Few-layered MoSe2 nanosheets as an efficient peroxidase nanozyme for highly sensitive colorimetric detection of H2O2 and xanthine†
Received
11th September 2017
, Accepted 19th November 2017
First published on 20th November 2017
Abstract
Molybdenum-containing and selenium-containing enzymes are generally prevalent in the biosphere, and the active sites of these involve molybdenum and selenium respectively. Herein, we for the first time demonstrated that few-layered molybdenum selenide (MoSe2) nanosheets possess intrinsic peroxidase-like activity. The few-layered MoSe2 nanosheets were prepared by a simple liquid exfoliation method. The catalytic process of the MoSe2 nanosheets accords with the Michaelis–Menten behavior and they have a higher affinity to both TMB and H2O2 compared to HRP. In addition, we established that the MoSe2 nanosheets catalyze the oxidation of TMB by promoting the electron transfer process from TMB to H2O2. More importantly, we applied the MoSe2 nanosheets to the field of biological detection by developing a highly sensitive and selective colorimetric detection of H2O2 and xanthine concentration. With these advantages, the few-layered MoSe2 nanosheets have critical potential in the diagnostics and biomedical fields.
Introduction
Nanozymes are artificial enzymes with the characteristics of inorganic nanomaterials.1 Since the first report that Fe3O4 nanoparticles possess an intrinsic peroxidase activity,2 an emerging class of nanomaterials such as metal oxides,2,3 metal-based nanomaterials,4 carbon-based nanomaterials,5,6 transition metal chalcogenides7 and others8 has been exploited as nanozymes. Nanozymes are equipped with numerous advantages such as high stability, low cost, high versatility and durability to harsh environments,9 making them potential alternatives for natural enzymes in various fields including the food industry, agriculture, biosensors and environmental treatment.10,11
Since the discovery of graphene was reported in 2004,12 increasing attention has been paid to 2D materials and their various potential applications.13–17 Transition metal dichalcogenides (TMDs),18 as a newly emerging class of 2D materials, possess abundant active edges and large surface areas, as well as unique electrical, chemical, mechanical and thermal properties.19 On the basis of these characteristics, TMDs harbor the potential to serve as nanozymes and have been applied to biological detection such as glucose detection.20–22 Moreover, xanthine is a kind of metabolite intermediate of purine nucleotides and deoxynucleotides in animals.23 An extremely abnormal level of xanthine will give rise to gout and hyperuricaemia.24 Hence, the use of TMDs as a nanozyme in xanthine detection is quite significant and indispensable.
As we know, molybdenum-containing enzymes and selenoenzymes have considerable universality around the biosphere. For almost all molybdenum-containing enzymes including dimethylsulfoxide (DMSO) reductase, xanthine oxidase, sulfite oxidase and aldehyde ferredoxin oxidoreductase (AOR),25 the catalytic site is the molybdenum cofactor (Moco) in different variants.26 Moco plays an important role in the transfer of an oxygen atom which ultimately is derived from or incorporated into water to or from a substrate.25 In addition, there are more than 50 selenoprotein families that consist of selenoenzymes including glutathione peroxidases, thioredoxin reductases and iodothyronine deiodinases.27,28 Their structure apparently ensures that the functions of most selenoproteins should be involved in redox-related reactions and selenocysteine (Sec) is an active-site residue essential for catalytic activity.28 Motivated by the critical importance of molybdenum and selenium, we propose that molybdenum selenide (MoSe2) nanosheets are likely to possess significant potential to be enzyme mimics.
In this contribution, we report few-layered MoSe2 nanosheets that were obtained via a liquid exfoliation method for their ability to catalyze peroxidative reactions in aqueous media.29 In the presence of H2O2, MoSe2 nanosheets can rapidly catalyze the oxidation of peroxidase substrate TMB to form blue oxTMB, and show peroxidase mimetic activity. The kinetics investigations show that the catalytic process follows Michaelis–Menten behavior, and their kinetic parameters are comparable to HRP. Furthermore, the catalytic pathways of peroxidase-like activity were also studied to clarify the catalytic mechanisms. Based on such characteristic activity of MoSe2 nanosheets, and given the selective catalytic oxidation of xanthine to produce H2O2 with xanthine oxidase, a colorimetric method to detect H2O2 and subsequently xanthine was developed. Therefore, these results demonstrated that this assay is sensitive and accurate.
