Quenched hexacene optoacoustic nanoparticles†
Received
2nd October 2017
, Accepted 10th October 2017
First published on 27th October 2017
Abstract
Optoacoustic (photoacoustic) imaging enables high-resolution optical imaging at depths well beyond optical microscopy, revolutionizing optical interrogation of tissues. Operation in the near-infrared (NIR) is nevertheless necessary to capitalize on the technology potential and reach depths of several centimeters. Using Flash NanoPrecipitation for highly-scalable single-step encapsulation of hydrophobic hexacene at self-quenching concentrations, we propose quenched fluorescence-dye nanoparticles as a potent alternative to NIR metal nanoparticles for strong optoacoustic signal generation. Comprehensive hexacene-based nanoparticle characterization was based on a 5-step approach that examined the physicochemical features (Step 1), optoacoustic signal generation (Step 2), stability (Step 3), biocompatibility (Step 4) and spectral sensitivity (Step 5). Using this characterization framework we showcase the discovery of two nanoparticle formulations, QH2-50 nm and QH2-100 nm that attain superior stability characteristics and optimal optoacoustic properties compared to gold standards commonly employed for near-infrared optoacoustics. We discuss encapsulation and self-quenching (ESQ) of organic dyes as a promising strategy to generate optimal optoacoustic particles.
1. Introduction
Optoacoustic imaging brings a revolution to optical interrogation by enabling high-resolution imaging deep inside tissues, well beyond the depths reached by optical microscopy.1 Optoacoustic signal can be generated by endogenous tissue absorbers (e.g. oxyhemoglobin, deoxyhemoglobin, melanin) or by exogenous optoacoustic reporters such as metallic nanoparticles or organic chromophores.2–4 Exogenously administered reporters contribute to an increased versatility of optoacoustic contrast and visualization ability. Compared to the visible spectrum, the lower light absorption by tissue offered in the near-infrared (NIR: 650–950 nm) has directed the use of particles with a NIR absorption spectrum, to achieve higher detection sensitivity and depths of several centimeters in vivo.5 Due to plasmon resonance, gold and other metal-based particles exhibit strong optoacoustic signal and have been prominently considered as optoacoustic contrast agents. Asymmetric gold particles (e.g. such as rods, stars, prisms etc.6–9), in particular have been broadly explored due to their strong near infra-red absorption properties for visualization deep in tissues.9–12 Gold nanorods were conjugated to vascular cell adhesion molecule 1 (VCAM-1)13 or folic acid14 to target inflammation or cancer cells respectively. Likewise, anti-αvβ3 integrin targeted Gold nanobeacons (liposomes encapsulating gold particles) have shown to enhance optoacoustic contrast of vasculature15 and aid detecting sentinel lymph nodes.16 The use of gold nanorods to track liposome delivery of therapeutic siRNA has also been demonstrated.17 Overall, the potential to utilize gold particles to visualize biological processes in tissues has been demonstrated in several other studies, including melanoma cell targeting,18 or macrophage tracking within atherosclerotic plaques.19
The use of near-infrared gold nanorods is limited however by three important phenomena. First, while nanorods may have an absorption maximum in the wavelength range of interest (680–900 nm) the absorption over the entire range is still quite high as it directly depends on the size distribution of the sample. A figure of merit for contrast sensitivity, which is required to enable background subtraction, is given by the difference in absorption between the peak and the minimum divided by the difference between the minimum and baseline within the imaging window of 680–900 nm:
| 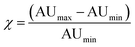 | (1) |
whereas
χ ∼ 1–3 for commercial gold nanorod systems
20–22 and
χ ∼ 20 for IndoCyanine Green,
χ ∼ 35 for the quenched hexacene nanoparticles reported herein, as will be shown below. The dramatic difference in contrast sensitivity is due to the near baseline absorbance for symmetric organic chromophores such as used here. A second limitation relates to photobleaching in an optoacoustic imaging setting. Gold nanorods or other asymmetric gold particles exposed for an extended amount of time to light at high fluence energy often lose their shape and convert to gold spheres, shifting their absorption spectrum to the visible,
23i.e. losing their near-infrared absorption advantage.
