Enhancement of interfacial bioelectrocatalysis in Shewanella microbial fuel cells by a hierarchical porous carbon–silica composite derived from distiller's grains†
Received
20th November 2017
, Accepted 27th December 2017
First published on 2nd January 2018
Abstract
The microbial fuel cell (MFC) is an attractive green energy source since it can use wastewater and/or organic wastes as fuels to generate electricity. A cost-effective anode material with superior bioelectrocatalysis activity is critical to MFC. In this study, a honeycomb-like hierarchical porous carbon–silica (PC/Si) composite with macropores (2–15 μm) and mesopores (3–4 nm) was derived from distiller's grains via a simple carbonization procedure and used as an anode in a Shewanella putrefaciens CN32 (S. putrefaciens CN32) fuel cell for the first time. The obtained three dimensional scaffold structure with meso pores on the wall provides not only a large surface for bacteria adhesion, but also more active sites for flavin mediated interfacial electron transfer. It is interesting that the siliceous crusts of this PC/Si composite greatly promote the bacteria adhesion as well as a uniform biofilm formation. Accordingly, the PC/Si anode delivers a maximum power density of 580.7 mW m−2 in S. putrefaciens CN32 MFC, which is 4.5-fold higher than that of the conventional carbon cloth anode. Considering the synthesis process of this biomass based carbon material is facile, this PC/Si anode could be very promising when applied for high performance, large scale MFC.
1. Introduction
The microbial fuel cell (MFC) as a renewable energy source has received much attention in recent years1,2 as it offers a solution for the production of sustainable energy by recovering electricity and treating organic waste simultaneously in a neutral carbon dioxide scenario.3 This type of bio-electrochemical system (BES) holds promise in a wide field of applications,4 including remote power sources,5 energy recovery from wastewater6,7 and marine sediment8 as well as bio-hydrogen production9,10 and water desalination.11,12 Currently, the low power output and high cost limits its practical application. The current generation performance of a MFC is decisively determined by the density of adhered exoelectrogens with a highly effective extracellular electron transfer (EET). With the high loading amount of the cells and the self-generated electron shuttles in the anode biofilm, the exoelectrogens can substantially facilitate EET between bacteria and electrodes. To achieve a perfect anodic catalysis, various electrode materials, including a hierarchical porous electrode with an enhanced surface area and biocompatible surface decoration, have been developed to increase biofilm formation. Promising power output performances from these MFCs based on nickel foam,13 micro channeled electrodes,14 nanotube-coated textiles and macroporous sponges,15,16 and conductive nanowire networks17 have been reported. However, considering the complicated fabricating processes or involvement of rare precursor materials, the costs of these electrode materials are not acceptable especially for large-scale devices. It is necessary to develop cost-effective anode materials with a green and facile method.
The direct carbonization of biomass wastes provides a sustainable and low-cost way to prepare porous carbon materials. Plant-based biomass wastes possess a naturally porous structure and can be directly carbonized to form porous carbon materials.18,19 Some of these porous materials have been used for energy storage20–23 or as catalyst supports and in CO2 capture24 according to their distinct meso- and micro-porous structures. Recently, new materials with three-dimensional (3D) architectures derived from the biomass have been designed to build a macroporous structure for enhancing the surface area and further used as an anode in MFC.25–28 In addition, besides the porous structure, the surface chemistry of an electrode is also a key factor for microbial electrocatalysis since it will affect the cell adhesion as well as the redox reaction of the electron shuttles. In this work, a 3D porous carbon has been derived from distiller's grains (DG) as an anode in a Shewanella putrefaciens CN32 (S. putrefaciens CN32) MFC for the first time. As a co-product of ethanol production by yeast fermentation of enzymatically hydrolyzed grain starch, a comprehensive utilization of distiller's grains could reduce environmental pollution and save food grain to reduce production costs. The 3D structure of DG could be maintained during a simple carbonization procedure and the final products are porous carbon–silica composites, which possess a very porous structure with nice surface properties for biofilm adhesion. The anodic performance of the 3D carbon material and the electrochemical behavior of the electroactive microbial biofilm grown on the carbon composite surface were systematically investigated and compared. Furthermore, a strong synergistic effect from the siliceous crust chemistry and the hierarchically porous structure to greatly enhance EET is discussed.