Experimental section
Materials preparation
MoSe2 powders were purchased from Alfa Aesar. 2,2′-Azino-bis(3-ethylbenzthiazoline-6-sulfonic acid) (ABTS) and o-phenylenediamine (OPD) were bought from Sigma-Aldrich. 3,3′,5,5′-Tetramethylbenzidine (TMB), NaOH, xanthine and xanthine oxidase were purchased from Aladdin Industrial Corporation. Anhydrous ethanol, acetic acid, acetic acid sodium salt, and hydrogen peroxide (30%) were bought from Guangzhou Chemical Reagent Factory. Terephthalic acid (TA) was purchased from Tokyo Chemical Industry Co. Ltd. Milli-Q water (18.2 MΩ cm) was used for all solution preparations.
The few-layered MoSe2 nanosheets were prepared from commercial MoSe2 flakes via a simple liquid exfoliation method. Briefly, commercial MoSe2 powders (2 g) were dissolved in a 45 vol% ethanol/water mixture (200 mL) and ultrasonicated for 8 h at 80% amplitude at 10 °C. Then the solution was centrifuged two times at 5000 rpm for 5 min and the supernatant was obtained by decantation. Suction filtration was carried out to remove ethanol and the filtered residue was redispersed into DI water by bath sonication for 10 min. This was followed by centrifugation at 3000 rpm for 3 min, and the supernatant was collected as the few-layered MoSe2 nanosheet solution for further characterization.
Material characterization
X-ray powder diffraction (XRD) was performed using a Rigaku D/Max-IIIA X-ray diffractometer with Cu Kα radiation (λ = 1.54056 Å, 40 kV, 20 mA) at a scanning rate of 2° s−1. The ultraviolet-visible (UV-vis) absorption spectra of the samples were obtained using a UV 3150 spectrophotometer (Shimadzu, Japan). Transmission electron microscopy (TEM) and high-resolution TEM (HRTEM) images were acquired by using an FEI Tecnai G2 F30 transition electron microscope equipped with a field-emission gun. The Raman spectra were collected by a Renishaw inVia spectrometer using a 514 nm laser for excitation. The thickness profile was acquired on a Bruker Multimode 8 atomic force microscope (AFM). Fluorescence spectroscopy was performed on a Hitachi F-4500 fluorescence spectrophotometer.
Peroxidase-like activity assay
Experiments were carried out at room temperature in a 1 mL tube. 50 μL of 0.15 mg mL−1 MoSe2 nanosheets and 100 μL of 1 M H2O2 were added into 750 μL of 200 mM acetate buffer (pH 2.5–7). Immediately after this 100 μL of 10 mM TMB was added, and the color of the reactions was observed.
The steady-state kinetic analysis of MoSe2 nanosheets with H2O2 and TMB as the substrates was performed by varying the concentrations of TMB at a fixed H2O2 initial concentration of 1 M and vice versa at a fixed TMB initial concentration of 10 mM in acetate buffer (200 mM, pH 3.5). The apparent kinetic parameters were calculated based on the Michaelis–Menten equation: v0 = Vmax × [S]/(Km + [S]), where v0 is the initial velocity, Vmax is the maximal reaction velocity, [S] is the concentration of the substrate and Km is the Michaelis constant.
Detection of hydroxyl radicals
Terephthalic acid (TA) was used as a fluorescent probe for the tracking of ˙OH and the generation of 2-hydroxy terephthalic acid (TAOH) emitted a unique fluorescence at around 430 nm. 0.2 mL of 0.15 mg mL−1 MoSe2 nanosheets, 0.4 mL of 1 M H2O2 and 0.4 mL of 25 mM TA were added into 3.0 mL of acetate buffer (pH 5.5). After 12 h of incubation in the dark at room temperature, fluorescence measurement was observed.
Cytochrome C electron transfer experiment
Cytochrome C (Cyt C) was used as an active reactant in the electron transfer process to understand the electron transfer mediator role of the MoSe2 nanosheets. 40 μL of 0.15 mg mL−1 MoSe2 nanosheets and 25 μL of 0.4 mM Cyt C were added into 120 mL acetate buffer (pH 3.5). Following 18 h of incubation in the dark at room temperature, the reaction solution was centrifuged at 8000 rpm for 5 min and then the supernatant was measured by using a UV-vis absorbance spectrometer.