20–22 Third, metal particles accumulate in tissue for extended periods of time leading to toxicity considerations.
24–28
As optoacoustic imaging detects photoabsorbance, it is also capable of detecting organic chromophores such as organic dyes and fluorophores. Optoacoustic imaging of fluorescent aqueous-soluble organic compounds, often cyanines such as IndoCyanine Green (ICG) and other organic fluorochromes, has been demonstrated.29 ICG is an approved NIR clinical imaging agent that exhibits high absorption cross-section and biocompatibility, and is routinely employed in ophthalmology or interventional medicine to visualize vasculature and perfusion.30 As another example, Pu, et al. (2014), have reported the development of an optoacoustic imaging platform utilizing the nanoprecipitation of semiconducting polymers. The nanoparticles formed are 40–50 nm in size and stabilized by DPPC. The polymers had broad absorption spectra centered around 650–700 nm and demonstrated detection of reactive oxygen species with a relatively high resistance to photobleaching.31 Nevertheless, metal based particles exhibit much higher absorption cross-section than organic dyes and fluorochromes and can generate significantly higher optoacoustic signals. Additionally, the loss of absorbance of chromophores due to light-activated oxidation hinders the use of organic photophores in longitudinal studies, as they lose their contrast generating properties under repeated illumination.
Progress in optoacoustic imaging requires advances in the development of appropriate agents that combine the biocompatibility and spectral properties of organic molecules with the strong signal generation common to nanoparticles. An additional requirement is the scalable and fast production of such agents for disseminated use. It has been theoretically predicted, that sufficient local accumulation of fluorescent dyes could generate similar optoacoustic responses compared to metal particles assuming the same administered mass.4 Based on this observation, we considered herein a new strategy for optoacoustic agent production based on the use of quenched fluorescent molecules encapsulated in nanoparticulate form. To achieve scalability we employed Flash NanoPrecipitation (FNP) (Scheme 1),32 a novel technology that enables highly efficient encapsulation of hydrophobic organic molecules into block-copolymer-stabilized nanoparticles.33–36 FNP allows high concentration of fluorochromes within nanoparticle dimensions effectively quenching the dye and limiting the loss of absorbed energy to fluorescence photon generation. This approach can further enhance thermoelastic generation necessary for optoacoustic signal generation. FNP rapidly mixes a solvent stream containing organic active with a dissolved amphiphilic block copolymer with an antisolvent stream, such as water. The rapid drop in solvent quality causes precipitation of the active and assembly of the block copolymer. When the mixing time scale is short and the time scales for nucleation and growth are well matched, the growing organic nuclei are arrested by the adsorption of the hydrophobic block of the block copolymer to form nanoparticles ranging 30–400 nm in size with uniform size distributions. Steric hindrance of the amphiphilic block, usually polyethylene glycol (PEG), protects the particles from further aggregation. The PEG layer also resists protein adsorption, which reduces clearance and allows for longer in vivo circulation.37 This process has been successfully employed to encapsulate a wide variety of hydrophobic drugs and prodrugs, organic imaging agents, peptides, and inorganic structures (gold, silver, and upconverting nanoparticles).38–48 Overall, the FNP platform enables the production of targeted nanoparticles by using block copolymers with functional groups attached to the terminal ends of some fraction of the block copolymer hydrophilic blocks. For small molecule ligands, such as mannose and RGD peptides, the ligand can be covalently bound to the PEG chain prior to nanoparticle assembly.34,49 For larger protein and antibody targeting agents, functionalization can be accomplished after nanoparticle formation.50 The dense PEG layers on the nanoparticle surface prevents non-specific clearance by the RES.51
