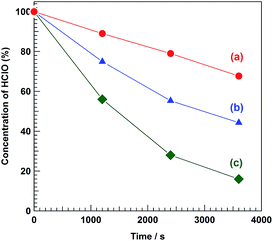 |
| Fig. 5 Decomposition rates of HClO in (a) 5.0 NaCl, (b) 0.5 M NaCl, and (c) 0.1 M NaCl aqueous solution under solar light irradiation. Light source: simulated solar light (AM 1.5) and anode and cathode chamber: ambient air atmosphere. No electrodes were immersed in the anolyte and catholyte, respectively. | |
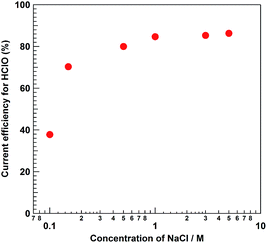 |
| Fig. 6 Dependence of NaCl concentration on the current efficiency for HClO production (ηHClO) in the photoelectrochemical Cl− oxidation using the BiVO4/WO3/FTO photoanode. Electrolyte: 0.1–5.0 M NaCl aqueous solution, light source: simulated solar light (AM 1.5) with an L-42 cut off filter, stable current: 0.5 mA, photoirradiation time: 1000 s, anode chamber: air atmosphere, cathode chamber: O2 atmosphere, and irradiation area: ca. 4.0 cm2. | |
Fig. 7 displays the wavelength dependence of CHClO and ηHClO in photoelectrochemical HClO production over the BiVO4/WO3/FTO photoelectrode. The value of CHClO was markedly decreased by the application of long-wavelength pass-type cut-off filters; for example, the photocurrent under solar light irradiation through a filter of T50 = 500 was not sufficient for storing HClO, which is in accordance with the absorption spectrum shown in Fig. S5a.† On the other hand, in photoelectrochemical Cl− oxidation, the ηHClO value was not affected by the photoirradiation wavelength. Although the photodecomposition of HClO under UV light irradiation is well-known, solar light irradiation was not a critical factor for the decomposition of HClO under the present experimental conditions. Thus, the changes in CHClO, when 5.0 M NaCl was used as an electrolyte solution, brought about by applying various cut-off filters were regulated only by the magnitude of the anodic photocurrent value. This result also affirmed that the positively charged holes on the valence band of the BiVO4, which were generated by band-gap excitation, oxidized Cl− to HClO with high selectivity. After photoirradiation (without applying a filter) for 2000 s, more than 660 μM of HClO was efficiently stored in 5.0 M NaCl electrolyte solution by using the BiVO4/WO3/FTO photoelectrode, and the resulting aqueous solution totally bleached a black melanin dye contained in human hair (Fig. 8). This study successfully demonstrated that the BiVO4/WO3/FTO multilayer photoelectrode effectively produced HClO from aqueous NaCl solution with a small external bias under solar light irradiation. Solar-driven HClO production, which is one type of artificial photosynthesis, could make an important contribution to sustainable development and health in the future.
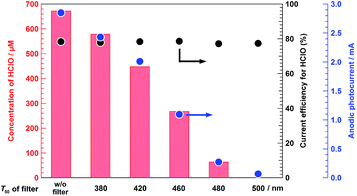 |
| Fig. 7 Irradiation wavelength dependence of the concentration of HClO (CHClO, left axis) and the current efficiency for HClO production (ηHClO) in the photoelectrochemical Cl− oxidation. Electrolyte: 5.0 M NaCl, light source: simulated solar light (AM 1.5) with various filters, external bias: 0.5 V vs. the counter electrode, photoirradiation time: 2000 s, anode chamber: air atmosphere, cathode chamber: O2 atmosphere, and irradiation area: ca. 4.0 cm2. T50 of the filter: wavelength at which transmittance is 50%. w/o filter: full range irradiation (AM 1.5). | |
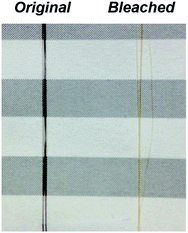 |
| Fig. 8 Photograph of before and after bleaching of human hair by using the photoelectrochemically produced HClO. | |
Conclusions
Our BiVO4/WO3/FTO multilayer photoelectrode exhibited superior activity for photoelectrochemical HClO production under solar light irradiation. The photogenerated holes on the BiVO4 effectively oxidized Cl− to Cl2 in an aqueous electrolyte solution, in preference to H2O oxidation to O2. It appeared that HClO was produced via the hydrolysis of Cl2 (disproportionation reaction of Cl2), and efficiently stored in the concentrated NaCl solution. In addition, unwanted oxyanions (ClO3− and ClO4−) were not produced at all. The current efficiency for HClO production (ηHClO) was 97% for the first 100 s when 5.0 M NaCl electrolyte solution was used. After solar light irradiation for 2000 s, 660 μM of HClO was accumulated in the aqueous electrolyte solution, which is a sufficient concentration for the disinfection of drinking water or use as a bleaching reagent. Moreover, the present study clearly demonstrated that the BiVO4/WO3/FTO photoanode is essential for achieving a high ηHClO value and low external bias for photoelectrochemical oxidative HClO production under solar light irradiation.