H2O2 and xanthine detection
H2O2 detection was carried out at room temperature in a 1 mL tube. 50 μL of 0.15 mg mL−1 MoSe2 nanosheets and 100 μL of H2O2 of different concentrations were added into 750 μL of 200 mM acetate buffer (pH 3.5). Immediately after this 100 μL of 10 mM TMB was added, and the color of the reactions was observed.
Xanthine detection was carried out at room temperature. First, 10 μL of 0.5 U mL−1 xanthine oxidase aqueous solution and 40 μL xanthine with different concentrations were added into 50 μL of 200 mM acetate buffer (pH 7.0). Following 20 min of incubation, 750 μL of 200 mM acetate buffer (pH 3.5), 50 μL of 0.15 mg mL−1 MoSe2 nanosheets and 100 μL of 10 mM TMB were successively added to the xanthine reaction solution and the color of the reactions was observed.
Results and discussion
The crystal structure of the as-exfoliated MoSe2 was studied by XRD, as shown in Fig. 1A. The XRD patterns of the bulk powders and the as-exfoliated MoSe2 exhibit a major peak at a 2θ value of 13.6 corresponding to the (002) plane of crystalline 2H-MoSe2. After exfoliation, the (002), (004), (006) and (008) peaks were still present while the others disappeared, indicating the potent exfoliation of MoSe2.
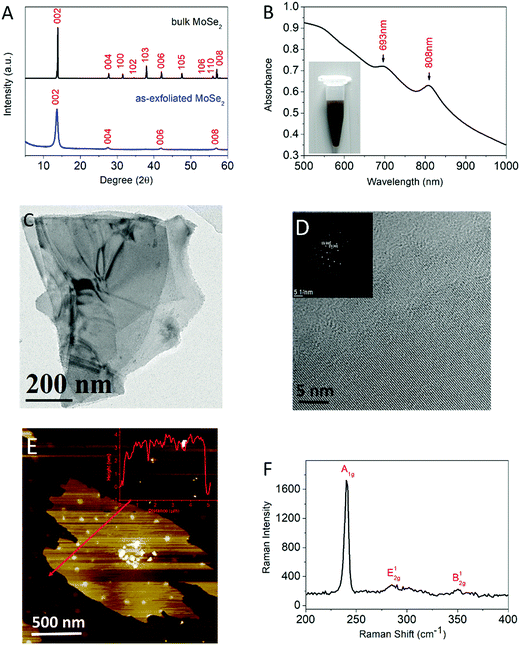 |
| Fig. 1 (A) XRD spectra of bulk MoSe2 and the corresponding exfoliated MoSe2 nanosheets prepared by liquid exfoliation. (B) UV-vis spectra and (inset) a photograph of MoSe2 nanosheets dispersed in water. (C) TEM image, (D) high resolution TEM image and the (inset) corresponding SAED pattern, (E) AFM topographic image and (F) Raman spectra of MoSe2 nanosheets. | |
As illustrated in Fig. 1B, the UV-vis spectra of the as-exfoliated MoSe2 exhibit typical characteristic peaks at 808 and 693 nm which originate from the energy split of the valence-band and spin-orbital coupling, indicating the generation of 2H-phase few-layered MoSe2 nanosheets which correspond to the XRD patterns.30 The inset of Fig. 1B shows the photograph of the dispersion of MoSe2 nanosheets stabilized in water.
The microscopic features of the as-exfoliated MoSe2 were first studied by TEM. As exhibited in Fig. 1C and Fig. S1 (ESI†), the morphology of the nanomaterials presents as two-dimensional thin sheets. Extremely thick nanosheets were rarely observed, indicating that the liquid-exfoliation process was highly efficient for obtaining few-layered sheets. In the HRTEM image (Fig. 1D), individual layer edges can be observed near the periphery of these nanosheets. The inset of Fig. 1D shows the corresponding SAED pattern which revealed the high crystallinity of the MoSe2 nanosheets and the crystal planes of (100) and (110).