2. Experimental
2.1 Preparation of activated carbon from distiller's grains
Preparing a honeycomb-like hierarchical porous carbon–silica composite from DG included washing, drying and carbonization. Firstly, the DG was washed with ethanol and distilled water several times, and then the DG was dried in an oven at 80 °C for 12 h. This was followed by carbonization in a tubular reactor at different temperatures for about 2 h (the heating rate from room temperature to the target temperature was 1 °C min−1) in N2. After cooling to room temperature, the sample in the reactor was removed and ground, and the honeycomb-like hierarchical porous carbon–silica composite was obtained. According to the different carbonization temperatures of 600 °C, 700 °C, 800 °C, and 900 °C, the porous carbon–silica composites were noted as PC/Si-I, PC/Si-II, PC/Si-III and PC/Si-IV, respectively. HF was used to remove silicon from the surface of the PC/Si composite, in detail, 200 mg of PC/Si-II was added 20 mL of 1 M HF with continuous stirring for 3 h, then washed with distilled water and ethanol several times, and dried in a vacuum oven for 24 h; and finally, named as PC/Si-II–HF.
2.2 Material characterization
Field emission scanning electron microscopy (FESEM, JSM-7800F, Japan) was used to investigate the morphology of the as-prepared PC/Si composite and the percentages of the elements in the composite were estimated by an energy dispersive spectrometer (EDS). X-ray diffraction (XRD) measurements were performed on a Shimadzu diffractometer (XRD-7000, Tokyo, Japan) operated in the reflection mode with Cu Kα radiation at a step size of 0.06° per second. Nitrogen adsorption–desorption experiments were carried out at 77.3 K by using an automated gas sorption system (BET, nova 1200e, America). The surface area and pore size distribution were calculated using the Brunauer–Emmett–Teller (BET) equation and Barrett–Joyner–Halenda (BJH) methods, respectively. X-ray photoelectron spectroscopy (XPS) measurements were performed on a Thermo Scientific ESCALAB 250Xi electron spectrometer.
2.3 MFC setup and operation
The bacterial culture, setup and operation of the H-type dual-chamber MFC used by us have been reported in our previous works.2,29 In particular, the powdered materials were mixed with poly(tetrafluoroethylene) solution (1 wt%) to prepare a paste, then coated on both surfaces of carbon cloth (CC, 1 cm × 1 cm) with a loading of about 2 mg cm−2 followed by drying at 100 °C for 3 h. The as-prepared electrode was used as the anode and a carbon fiber brush was used as the cathode. Lactate with a final concentration of 18 mM was added into the anolyte (M9 buffer, Na2HPO4, 6 g L−1; KH2PO4, 3 g L−1; NH4Cl, 1 g L−1; NaCl, 0.5 g L−1; MgSO4, 1 mM; and CaCl2, 0.1 mM) as the sole electron donor and potassium ferricyanide (50 mM) was dissolved in the catholyte (0.01 M phosphate buffer) as the terminal electron acceptor. The MFC was operated at room temperature with an external loading resistance (R) of 1.5 kΩ, and the output voltage was recorded by a digital multimeter. At the steady state of the MFC, the polarization and power density curves were obtained by measuring the stable voltage generated at various external resistances (1–80 kΩ). The surface morphology of the discharged anode was investigated in our previous work.30
2.4 Electrochemical measurements
All the electrochemical experiments were carried out with a potentiostat (CHI660E, Shanghai Chenhua, China) in a three-electrode cell that consisted of a working electrode, a saturated calomel electrode (SCE) as reference electrode, and a platinum foil counter electrode. Electrochemical impedance spectra measurements were performed over a frequency range of 0.1 Hz to 100 kHz at −0.4 V (vs. SCE) with a perturbation signal of 5 mV. All the tests were conducted at room temperature and the CVs were recorded between −0.8 V and 0.6 V (vs. SCE) with a scan rate of 1 mV s−1 after discharge over 12 h under 0.2 V (vs. SCE).
3. Results and discussion
The FESEM images of the porous carbon–silica (PC/Si) composite prepared at different conditions are shown in Fig. 1a–d. It can be found that the PC/Si possesses a porous structure with distributed pore sizes of 0.5–15 μm, which illustrates that the distribution of mesopores and macropores in the DG remain after carbonization. Both the amount and the size of the pores increase as the increase of temperature, but the porous scaffold collapses when the temperature is higher than 700 °C (Fig. 1c and d). The emerging of pore and channel structures may be caused by the fast removal of volatile organic components from the distiller's grains.31,32 From these images, the PC/Si-II (Fig. 1b) seems to possess an ordered porous structure with the largest pore size of all the PC/Si composites. This hierarchical porous structure could provide more room for cell adhesion and a large active surface area for electro catalytic reactions.