Conflicts of interest
There are no conflicts to declare.
Acknowledgements
The present work was partially supported by the International Joint Research Program for Innovative Energy Technology.
References
- W. A. Rutala and D. J. Weber, Clin. Microbiol. Rev., 1997, 10, 597–610 CAS.
- M. Bissen and F. H. Frimmel, Acta Hydrochim. Hydrobiol., 2003, 31, 97–107 CrossRef CAS.
- R. Mikutta, M. Kleber, K. Kaiser and R. Jahn, Soil Sci. Soc. Am. J., 2005, 69, 120–135 CrossRef CAS.
-
World Health Organization, Guidelines for drinking-water quality, 4th edn, 2011, http://www.who.int/water_sanitation_health/publications/2011/dwq_guidelines/en/ Search PubMed.
-
L. R. Czarnetzki, Aspects of electrochemical production of hypochlorite and chlorate, Technische Universiteit Eindhoven, Eindhoven, 1989 Search PubMed.
- R. K. Karlsson and A. Cornell, Chem. Rev., 2016, 116, 2982–3028 CrossRef CAS PubMed.
- I. Moussallem, J. Jörissen, U. Kunz, S. Pinnow and T. Turek, J. Appl. Electrochem., 2008, 38, 1177–1194 CrossRef CAS.
- D. Gust, T. A. Moore and A. L. Moore, Acc. Chem. Res., 2009, 42, 1890–1898 CrossRef CAS PubMed.
- D. G. Nocera, Acc. Chem. Res., 2012, 45, 767–776 CrossRef CAS PubMed.
- A. Mills and S. LeHunte, J. Photochem. Photobiol., A, 1997, 108, 1–35 CrossRef CAS.
- A. Kudo, J. Ceram. Soc. Jpn., 2001, 109, S81–S88 CrossRef CAS.
- A. Kudo and Y. Miseki, Chem. Soc. Rev., 2009, 38, 253–278 RSC.
- F. E. Osterloh, Chem. Soc. Rev., 2013, 42, 2294–2320 RSC.
- Y. B. Kuang, Q. X. Jia, H. Nishiyama, T. Yamada, A. Kudo and K. Domen, Adv. Energy Mater., 2016, 6, 1501645 CrossRef.
- K. Rajeshwar, P. Singh and J. Dubow, Electrochim. Acta, 1978, 23, 1117–1143 CrossRef CAS.
- C. G. Granqvist, Sol. Energy Mater. Sol. Cells, 2000, 60, 201–262 CrossRef CAS.
- T. Bak, J. Nowotny, M. Rekas and C. C. Sorrell, Int. J. Hydrogen Energy, 2002, 27, 991–1022 CrossRef CAS.
- V. M. Aroutiounian, V. M. Arakelyan and G. E. Shahnazaryan, Sol. Energy, 2005, 78, 581–592 CrossRef CAS.
- K. Sayama, T. Oi, R. Abe, M. Yanagida, H. Sugihara and Y. Iwasaki, Sol. Energy Mater. Sol. Cells, 2006, 90, 2429–2437 CrossRef CAS.
- B. D. Alexander, P. J. Kulesza, I. Rutkowska, R. Solarska and J. Augustynski, J. Mater. Chem., 2008, 18, 2298–2303 RSC.