The Raman spectrum is illustrated in Fig. 1F. Typical peaks of MoSe2 nanosheets at 240.2 cm−1, 285.2 cm−1 and 350.4 cm−1 were observed, which corresponded to the out-of-plane A1g mode, in-plane E12g mode and B12g mode.31 The red shift of the A1g mode by approximately 2 cm−1 compared to the bulk MoSe2, the blue shift of the E12g mode, and the coexistence of all three modes indicate the formation of few-layered MoSe2 nanosheets.31,32
The thicknesses of the as-exfoliated MoSe2 were monitored through AFM examination. The AFM image of MoSe2 (Fig. 1E and Fig. S1, ESI†) exhibits thin polydispersed wrinkled nanosheets corresponding to few-layered nanosheets given that the thickness of a MoSe2 monolayer is about 0.65 nm. All the aforementioned results confirmed that the few-layered MoSe2 nanosheets had been produced.
Kinetics investigations of peroxidase-like catalysis activities of the few-layered MoSe2 nanosheets
In the first set of experiments, the intrinsic peroxidase catalytic activity of the MoSe2 nanosheets was first investigated. As the result shows in Fig. 2A, when the MoSe2 nanosheets, H2O2 and TMB coexisted in the reaction solution, the absorbance at 652 nm increased rapidly with time. In the absence of H2O2 or MoSe2 nanosheets, the increase in absorbance was negligible, revealing that the MoSe2 nanosheets actually possess intrinsic peroxidase catalytic activity, facilitating the oxidation of TMB in the presence of H2O2. Under the same conditions, 2,2′-azino-bis(3-ethylbenzthiazoline-6-sulfonic acid) (ABTS) and o-phenylenediamine (OPD) were adopted as the substrates for the catalytic experiment. However, only the former shows an obvious color change, indicating that the MoSe2 nanosheets possess substrate specificity33 (Fig. S2, ESI†). Given the redox-related selenoproteins, a hypothesis was proposed that to some degree selenium contributes to the peroxidase-like activity. The inset of Fig. 2A exhibits the color reaction aforementioned, showing that TMB was oxidized to blue oxTMB. Similar to HRP, the catalytic activities of the MoSe2 nanosheets show pH and H2O2 concentration dependence.34 Different buffer types and pH values as well as sizes of the MoSe2 nanosheets lead to different catalytic velocities. As can be seen in Fig. 2B and Fig. S3 (ESI†), the MoSe2 nanosheets show optimal activity at around pH 3–3.5, which is very close to the value for HRP.2 The acetate buffer and MES buffer can maintain the maximum catalytic ability of the MoSe2 nanosheets. Thus, acetate buffer at pH 3.5 was adopted as the standard condition for subsequent analysis of MoSe2 nanosheet activity.
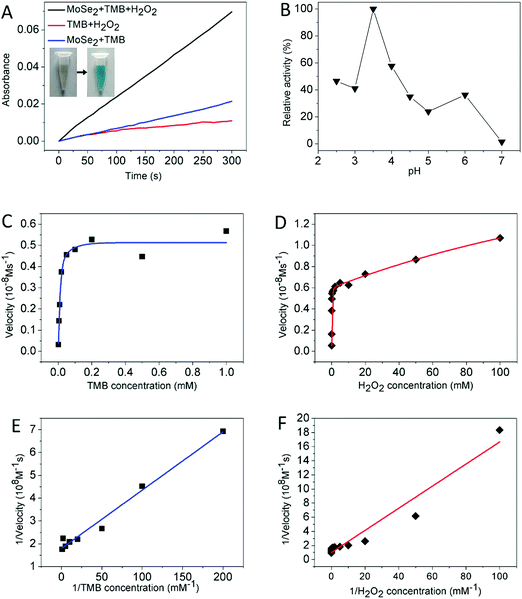 |
| Fig. 2 (A) Time-dependent absorbance of TMB at 652 nm in different reaction systems and (inset) photographs of oxidation of TMB by MoSe2 nanosheets in the presence of H2O2. (B) pH dependent peroxidase-like activity of MoSe2 nanosheets in acetate buffer and the maximum point was set as 100%. (C–F) Steady-state kinetic assay of MoSe2 nanosheets. Michaelis–Menten curve fit for (C) variation of TMB concentration and keeping H2O2 concentration constant and (D) variation of H2O2 concentration and maintaining the TMB concentration constant and the corresponding Lineweaver–Burk plots of MoSe2 nanosheets with (E) TMB as a substrate and (F) H2O2 as a substrate. | |