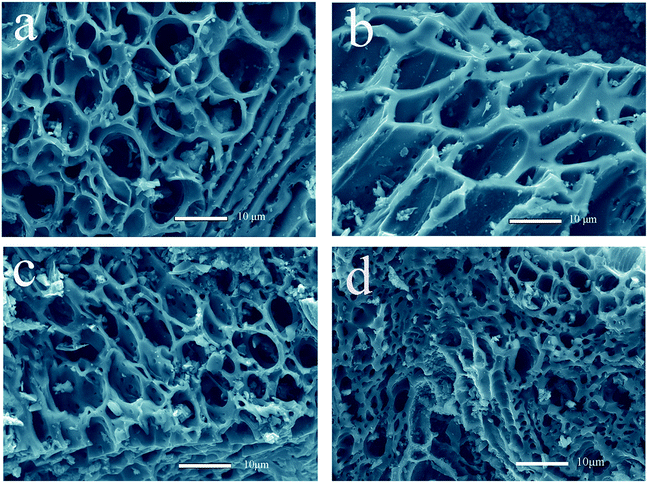 |
| Fig. 1 FESEM images of porous carbon–silica composites derived from distiller's grains carbonized at different temperatures ((a) PC/Si-I, (b) PC/Si-II, (c) PC/Si-III, (d) PC/Si-IV). | |
Interestingly, the results of the EDS and XPS of PC/Si-II shown in Fig. 2a reveal that there are Si and O elements covering the surface of PC/Si-II with the percentages of 19.5% and 27.4%, respectively. From the XPS spectra, the peaks centered at 154.08 eV and 103.27 eV are assigned to Si 2s and Si 2p, respectively. The presence of silicon oxide was further confirmed by the binding energies of the O 1s electrons (Fig. 2b), in which the composite exhibited strong O–Si bonds of SiO2 at 532.7 eV.33,34 The XRD pattern (Fig. 2c) features two diffused peaks at 22.2° and 44.5°, which correspond to the presence of amorphous silica and graphite structures, respectively.32,35 In addition, all the samples show a tiny peak at 26.8°, which corresponds to carbon.36 EDS element mapping micrographs (Fig. S1b–d†) of C, O and Si suggest a very uniform distribution of these three elements. It suggests that silica residues are distributed on the surface of PC/Si-II. Since the siliceous crusts or silica-based surface could attract more bacteria to adsorb on its surface,37–40 and the additional macropores resulting from the intergranular spaces produce an important increase to the overall accessible surface for the bacteria,40 the PC/Si-II is predicted to achieve a superior bioelectrocatalytic activity compared to carbon cloth in the MFC.
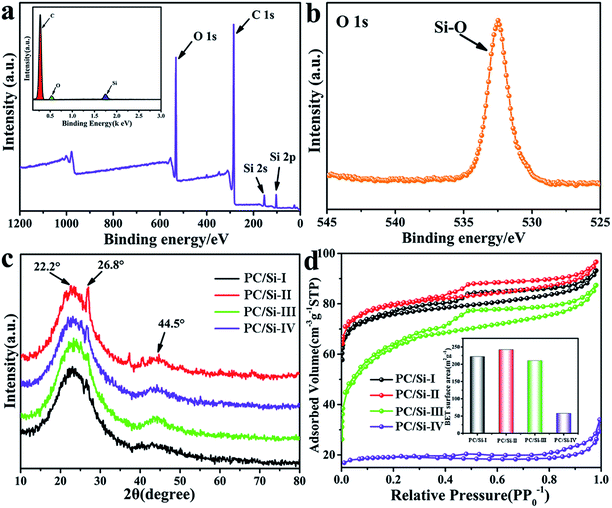 |
| Fig. 2 (a) XPS wide-scanned and EDS spectra (inset of a) of the PC/Si-II surface, (b) survey spectra and binding energies of O 1s electrons of the PC/Si-II surface, (c) XRD patterns of different PC/Si composites, (d) nitrogen adsorption–desorption isotherms of different PC/Si composites (the inset is a histogram of the specific surface areas). | |
The isotherms of the PC/Si composites carbonized at different temperatures are shown in Fig. 2d, in which the inset shows the specific surface area comparison between different temperatures. The PC/Si composite carbonized at 700 °C has the largest the specific surface area (242.4 m2 g−1), which is not surprising since the porous scaffold collapsed at higher temperature (800 °C and 900 °C) as observed in the SEM images. The isotherm has a hysteretic loop, which is a characteristic of the adsorption–desorption of mesopores. The typical pore size distribution of the PC/Si-II composite shown in Fig. S2† indicates that it has a narrow and uniform pore size distribution in the range of 3–4 nm. These mesopores will provide a large active surface area for the electron shuttle reaction so that the PC/Si composite will provide a promising potential for a fast direct electrochemistry of electron mediators.