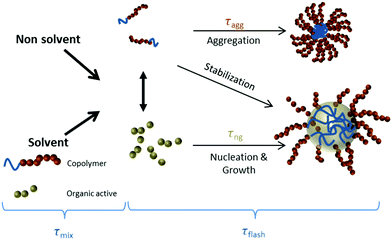 |
| Scheme 1 Nanoparticle formation by Flash NanoPrecipitation. The organic active is the long wavelength hexacene dye iBu-TSBSH5 (referred to here as LW2 or QH2). | |
FNP production and characterization was applied to deliver a set of novel near-infrared particles with particularly attractive optoacoustic imaging features. To screen and select optimal particles, we followed a 5-step approach whereby a comprehensive evaluation of optoacoustic contrast agents could be performed for optimal selection of promising candidates. Particle characterization comprised the investigation of physicochemical FNP particle characteristics (Step 1), evaluation of optoacoustic signal generation (Step 2), measurement of optical and chemical stability (Step 3), determination of biocompatibility (Step 4) and finally imaging studies (Step 5). Of particular importance herein was the development of near-infrared particles which generate strong optoacoustic responses while avoiding low photo-stability of other contrast agents alternatively employed for near-infrared optoacoustic imaging.6,52 To ensure complete encapsulation within the particle it is important to employ highly hydrophobic compounds. We selected hexacene, a highly hydrophobic near-infrared fluorescent compound53–59 with partition coefficient between water and hydrophobic phases of 1 ppb or log
P = 9. The hydrophobic characteristics ensure that the dye resides completely encapsulated in the interior of the nanoparticle and cannot partition to circulating albumin or lipid components as common with amphiphilic compounds such as ICG. The quenched-hexacene (QH) resulting particles are block copolymer, near-infrared quenched-dye nanoparticles of diameters in the sub-100 nm range and are characterized herein.
2. Results
Near-infrared absorbing, quenched hexacene dye: 6,15-bis(tri-sec-butylsilylethynyl)-2,3,10,11-bis(diisobutyldioxolanato) particles (referred to here as QH2 particles) produced by FNP were characterized as to their suitability for optoacoustic agents in a 5-steps process.
2.1. Step 1: physicochemical and optical characterization of QH2-nanoparticles
Fig. 1 summarizes optical and physicochemical characterization of the reporter probes. Fig. 1A presents a schematic of the dye loaded into polymeric nanoparticles via Flash NanoPrecipitation. The chemical structure of hexacene is shown in Fig. 1B, and its excitation/emission spectra shown in Fig. 1C. Excitation and emission peaks of QH2 fall in the optimal optical imaging window (680–900 nm), where absorbance by blood and tissue components is reduced, and light penetration in tissue is maximized. Fig. 1D presents the spectra of the hexacene dye dissolved in THF (free) versus encapsulated within the nanoparticle core. As observed, there is a 5 nm red-shift resulting from encapsulation, which is often seen for dyes in different hydrophobic environments. At high loadings of dye within the nanoparticle core, the intrinsic fluorescence (fluorescence per particle) of the nanoparticle is reduced due to quenching from Förster Resonant Energy Transfer (FRET) between dye molecules. Maximizing the fluorescence of the nanoparticle requires determining the maximum dye loading just before FRET effects dominate. By varying the dye loading within nanoparticles, but holding the nanoparticle size constant (120 nm), we determined this to be at 3.8 wt% core loading. The optimization of the QH2 loading to maximize fluorescence is described in ESI† (Fig. S1). For the purposes of optoacoustic imaging, this fluorescence maximum is not crucial as the optoacoustic signal is maximized when incident electromagnetic radiation is mostly converted to heat rather than emitted light. Fig. 1E shows the results from dynamic light scattering (DLS) for each formulation, indicating an average size of 64 nm and 95 nm for QH2-50 nm and QH2-100 nm, respectively. Fig. 1F and G present TEM images of QH2-100 nm nanoparticles. Due to the organic core, the contrast of the micrographs is relatively low, but the nanoparticles are visible as grey ‘fuzzy’ balls. The size as seen by TEM matches well with that reported by DLS (Fig. 1E). Fig. 1H compares the size, PDI width and dye core loading of both QH2-100 nm and QH2-50 nm.