For a better understanding of the MoSe2 nanosheet catalytic activities, a steady-state kinetics analysis was performed to acquire the kinetic parameters. Within the suitable range of TMB or H2O2 and at the same time fixing the other concentration, the Michaelis–Menten curves were obtained as shown in Fig. 2C and D. And the kinetic parameters including the Michaelis–Menten constant (Km) and the maximal velocity (Vmax) were obtained according to the Lineweaver–Burk plot (Fig. 2E and F). The apparent Km value is an index that measures the affinity of the substrates to enzymes and the lower the Km value is, the higher the affinity the substrate bears. Table 1 lists the Km and Vmax values for the MoSe2 nanosheets and HRP. For both TMB and H2O2 as the substrates, the apparent Km values of HRP are 30 times and 22.8 times higher than those of the MoSe2 nanosheets respectively, suggesting that the MoSe2 nanosheets bear a higher affinity for both TMB and H2O2 than HRP. The reasons will be summarized in the following two contributions. Firstly, the plentiful active edges and defects on the surface as well as large the surface areas of the 2D materials provide the MoSe2 nanosheets with numerous bonding points to TMB and H2O2.35 Secondly, taking into consideration the functions of molybdenum in Moco and selenium in selenocysteine, an assumption was proposed that molybdenum and selenium are apt to absorb the substrate which needs further research in the future.
Table 1 Comparison of the kinetic parameters of MoSe2 nanosheets and HRP. Km is the Michaelis constant, Vmax is the maximal velocity
Catalyst |
Substrate |
K
m (mM) |
V
max (10−8 M s−1) |
Ref. |
HPR |
TMB |
0.434 |
10.0 |
2
|
H2O2 |
3.70 |
8.71 |
|
MoSe2 nanosheets |
TMB |
0.014 |
0.56 |
This work |
H2O2 |
0.155 |
0.99 |
Reaction mechanism
In order to figure out the mechanism with which MoSe2 nanosheets catalyze the oxidation of TMB in the presence of H2O2, two experiments were designed.36 On the one hand, the hydroxyl radicals (˙OH) generated by H2O2 were detected to find out whether the ˙OH radicals oxidise TMB. Terephthalic acid (TA) was used as a fluorescence probe for the tracking of ˙OH and the generation of 2-hydroxy terephthalic acid (TAOH) emitted a unique fluorescence at around 430 nm. As illustrated in Fig. 3A, after 12 h of incubation in the dark, the fluorescence at 430 nm decreased with the increased concentration of MoSe2 nanosheets, indicating the ˙OH consumption due to the presence of MoSe2 nanosheets, excluding that the catalytic action was caused by ˙OH. On the other hand, reduced cytochrome C (Cyt C) was used as an active reactant in the electron transfer process to understand the electron transfer mediator role of the MoSe2 nanosheets. After 18 h of incubation in dark in the presence of MoSe2 nanosheets, the absorbance of Cyt C at typically 520 and 550 nm disappeared while the evolution of a new peak at 530 nm appeared (Fig. 3B). The change from the reduced state to the oxidized state of Cyt C, reveals the electron transfer from Cyt C to the MoSe2 nanosheets.
In addition, considering the function of Moco that catalyzes the transfer of an oxygen atom and redox-related selenoproteins with selenocysteine as the active-site residue, molybdenum and selenium are likely to facilitate electron transfer and redox-related reactions. The mechanism of the MoSe2 nanosheets acting as a peroxidase was proposed as follows. First, TMB is absorbed to the surface of the MoSe2 nanosheets and transfers lone-pair electrons in the amino groups to the MoSe2 nanosheets. Because of the large specific surface area of the 2D MoSe2 nanosheets, plenty of active sites are available for the transfer process, leading to the oxidation of TMB and increase of the MoSe2 nanosheet electron density. Then, the electron-rich MoSe2 nanosheets transfer their electrons to nearby H2O2 and reduce H2O2 to water. The electron concentration gradient accelerates the electron transfer from the MoSe2 nanosheets to H2O2 and consequently the decrease in electron concentration of the MoSe2 nanosheets promotes the electron transfer from TMB to the MoSe2 nanosheets. As a result, TMB is oxidized to blue oxTMB and H2O2 is reduced to water. In short, the MoSe2 nanosheets catalyze the process which is similar to the case of graphene oxide,5 that is, MoSe2 nanosheets promote the electron transfer from TMB to H2O2, producing blue oxTMB and water.