The electrochemical behaviors of different electrode materials coated on carbon cloth were measured in the anaerobic S. putrefaciens CN32 cell culture. As shown in Fig. 3a, the turnover CV was carried out after discharge at 0.2 V for 12 h at a scanning rate of 1 mV s−1. The inset shows the CV of the PC/Si-II anode with or without a turnover current. It is noted that the PC/Si-II anode possesses the highest catalytic current and has a more negative on-set potential over other anodes. This is mainly because the two advantages of PC/Si-II are based on the hierarchical pore structure and siliceous crust surface for an enhanced biofilm formation and EET efficiency. Firstly, the macro porous structure provides free space for the bacteria to move in and form a biofilm. At the same time, the mesopores can trap the electron shuttles (flavins) in and allow the two electroactive sites to simultaneously access the electrode surface for a direct two-electron transfer.41
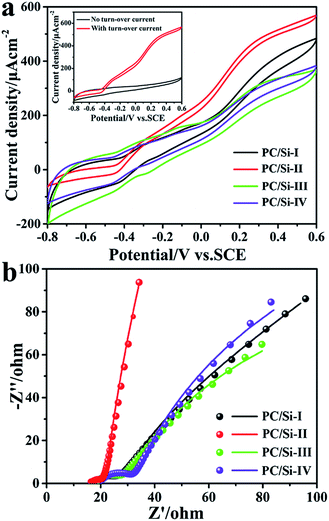 |
| Fig. 3 (a) CV curves with turnover current at the scanning rate of 1 mV s−1 of different electrode materials coated on carbon cloth measured in the anaerobic M9 buffer supplemented with a S. putrefaciens CN32 cell suspension; inset shows the CVs with the turnover current compared with non-turnover current for the PC/Si-II anode. (b) Nyquist plots of different anode materials in the anaerobic suspension of S. putrefaciens CN32. | |
Furthermore, a siliceous crust surface may provide a comfortable surface for more bacteria adhesion to benefit the contact-based direct electron transfer (DET) process via outer-membrane c-type cytochromes. Therefore, the PC/Si-II anode promotes both the shuttle-mediated electron transfer (MET) process via flavins and the DET processes and thus achieves the best bioelectrocatalysis activity. In addition, the Nyquist plots (Fig. 3b) also reveal that this PC/Si-II anode possesses a much faster interfacial charge transfer than other anodes, which is in good agreement with CV results.
The polarization and power density curves of the MFCs were measured by using various external loading resistances (1–80 kΩ). As shown in Fig. 4a and b, the open circuit voltages of all MFCs equipped with different PC/Si composite anodes show no significant difference, but the maximum power densities are quite different. The PC/Si-II anode delivers a maximum power density of 580.7 mW m−2, which is much higher than those of the PC/Si-I (403.7 mW m−2), PC/Si-III (339 mW m−2) and PC/Si-IV (289.9 mW m−2) anodes. Furthermore, the maximum power density of the PC/Si-II anode as-prepared here is about 4.5-fold higher than that of a bare carbon cloth anode (127 mW m−2), which should be attributed to its excellent hierarchically porous structure and the unique siliceous crust surface discussed above. In addition, the potential variations of electrodes versus current densities during the MFC operation were investigated to verify that the improved power density is mainly attributed to the contribution of the anode.