- V. Chakrapani, J. Thangala and M. K. Sunkara, Int. J. Hydrogen Energy, 2009, 34, 9050–9059 CrossRef CAS.
- Z. B. Chen, T. F. Jaramillo, T. G. Deutsch, A. Kleiman-Shwarsctein, A. J. Forman, N. Gaillard, R. Garland, K. Takanabe, C. Heske, M. Sunkara, E. W. McFarland, K. Domen, E. L. Miller, J. A. Turner and H. N. Dinh, J. Mater. Res., 2010, 25, 3–16 CrossRef CAS.
- Y. H. Ng, A. Iwase, A. Kudo and R. Amal, J. Phys. Chem. Lett., 2010, 1, 2607–2612 CrossRef CAS.
- T. Hisatomi, J. Kubota and K. Domen, Chem. Soc. Rev., 2014, 43, 7520–7535 RSC.
- Y. Pihosh, I. Turkevych, K. Mawatari, T. Asai, T. Hisatomi, J. Uemura, M. Tosa, K. Shimamura, J. Kubota, K. Domen and T. Kitamori, Small, 2014, 10, 3692–3699 CrossRef CAS PubMed.
- C. Santato, M. Ulmann and J. Augustynski, J. Phys. Chem. B, 2001, 105, 936–940 CrossRef CAS.
- J. Desilvestro and M. Grätzel, J. Electroanal. Chem. Interfacial Electrochem., 1987, 238, 129–150 CrossRef CAS.
- K. Fuku, N. Wang, Y. Miseki, T. Funaki and K. Sayama, ChemSusChem, 2015, 8, 1593–1600 CrossRef CAS PubMed.
- I. M. Kolthoff and I. K. Miller, J. Am. Chem. Soc., 1951, 73, 3055–3059 CrossRef CAS.
- S. Tokunaga, H. Kato and A. Kudo, Chem. Mater., 2001, 13, 4624–4628 CrossRef CAS.
- R. Saito, Y. Miseki and K. Sayama, Chem. Commun., 2012, 48, 3833–3835 RSC.
- R. Saito, Y. Miseki and K. Sayama, J. Photochem. Photobiol., A, 2013, 258, 51–60 CrossRef CAS.
- K. Fuku and K. Sayama, Chem. Commun., 2016, 52, 5406–5409 RSC.
- K. Fuku, Y. Miyase, Y. Miseki, T. Funaki, T. Gunji and K. Sayama, Chem.–Asian J., 2017, 12, 1111–1119 CrossRef CAS PubMed.
- W. Luo, Z. Yang, Z. Li, J. Zhang, J. Liu, Z. Zhao, Z. Wang, S. Yan, T. Yu and Z. Zou, Energy Environ. Sci., 2011, 4, 4046 CAS.
- H. Tateno, Y. Miseki and K. Sayama, Chem. Commun., 2017, 53, 4378–4381 RSC.
- L. Moberg and B. Karlberg, Anal. Chim. Acta, 2000, 407, 127–133 CrossRef CAS.
- H. Bader, V. Sturzenegger and J. Hoigné, Water Res., 1988, 22, 1109–1115 CrossRef CAS.
- T. Nakajima, A. Hagino, T. Nakamura, T. Tsuchiya and K. Sayama, J. Mater. Chem. A, 2016, 4, 17809–17818 CAS.
- I. Fujimoto, N. N. Wang, R. Saito, Y. Miseki, T. Gunji and K. Sayama, Int. J. Hydrogen Energy, 2014, 39, 5202 CrossRef CAS.
- L. Huang, R. Li, R. Chong, G. Liu, J. Han and C. Li, Catal. Sci. Technol., 2014, 4, 2913 CAS.
Footnote |
† Electronic supplementary information (ESI) available: Molar fraction of Cl2 in an aqueous solution, the photograph of the photoelectrochemical reaction setup, I–V curves, absorption spectra of photoelectrodes, and the results of control experiments. See DOI: 10.1039/c7se00453b |
|
This journal is © The Royal Society of Chemistry 2018 |
Click here to see how this site uses Cookies. View our privacy policy here.