Colorimetric detection of H2O2 and xanthine
On the basis of the peroxidase catalytic activity of the MoSe2 nanosheets, a colorimetric detection method for H2O2 and xanthine concentration was designed and a schematic of the colorimetric methods was proposed as illustrated in Fig. 4A. As mentioned above, the catalytic activities of MoSe2 nanosheets show H2O2 concentration dependence, which shows the potential for MoSe2 nanosheets to detect H2O2 concentration. Fig. 4B shows the increased time-course absorbance at 652 nm with different H2O2 concentrations, suggesting that the oxidation rate is in proportion to the H2O2 concentration. The corresponding H2O2 concentration–response curve is shown in Fig. 4C. A linear relationship was obtained in the inset of Fig. 4C and the H2O2 concentration was in the range of 0.01 mM to 0.16 mM with a detection limit of 0.408 μM. Given the H2O2 production of the xanthine oxidation, a colorimetric detection method for xanthine was established consequently. The same as the detection of H2O2, the increased time-course absorbance at 652 nm is in proportion to the xanthine concentration as shown in Fig. 4D and E. The inset of Fig. 4E illustrates the linear relationship and the xanthine concentration is in the range of 0.01 mM to 0.32 mM with a detection limit of 1.964 μM. This method based on MoSe2 nanosheets possessed a larger linear range compared to other nanomaterials37,38 (Table S1, ESI†) and showed a high selectivity for xanthine (Fig. S4, ESI†). In addition, a serum sample was detected using this method with the result 0.215 ± 0.009 mM, indicating the practicability of this method.
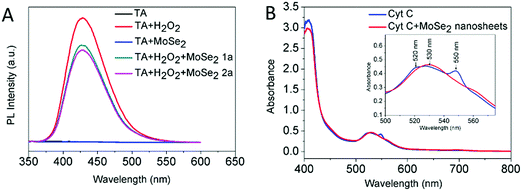 |
| Fig. 3 (A) Fluorescence at 430 nm of 2-hydroxy terephthalic acid (TAOH), and the generation of terephthalic acid (TA) tracking ˙OH. (B) Electron transfer experiment from cytochrome C (Cyt C) to MoSe2 nanosheets. | |
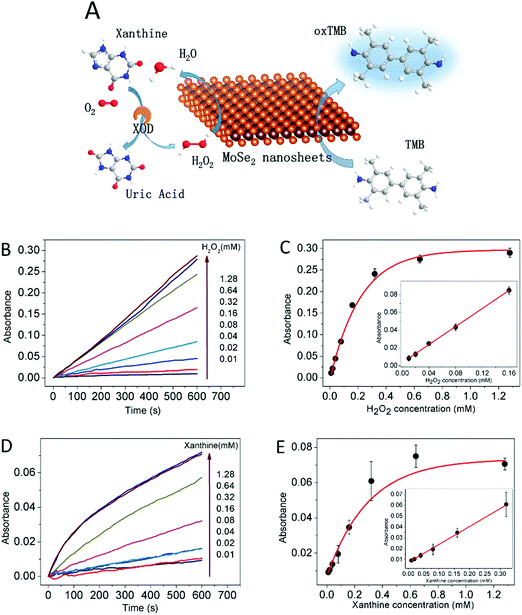 |
| Fig. 4 (A) Schematic illustration of colorimetric detection of H2O2 and xanthine. (B and D) Time-dependent absorbance of TMB at 652 nm in the presence of increased H2O2 or xanthine concentration. (C and E) The corresponding H2O2 or xanthine concentration–response curve using MoSe2 nanosheets as a nanozyme and (inset) the linear calibration plot for H2O2 or xanthine. | |
Conclusion
In summary, few-layered MoSe2 nanosheets were prepared via a liquid exfoliation method and these MoSe2 nanosheets exhibit a high peroxidase-like activity in that they can oxidize TMB in the presence of H2O2, producing a color reaction. The catalytic process complies with the Michaelis–Menten behavior and the steady-state kinetics analysis indicates that MoSe2 nanosheets have a higher affinity for both TMB and H2O2 compared to HRP. The catalytic mechanism assays illustrate that the MoSe2 nanosheets promote the electron transfer process from TMB to H2O2. On the basis of the color reaction, a detection method with high sensitivity and selectivity for H2O2 and xanthine concentration was designed. The detection of H2O2 and xanthine was in a linear range from 0.01 mM to 0.16 mM and 0.01 mM to 0.32 mM respectively, opening up possibilities for few-layered MoSe2 nanosheets as peroxidase mimics in the diagnostics and biomedical fields.