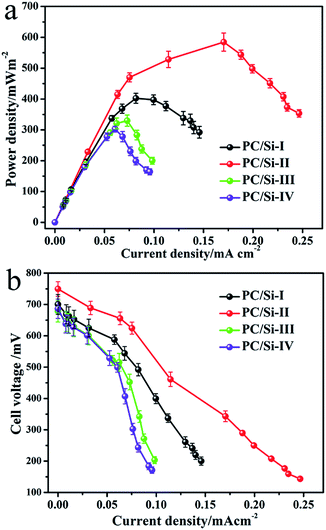 |
| Fig. 4 Power curves (a) and polarization curves (b) of different anodes during the MFC operation. | |
The current output profiles under a constant loading resistance of 1.5 kΩ were measured in a batch-type dual-chamber MFC under the same conditions to explore the electricity yields. As shown in Fig. S3,† the PC/Si-II anode delivers a stable current density of 1.8 A m−2, and four reproducible cycles of current generation obtained in the MFC with PC/Si-II indicate the long-term stability of the PC/Si-II anode. In addition to a highly efficient EET and high electrical conductivity, the improvement in the MFC performance can be further interpreted by active biofilms and bacteria produced on large surface areas of the PC/Si composite anode. After discharge, the surface morphology of the PC/Si-II was examined and the images (Fig. 5d and S4†) show that the cell density is quite high and cells adhere to each other to form a biofilm on the PC/Si composite surface due to its good biocompatibility. Interestingly, the biofilm not only firmly covers almost the entire surface of the PC/Si composite, but it also lives in the pores by adhering on the pore inside surface. In addition, this would load a much larger number of biocatalysts and facilitate the cell-to-cell electron communication for fast electron transport on the PC/Si composite anode resulting in simultaneously boosting the bio- and electro-catalytic processes for a high power MFC.
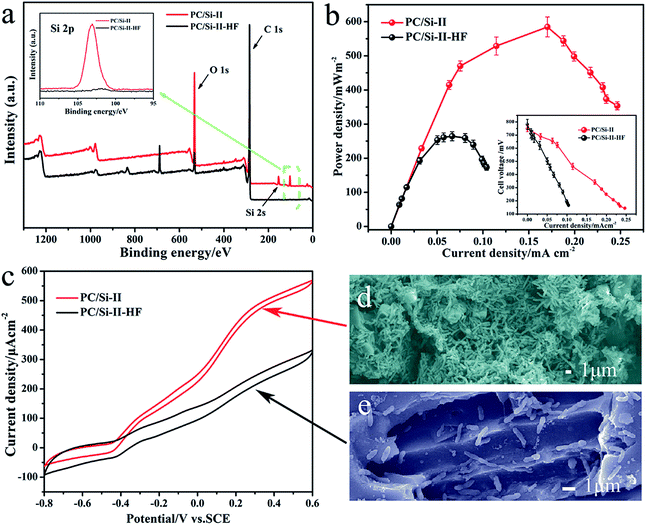 |
| Fig. 5 (a) XPS wide-scanned (inset shows the Si 2p core-level spectra), (b) output power density plots (inset is the polarization curve), (c) CV with turnover current at the scanning rate of 1 mV s−1 of the porous carbon–silica composite before and after removing silicon from the surface. FESEM images of S. putrefaciens CN32 cells grown on anodes of PC/Si-II (d) and PC/Si-II–HF (e) after discharge in a dual-chamber MFC. | |
For a more in-depth explanation of the beneficial effects of a siliceous crust surface, the electro catalysis behavior of silica removed PC/Si-II (PC/Si-II–HF) was also investigated. For the PC/Si-II–HF, the morphology (Fig. S5a and b†) does not change much but the silicon content on the surface (Fig. S5c and d†) was reduced significantly, which is consistent with the results of XPS (Fig. 5a). The power curve shows that the maximum power density (Fig. 5b) is only 263.5 mW m−2 for the MFC PC/Si anode with the silica removed and the CV also proved a decreased catalytic current for the silica removed one (Fig. 5c). The SEM images (Fig. 5d and e) of these two anodes prove that the bacteria cells prefer to accumulate on the silica crusts rather than the silica-removed surface. To further investigate the function of the siliceous crusts in the interfacial electron transfer, the electrochemical behaviors of the PC/Si-II and PC/Si-II–HF anodes in a phosphate buffer containing 2 μM flavin mononucleotide (FMN, the self-generated electron shuttle) were recorded (Fig. S6†). This shows that the redox peaks of flavins and the charge transfer resistance are almost same before and after silica crust removal, which suggests that the presence of silica crusts promotes the biofilm formation without affecting the flavin redox reaction. According to the above discussion, the best bioelectrocatalysis performance of the PC/Si-II should be ascribed to the hierarchical porous structure as well as the silica crust surface.