 |
| Fig. 1 Characterization of the QH2-nanoparticles. (A) Schematic representation of the QH2-nanoparticles where the ‘LW2 Dye’ molecule is the same as the QH2 dye molecule. (B) Schematic of the NIR dye used (named LW2 dye or QH2 dye) for the formulation of both probes. (C) Fluorescence excitation (black) and emission (red) spectra for QH2 dissolved in THF. (D) Optical absorbance spectra for QH2 in nanoparticle cores (black) and dissolved in THF. (E) Dynamic light scattering size distribution of concentrated formulations: QH2-100 nm (black) and QH2-50 nm (red). (F) Representative TEM micrograph of QH2-100 nm. Scale bar: 400 nm. (G) High magnification TEM of dashed square region on F. Scale bar 100 nm. (H) Summary of formulation data of size, polydispersity and dye loading. | |
2.2. Step 2: optoacoustic performance of QH2-nanoparticles
Fig. 2 depicts the performance of QH2-nanoparticles as optoacoustic contrast agents using Multispectral Optoacoustic Tomography (MSOT). Different dilutions of each QH2-nanoparticle were prepared with absorbances ranging from 0.3 to 2.2 (Fig. 2A). Fig. S2 (ESI†) presents the spectrophotometric studies of these dispersions; and Fig. S3 (ESI†) presents the optoacoustic spectrum measured by MSOT for the different concentrations, that was used as an input for multispectral post-processing of optoacoustic signals. As presented in Fig. 2G, each of the diluted solutions of QH2-nanoparticles, together with the internal control (Indian ink) were prepared in a light scattering agar phantom for optoacoustic imaging. The intensity of the optoacoustic signal versus the concentration is shown in Fig. 2B, with the optoacoustic signal versus absorbance being presented in Fig. 2C. Fig. 2D shows the correlation between the normalized optoacoustic spectrum of QH2-100 nm obtained by MSOT (dashed line) and its absorption spectrum obtained by spectrophotometry (full line). The same was also performed for QH2-50 nm (Fig. 2E), showing the correlation between normalized spectra. Fig. 2H and I depict, respectively, the MSOT results for QH2-100 nm and QH2-50 nm after unmixing of the optoacoustic signal. The intensity of optoacoustic signal (pseudo bright green color) varies according to the concentration of the solution tested, with increasing green intensity correlated with increasing concentration of the sample. For both formulations tested, even at low concentrations, the optoacoustic signal can be detected, revealing the high sensitivity of MSOT for both QH2 nanoparticles in phantoms. Notably, both nanoparticles exhibit a sharp adsorption band, which improves the recognition of the particle over background by spectral unmixing methods (see Fig. S4, ESI†)
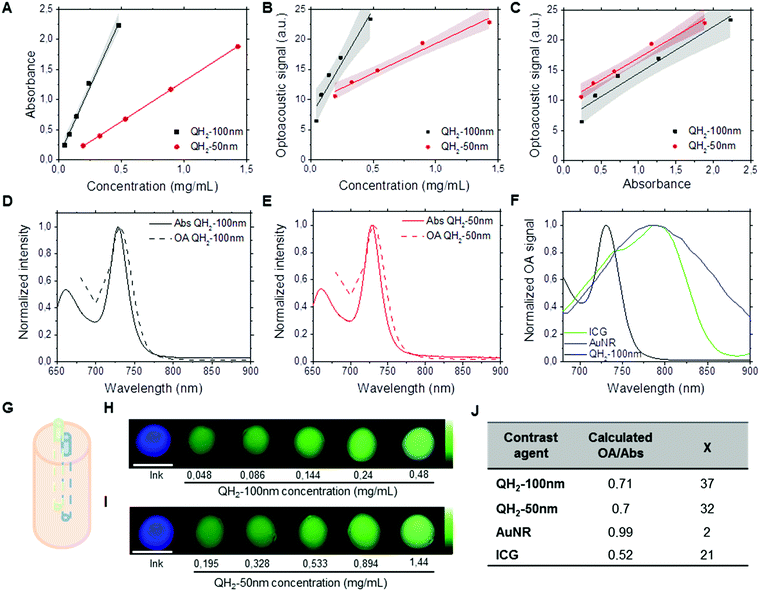 |
| Fig. 2 Performance of QH2 loaded nanoparticles as optoacoustic contrast agents. (A) Relation between absorbance and concentration for QH2-100 nm (black) and QH2-50 nm (red) with 90% confidence band. (B) Optoacoustic signal at 730 nm in an agar phantom as function of concentration for QH2-100 nm (black) and QH2-50 nm (red) with 90% confidence band. (C) Optoacoustic signal at 730 nm in an agar phantom as function of absorbance for QH2-100 nm (black) and QH2-50 nm (red) with 90% confidence band. (D and E) Normalized optoacoustic (OA) signal and absorbance (Abs) of QH2-100 nm (D) and QH2-50 nm (E). (F) Normalized optoacoustic (OA) signal obtained from QH2-100 nm (black), IndoCyanine Green (ICG, green) and PEGylated gold nanorods (AuNR, blue) at similar optical densities. (G) Example setup of a scattering agar phantom for optoacoustic measurements. (H and I) Spectral unmixing of multispectral optoacoustic signal after phantom acquisition by multispectral optoacoustic tomography for QH2-100 nm (H) and QH2-50 nm (I) at different concentrations (scale bar = 3 mm). (J) Performance of QH2-nanoparticles optoacoustic signal (OA) properties in comparison with AuNRs and ICG at similar absorbance (Abs). | |