Conflicts of interest
There are no conflicts to declare.
Acknowledgements
The National Basic Research Program of China (2014CB931700) and the State Key Laboratory of Optoelectronic Materials and Technologies supported this work.
References
- H. Wei and E. Wang, Chem. Soc. Rev., 2013, 42, 6060–6093 RSC.
- L. Gao, J. Zhuang, L. Nie, J. Zhang, Y. Zhang, N. Gu, T. Wang, J. Feng, D. Yang, S. Perrett and X. Yan, Nat. Nanotechnol., 2007, 2, 577–583 CrossRef CAS PubMed.
- M. Liang, K. Fan, Y. Pan, H. Jiang, F. Wang, D. Yang, D. Lu, J. Feng, J. Zhao, L. Yang and X. Yan, Anal. Chem., 2013, 85, 308–312 CrossRef CAS PubMed.
- X. Zheng, Q. Liu, C. Jing, Y. Li, D. Li, W. Luo, Y. Wen, Y. He, Q. Huang, Y. T. Long and C. Fan, Angew. Chem., 2011, 50, 11994–11998 CrossRef CAS PubMed.
- Y. Song, K. Qu, C. Zhao, J. Ren and X. Qu, Adv. Mater., 2010, 22, 2206–2210 CrossRef CAS PubMed.
- Y. Huang, C. Liu, F. Pu, Z. Liu, J. Ren and X. Qu, Chem. Commun., 2017, 53, 3082–3085 RSC.
- T. Lin, L. Zhong, L. Guo, F. Fu and G. Chen, Nanoscale, 2014, 6, 11856–11862 RSC.
- J. W. Zhang, H. T. Zhang, Z. Y. Du, X. Wang, S. H. Yu and H. L. Jiang, Chem. Commun., 2014, 50, 1092–1094 RSC.
- R. Ragg, M. N. Tahir and W. Tremel, Eur. J. Inorg. Chem., 2016, 1906–1915 CrossRef CAS.
- C. Mateo, J. M. Palomo, G. Fernandez-Lorente, J. M. Guisan and R. Fernandez-Lafuente, Enzyme Microb. Technol., 2007, 40, 1451–1463 CrossRef CAS.
- C. Charcosset, J. Chem. Technol. Biotechnol., 1998, 71, 95–110 CrossRef CAS.
- K. S. Novoselov, A. K. Geim, S. V. Morozov, D. Jiang, Y. Zhang, S. V. Dubonos, I. V. Grigorieva and A. A. Firsov, Science, 2004, 306, 666–669 CrossRef CAS PubMed.
- B. Mendoza-Sanchez and Y. Gogotsi, Adv. Mater., 2016, 28, 6104–6135 CrossRef CAS PubMed.
- D. Deng, K. S. Novoselov, Q. Fu, N. Zheng, Z. Tian and X. Bao, Nat. Nanotechnol., 2016, 11, 218–230 CrossRef CAS PubMed.
- Q. He, Z. Zeng, Z. Yin, H. Li, S. Wu, X. Huang and H. Zhang, Small, 2012, 8, 2994–2999 CrossRef CAS PubMed.
- J. D. Roy-Mayhew and I. A. Aksay, Chem. Rev., 2014, 114, 6323–6348 CrossRef CAS PubMed.