Thus, a reasonable mechanism for the greatly improved MFC performance with a hierarchical porous structure and siliceous crust surface of the porous carbon–silica anode is summarized in Fig. 6. The unique porous structure and surface chemistry promote bacterial growth on the anode surface to form an electroactive biofilm, which can apparently promote the direct electron transfer from their outer membrane redox species, such as c-type cytochromes (OM c-Cyts), to the electrode.42–45 Furthermore, the large area of biofilm will accumulate flavins on the anode surface to promote the interfacial electron transfer. Considering that self-excreted electron shuttles are often found at nM or μM concentrations,46,47 the biofilm could guarantee sufficient flavins for a mediated EET. After removing siliceous crusts from the porous carbon–silica anode, the long distance for the free flavin diffusion leads to a sluggish EET from the exoelectrogen to the anode and thus a low power output. Thus, this work demonstrates that the PC/Si composite derived from distiller's grain biomass with a hierarchically porous structure and siliceous crust surface could improve the bioelectrocatalysis by a large amount of biofilm adhesion as well as an enhanced interfacial electron transfer.
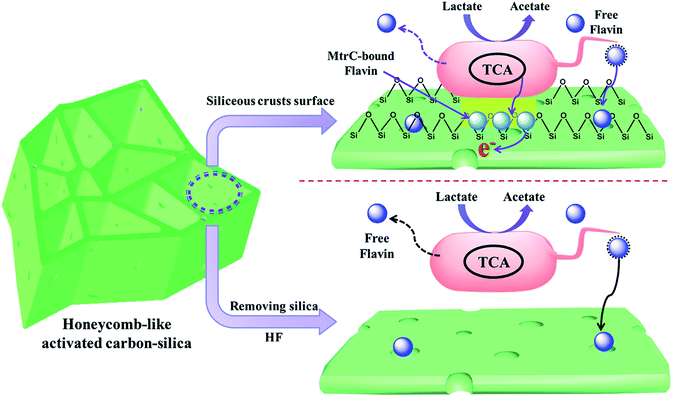 |
| Fig. 6 Schematic model illustration of a hierarchically porous structure and siliceous crust surface on the enhancement of the biofilm anode electrocatalysis in S. putrefaciens CN32 MFC. | |
4. Conclusions
In summary, a honeycomb-like hierarchical porous carbon–silica composite was derived from distiller's grains and employed as the anode for S. putrefaciens CN32 MFC for the first time. After optimization with the carbonization temperature, the PC/Si anode delivers a much higher maximum power density than carbon cloth due to the unique nanostructure and siliceous crust surface for a high bioelectrocatalysis activity. The 3D scaffold with mesopores on the wall provide a large surface for both biofilm formation and the flavin redox reaction. At the same time, the siliceous crusts greatly promote the adhesion of bacteria cells on the PC/Si surface so that a large amount of electroactive biofilm can enhance the rate of interfacial electron transfer. Furthermore, the PC/Si electrode material is quite stable in the MFC system for long-term discharging. Considering the synthesis process is facile and the cost of distiller's grains is very low, this PC/Si anode could be very promising to be applied for high performance, large scale MFCs.
Conflicts of interest
There are no conflicts to declare.
Acknowledgements
We gratefully acknowledge the financial support from the Fundamental Research Funds for the Central Universities (XDJK2015B018); Chongqing Key Laboratory for Advanced Materials and Technologies of Clean Energies and Chongqing Science and Technology Commission (cstc2017jcyjAX0199); National Program of College Students Innovation and Entrepreneurship Training (No. 201310635039).
References
- B. E. Logan, Appl. Microbiol. Biotechnol., 2010, 85, 1665–1671 CrossRef CAS PubMed.
- Y. Qiao, X.-S. Wu and C. M. Li, J. Power Sources, 2014, 266, 226–231 CrossRef CAS.
- E. Powell and G. Hill, Energy, 2010, 35, 4582–4586 CrossRef CAS.
- G. Kumar, R. G. Saratale, A. Kadier, P. Sivagurunathan, G. Zhen, S.-H. Kim and G. D. Saratale, Chemosphere, 2017, 177, 84–92 CrossRef CAS PubMed.