Finally, gold nanorods (AuNr780) as well as free ICG were measured by MSOT to compare the signal generation efficiency of the QH2 nanoparticles against these two commonly used optoacoustic contrast agents. Fig. 2F shows the optoacoustic spectra recorded from solutions of ICG, AuNR and QH2-50 nm at similar absorbance (1.3). While AuNR show a characteristic broad spectra generating at least 40% of its maximum over the range of wavelengths of interest (680–900 nm), due mostly to the size distribution of the nanoparticles, ICG shows a much narrower peak with an almost complete but progressive loss of signal generation properties between 780 nm and 900 nm. On the other hand, QH2 particles display an even sharper absorbance peak, with an almost complete loss of optoacoustic signal generation properties between 730 nm and 770 nm. The values presented in Fig. 2J indicate that while ICG alone only provided around 50% of signal per unit of absorbance, both QH2 nanoparticles appear to generate approximately 70% of optoacoustic signal per unit of absorbance when compared to AuNr, considered herein being the gold standard. Calculating the χ values representing contrast sensitivity according to eqn (1) shows AuNR with a χ ∼ 2, while ICG at around 21. Both QH2-nanoparticle formulations present a χ of around 35.
2.3. Step 3 & 4: stability and biocompatibility of QH2-nanoparticles
We investigated the stability and toxicity of the QH2-nanoparticles formulations. Fig. 3A and B depict, respectively, the stability of QH2-100 nm and QH2-50 nm (OD = 1) after incubation at 4 °C in the dark for seven days. Fig. 3A and B shows the changes in absorption spectrum of each QH2-nanoparticle formulation during this period of time. The QH2-100 nm is stable overtime, without major changes in its absorption spectrum; however, the QH2-50 nm shows a decrease in the intensity of absorption over the 7 days. A photobleaching experiment was conducted by exposing samples to pulsed laser light illumination at their maximum absorbance wavelength for 30 minutes (Fig. 3C). The QH2-100 nm particle remain extremely stable, however the QH2-50 nm shows a 60% decrease in optoacoustic signal intensity. Comparatively, ICG shows a rapid loss in signal intensity to around 40% of its initial signal after 20 min, while gold nanorods exposed in the same condition prove capable of providing a constant optoacoustic signal. Fig. 3D shows the absorption spectrum of QH2-100 nm and QH2-50 nm before and after the experiment. The decrease in optoacoustic signal is tracked by the loss of light absorption. It is important to highlight that the scenario tested is unlikely to happen during in vivo MSOT imaging, since the dyes are exposed to much lower light intensity levels during an actual in vivo experiment due to natural tissue absorbance and scattering. Nevertheless, this experiment allowed the evaluation of the QH2-100 nm and QH2-50 nm particles, which exhibited improved photo-stability over ICG and gold nanorods at high concentration and fluence compared to the values reported in ref. 20–22.