- F. Cao, E. Ju, Y. Zhang, Z. Wang, C. Liu, W. Li, Y. Huang, K. Dong, J. Ren and X. Qu, ACS Nano, 2017, 11, 4651–4659 CrossRef CAS PubMed.
- C. Tan and H. Zhang, Chem. Soc. Rev., 2015, 44, 2713–2731 RSC.
- M. Chhowalla, H. S. Shin, G. Eda, L. J. Li, K. P. Loh and H. Zhang, Nat. Chem., 2013, 5, 263–275 CrossRef PubMed.
- S. Cai, Q. Han, C. Qi, Z. Lian, X. Jia, R. Yang and C. Wang, Nanoscale, 2016, 8, 3685–3693 RSC.
- T. Lin, L. Zhong, Z. Song, L. Guo, H. Wu, Q. Guo, Y. Chen, F. Fu and G. Chen, Biosens. Bioelectron., 2014, 62, 302–307 CrossRef CAS PubMed.
- T. M. Chen, X. J. Wu, J. X. Wang and G. W. Yang, Nanoscale, 2017, 9, 11806–11813 RSC.
- F. Qiao, J. Wang, S. Ai and L. Li, Sens. Actuators, B, 2015, 216, 418–427 CrossRef CAS.
- X. Liu, W. M. Lin, X. H. Yan, X. H. Chen, J. R. Hoidal and P. Xu, J. Chromatogr. B: Anal. Technol. Biomed. Life Sci., 2003, 785, 101–114 CrossRef CAS.
- C. Kisker, H. Schindelin and D. C. Rees, Annu. Rev. Biochem., 1997, 66, 233–267 CrossRef CAS PubMed.
- R. R. Mendel, J. Biol. Chem., 2013, 288, 13165–13172 CrossRef CAS PubMed.
- V. M. Labunskyy, D. L. Hatfield and V. N. Gladyshev, Physiol. Rev., 2014, 94, 739–777 CrossRef CAS PubMed.
- J. Lu and A. Holmgren, J. Biol. Chem., 2009, 284, 723–727 CrossRef CAS PubMed.
- K. G. Zhou, N. N. Mao, H. X. Wang, Y. Peng and H. L. Zhang, Angew. Chem., 2011, 50, 10839–10842 CrossRef CAS PubMed.
- N. Dong, Y. Li, Y. Feng, S. Zhang, X. Zhang, C. Chang, J. Fan, L. Zhang and J. Wang, Sci. Rep., 2015, 5, 14646 CrossRef CAS PubMed.
- P. Tonndorf, R. Schmidt, P. Bottger, X. Zhang, J. Borner, A. Liebig, M. Albrecht, C. Kloc, O. Gordan, D. R. Zahn, S. Michaelis de Vasconcellos and R. Bratschitsch, Opt. Express, 2013, 21, 4908–4916 CrossRef CAS PubMed.
- S. K. Balasingam, J. S. Lee and Y. Jun, Dalton Trans., 2015, 44, 15491–15498 RSC.
- Z. Zhang, X. Zhang, B. Liu and J. Liu, J. Am. Chem. Soc., 2017, 139, 5412–5419 CrossRef CAS PubMed.
- J. Mu, Y. Wang, M. Zhao and L. Zhang, Chem. Commun., 2012, 48, 2540–2542 RSC.
- T. M. Chen, J. Xiao and G. W. Yang, RSC Adv., 2016, 6, 70124–70132 RSC.
- H. Su, D. D. Liu, M. Zhao, W. L. Hu, S. S. Xue, Q. Cao, X. Y. Le, L. N. Ji and Z. W. Mao, ACS Appl. Mater. Interfaces, 2015, 7, 8233–8242 CAS.
- Z. Yan, Q. Niu, M. Mou, Y. Wu, X. Liu and S. Liao, J. Nanopart. Res., 2017, 19 Search PubMed.
- M. Cui, J. Zhou, Y. Zhao and Q. Song, Sens. Actuators, B, 2017, 243, 203–210 CrossRef CAS.
Footnote |
† Electronic supplementary information (ESI) available. See DOI: 10.1039/c7tb02434g |
|
This journal is © The Royal Society of Chemistry 2018 |
Click here to see how this site uses Cookies. View our privacy policy here.