- F. Rezaei, T. L. Richard, R. A. Brennan and B. E. Logan, Environ. Sci. Technol., 2007, 41, 4053–4058 CrossRef CAS PubMed.
- J. Li and Z. He, Environ. Sci.: Water Res. Technol., 2015, 1, 355–362 CAS.
- A. Marone, A. Carmona-Martínez, Y. Sire, E. Meudec, J. Steyer, N. Bernet and E. Trably, Water Res., 2016, 100, 316–325 CrossRef CAS PubMed.
- D. R. Bond, D. E. Holmes, L. M. Tender and D. R. Lovley, Science, 2002, 295, 483–485 CrossRef CAS PubMed.
- A. Kadier, Y. Simayi, M. S. Kalil, P. Abdeshahian and A. A. Hamid, Renewable Energy, 2014, 71, 466–472 CrossRef CAS.
- M. Kitching, R. Butler and E. Marsili, Enzyme Microb. Technol., 2017, 96, 1–13 CrossRef CAS PubMed.
- X. Cao, X. Huang, P. Liang, K. Xiao, Y. Zhou, X. Zhang and B. E. Logan, Environ. Sci. Technol., 2009, 43, 7148–7152 CrossRef CAS PubMed.
- Q. Ping, C. Zhang, X. Chen, B. Zhang, Z. Huang and Z. He, Environ. Sci. Technol., 2014, 48, 13010–13019 CrossRef CAS PubMed.
- Y. Qiao, X.-S. Wu, C.-X. Ma, H. He and C. M. Li, RSC Adv., 2014, 4, 21788–21793 RSC.
- K. Katuri, M. L. Ferrer, M. C. Gutiérrez, R. Jiménez, F. del Monte and D. Leech, Energy Environ. Sci., 2011, 4, 4201–4210 CAS.
- X. Xie, L. Hu, M. Pasta, G. F. Wells, D. Kong, C. S. Criddle and Y. Cui, Nano Lett., 2010, 11, 291–296 CrossRef PubMed.
- X. Xie, M. Ye, L. Hu, N. Liu, J. R. McDonough, W. Chen, H. Alshareef, C. S. Criddle and Y. Cui, Energy Environ. Sci., 2012, 5, 5265–5270 CAS.
- Y. Zhao, K. Watanabe, R. Nakamura, S. Mori, H. Liu, K. Ishii and K. Hashimoto, Chem.–Eur. J., 2010, 16, 4982–4985 CrossRef CAS PubMed.
- M.-M. Titirici and M. Antonietti, Chem. Soc. Rev., 2010, 39, 103–116 RSC.
- R. J. White, V. Budarin, R. Luque, J. H. Clark and D. J. Macquarrie, Chem. Soc. Rev., 2009, 38, 3401–3418 RSC.
- P. Cheng, S. Gao, P. Zang, X. Yang, Y. Bai, H. Xu, Z. Liu and Z. Lei, Carbon, 2015, 93, 315–324 CrossRef CAS.
- Z. Li, W. Lv, C. Zhang, B. Li, F. Kang and Q.-H. Yang, Carbon, 2015, 92, 11–14 CrossRef CAS.
- C. Long, L. Jiang, X. Wu, Y. Jiang, D. Yang, C. Wang, T. Wei and Z. Fan, Carbon, 2015, 93, 412–420 CrossRef CAS.
- M. Sevilla and A. B. Fuertes, Energy Environ. Sci., 2011, 4, 1765–1771 CAS.
- M. Sevilla, A. Fuertes and R. Mokaya, Energy Environ. Sci., 2011, 4, 1400–1410 CAS.
- S. Chen, G. He, X. Hu, M. Xie, S. Wang, D. Zeng, H. Hou and U. Schröder, Chemsuschem, 2012, 5, 1059 CrossRef CAS PubMed.
- S. Chen, Q. Liu, G. He, Y. Zhou, M. Hanif, X. Peng, S. Wang and H. Hou, J. Mater. Chem., 2012, 22, 18609–18613 RSC.
- Y. Yuan, S. Zhou, Y. Liu and J. Tang, Environ. Sci. Technol., 2013, 47, 14525–14532 CrossRef CAS PubMed.
- H. Zhu, H. Wang, Y. Li, W. Bao, Z. Fang, C. Preston, O. Vaaland, Z. Ren and L. Hu, Nano Energy, 2014, 10, 268–276 CrossRef CAS.