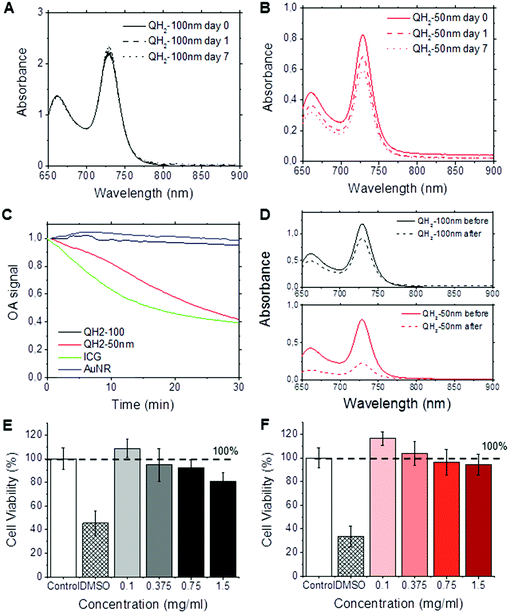 |
| Fig. 3 Stability and in vitro toxicity of QH2 nanoparticles. (A and B) Stability study of QH2-100 nm (A) and QH2-50 nm (B) in PBS stored in dark during 7 days at 4 °C. (C) Photobleaching profile acquired by optoacoustic monitoring of QH2-100 nm (black), QH2-50 nm (red), Indocyanine Green (ICG, green) and gold nanorods (AuNR, blue) during 30 minutes of prolonged pulsed laser illumination at their respective maximum absorbance wavelength. (D) Absorption spectrum of QH2-100 nm (top, black) and QH2-50 nm (bottom, red) acquired before (full line) and after (dashed line) photobleaching experiment. (E and F) In vitro toxicity study of QH2-100 nm (E) and QH2-50 nm (F) 5 days after incubation within MDA MB 231 human breast cancer cell line (n = 5). | |
Fig. 3E and F present the in vitro toxicity study of QH2-100 nm (Fig. 3E) and QH2-50 nm (Fig. 3F) after incubation with human MDA-231 mammalian tumor cells. The results demonstrate the biocompatibility of both QH2-100 nm and QH2-50 nm. Neither QH2-nanoparticles caused toxicity up to 1.5 mg mL−1. It is possible that the nanoparticles may have increased toxicity against normal cells such as NIH/3T3.
2.4. Step 5: sensitivity of QH2-nanoparticles – ex vivo proof-of-concept
A proof-of-concept ex vivo study demonstrates the applicability of QH2-nanoparticles for optoacoustic imaging. The five different dilutions of QH2-nanoparticles, along with Indian Ink as a control, were injected subcutaneously into the backs of mice post-mortem. Fig. 4A and B are color photographs with a schematic representation of each subcutaneous (s.c.) injection (left panel) location and a comparison between planar epi-fluorescent imaging (middle panel) and planar MSOT imaging (right panel) for QH2-100 nm (Fig. 4A) and QH2-50 nm (Fig. 4B). Epi-fluorescent imaging on Fig. 4A and B confirm the ex vivo MSOT results. MSOT can resolve the particles even in the lower concentration solutions (s.c. no. 5). However epi-illumination imaging cannot resolve the QH2-nanoparticles in the lower concentrations, likely due to partial fluorochrome quenching. This finding confirms the significant quenching achieved within the nanoparticles leading to higher MSOT sensitivity compared to fluorescence epi-illumination imaging. Naturally, the major advantage of MSOT over epi-fluorescent techniques is its capability for depth imaging, as shown in Fig. 4C and D. In addition to the molecular information, MSOT provides structural and anatomical information. For example, in the plan view, the internal organs, such as kidney, spleen, spinal cord can be identified. Fig. 4C and D depicts 2D coronal plan of subcutaneous injection of QH2-100 nm (Fig. 4C) and QH2-50 nm (Fig. 4D), respectively. After spectral unmixing, the QH2-nanoparticles can be identified at the site of injection by a pseudo green color. The intensity of the green color decreases according to the decrease of the concentration (OD's) of each dilution injected, showing the linearity of the optoacoustic signal strength in regards to concentration, the spectral identification potency of the approach and the acquisition of in depth information allowing for the accurate mapping of the injected pellet.
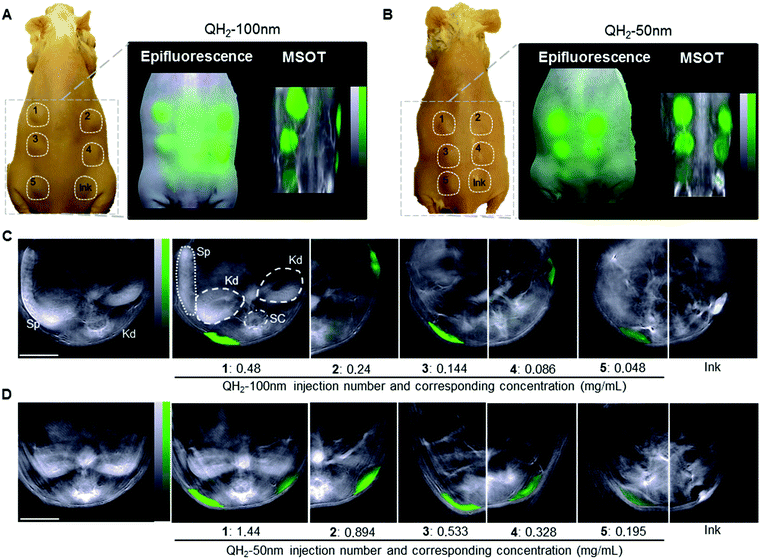 |
| Fig. 4
Ex vivo study performed on CD-1 mice after subcutaneous injection of different concentrations of QH2-nanoparticles. (A and B) Shows a color photograph of the CD-1 mouse back after SC injection with different concentration of QH2-100 nm (A, left) and QH2-50 nm (B, left) with a numbered indication of the location of the subcutaneous injections; epifluorescence imaging of QH2-100 nm (A, middle) and QH2-50 nm (B, middle) (green) at their injection sites; maximum intensity projection of multispectral optoacoustic tomography (MSOT) of QH2-100 nm (A, right) and QH2-50 nm (B, right) (green scale) overlayed on a single wavelength illumination image (800 nm, grey scale). (C and D) Coronal section by MSOT after subcutaneous injection of QH2-100 nm (C) and QH2-50 nm (D) (green scale) overlaid on a single wavelength illumination image (800 nm, grey scale). Scale bar = 3 mm. Spleen (Sp, dotted line), kidney (Kd, dashed line) and spinal cord (SC dotted-dashed circle) indicated for anatomical reference. | |
3. Discussion
The design of agents optimized for optoacoustic imaging considers criteria that optimize for (1) absorption in the near infra-red (NIR) range (700–900 nm), (2) the demonstration of a narrow optoacoustic spectrum profile necessary over broad absorption spectra for facilitating sensitive spectral unmixing,4,60 (3) the physicochemical stability, (4) photo-stability against bleaching of the absorption spectrum and (5) the biocompatibility of the nanoparticle.52 Photo-stability and biocompatibility is a highly sought after target over gold nanorods and other asymmetric near-infrared gold particles. Additional criteria considered herein include the potential for scaling the agent production and the overall size and surface functionality achieved, as it relates to ligand conjugation for biological targeting applications. Based on these criteria, the QH2-nanoparticle system produced by the FNP rapid precipitation process is a highly promising optoacoustic platform.