- L. Zou, Y. Qiao, X.-S. Wu and C. M. Li, J. Power Sources, 2016, 328, 143–150 CrossRef CAS.
- Y. Qiao, G.-Y. Wen, X.-S. Wu and L. Zou, RSC Adv., 2015, 5, 58921–58927 RSC.
- A. Bharadwaj, Y. Wang, S. Sridhar and V. Arunachalam, Curr. Sci., 2004, 87, 981–986 Search PubMed.
- K. Kenes, O. Yerdos, M. Zulkhair and D. Yerlan, J. Non-Cryst. Solids, 2012, 358, 2964–2969 CrossRef CAS.
- J. Binner and Y. Zhang, J. Mater. Sci. Lett., 2001, 20, 123–126 CrossRef CAS.
- J. Gao, J. Y. Yu, L. Zhou, J. Muhammad, X. L. Dong, Y. N. Wang, H. T. Yu, X. Quan, S. J. Li and Y. Jung, Nano Res., 2017, 10, 2644–2656 CrossRef CAS.
- S. Rehman, S. Guo and Y. Hou, Adv. Mater., 2016, 28, 3166 CrossRef CAS PubMed.
- X. G. Chen, S. S. Lv, P. P. Zhang, L. Zhang and Y. Ye, J. Therm. Anal. Calorim., 2011, 104, 1055–1062 CrossRef CAS.
- Y. Chen, Y.-L. Li, G.-T. Zhou, H. Li, Y.-T. Lin, X. Xiao and F.-P. Wang, Sci. Rep., 2014, 4, 5696 CrossRef CAS PubMed.
- M. Colilla, M. Martínez-Carmona, S. Sánchez-Salcedo, L. Ruiz-Gonzales, J. M. Gonzalez-Calbet and M. Vallet-Regi, J. Mater. Chem. B, 2014, 2, 5639–5651 RSC.
- M. Katsikogianni and Y. F. Missirlis, Eur. Cells Mater., 2004, 8, 37 CrossRef CAS PubMed.
- T. Kinnari, J. B. Esteban, E. N. Zamora, R. Fernandez-Roblas, A. Nieto, J. Doadrio, A. Lopez-Noriega, E. Ruiz-Hernandez, D. Arcos and M. Vallet-Regi, J. Biomed. Mater. Res., Part A, 2010, 89, 215–223 Search PubMed.
- L. Zou, Y. Qiao, Z.-Y. Wu, X.-S. Wu, J.-L. Xie, S.-H. Yu, J. Guo and C. M. Li, Adv. Energy Mater., 2016, 6, 1501535 CrossRef.
- V. Flexer, J. Chen, B. C. Donose, P. Sherrell, G. G. Wallace and J. Keller, Energy Environ. Sci., 2013, 6, 1291–1298 CAS.
- X. W. Liu, J. J. Chen, Y. X. Huang, X. F. Sun, G. P. Sheng, D. B. Li, L. Xiong, Y. Y. Zhang, F. Zhao and H. Q. Yu, Sci. Rep., 2014, 4, 3732 CrossRef PubMed.
- H. Wang, G. Wang, Y. Ling, F. Qian, Y. Song, X. Lu, S. Chen, Y. Tong and Y. Li, Nanoscale, 2013, 5, 10283 RSC.
- Y. Yang, M. Xu, J. Guo and G. Sun, Process Biochem., 2012, 47, 1707–1714 CrossRef CAS.
- C. I. Torres, A. K. Marcus, H. S. Lee, P. Parameswaran, R. Krajmalnikbrown and B. E. Rittmann, FEMS Microbiol. Rev., 2010, 34, 3–17 CrossRef CAS PubMed.
- E. Marsili, D. B. Baron, I. D. Shikhare, D. Coursolle, J. A. Gralnick and D. R. Bond, Proc. Natl. Acad. Sci. U. S. A., 2008, 105, 3968–3973 CrossRef CAS PubMed.
Footnote |
† Electronic supplementary information (ESI) available: EDS mapping, average pore diameter, constant resistance discharge plots, SEM and EDS after remove the silicon. See DOI: 10.1039/c7se00560a |
|
This journal is © The Royal Society of Chemistry 2018 |
Click here to see how this site uses Cookies. View our privacy policy here.