The selection of optoacoustic agents based on organic dyes within a nano-formulation approach is proposed herein as a highly promising strategy for biomedical optoacoustics over the use of metal nanoparticles. This proposed strategy possibly avoids the toxicity limitations of metals, offers quenching as a mechanism to reduce illumination energy lost to fluorescence and significantly improves the photo-stability of the particles in the near-infrared over metal compounds. The Flash NanoPrecipitation platform provides a flexible and scalable way to make nanoparticles with variable size (50 nm and 100 nm) that incorporate the hexacene dye at high concentrations. The strategy behind the selection of the dye was based on the requirement for hydrophobic dyes that do not partition out of the nanoparticle so that the nanoparticle biodistribution characteristics is determined solely by the PEG surface coating of the particle, and is independent of the particular hydrophobic NIR dye in the core. The hexacene dye offers a high optical cross-section in the NIR range which is then further effectively amplified when employed in nanoparticulate formulations. The larger diameter nanoparticle was found to be more photostable in storage and under NIR illumination than the smaller diameter nanoparticle. This appears to be due to the larger surface-to-volume ratio as nanoparticle size decreases.
The 5-step analysis followed in the work enabled the multi-faceted characterization of the QH2-nanoparticle system produced by the FNP rapid precipitation process, i.e.:
Step 1: nanoparticles. The highly hydrophobic QH2 dye used for the formulation of this novel class of optoacoustic contrast agents is a part of the acene family of dyes, the most studied being the anthracene, tetracene, and pentacene derivatives.53,61 The bulky side groups reduce π-stacking in the crystalline state, hence reducing the degree of self-quenching. It is fluorescent, which is not crucial for the optoacoustic application, but its absorption maximum at 730 nm puts it in the long-wavelength optical window for optoacoustic imaging. The high loading (∼80 wt%) afforded by the rapid precipitation process, FNP, produces particles with high optical contrast. FNP can produce particles from 50 nm to 400 nm in size; here we focus on 50 nm and 100 nm particles (Fig. 1). Our approach for incorporating the imaging agent differs from the standard, where imaging molecules are usually attached to the surface of the nanocarrier. By placing them in the core, a much higher optoacoustic signal can be achieved due to the larger number of dye molecules that can be packed in a 100 nm core compared to the surface (390
000 in core vs. 15
000 on surface). This approach also eliminates potential interference between imaging molecules and targeting agents at the nanoparticle surface.
FNP has been used successfully to produce block-copolymer-protected nanoparticles containing a wide range of actives including drugs, peptides, imaging agents, and inorganic structures.42,43,45–47 The process is scalable from milligram laboratory samples to a thousand kilograms per day in continuous operation.62,63 The ability to set nanoparticle composition by setting the concentrations of the inputs enables the production of nanoparticles that deliver both therapeutic compounds and imaging agents, such as QH2, in theranostic applications. Furthermore, the PEG block on the block copolymer stabilizing agent allows for the attachment of targeting ligands to the PEG surface.64
Step 2: optoacoustic performance. The optoacoustic signal intensity is essentially linearly correlated with absorbance, and absorbance is linearly correlated with nanoparticle concentration (Fig. 2). However, there is a difference in absorbance depending on nanoparticle size. The 50 nm particles are 1/3 as light absorbing as the 100 nm particles. The reason for this is associated with singlet oxygen degradation of the dye. The smaller particles, with higher surface-to-volume ratios have more dye exposed to degradation. We have observed this phenomena with other fluorescent dyes encapsulated in FNP nanoparticles.38 For QH2 dye in 30 nm micelles, the degradation rate was even higher (data not shown). The lower absorbance of the 50 nm nanoparticles is associated with degradation that occurs during normal processing and purification prior to the start of the experiments. A change in color of the QH2 dispersion from green to yellow, indicating initial degradation, was observed during processing of the smaller particles. This is also seen in Step 3 (stability). Fig. 3A shows a 10% decrease in absorbance for the 100 nm particles stored at 4 °C in the dark for a week. The 50 nm particles show in Fig. 3B a 25% decrease in absorbance over the same period. Photobleaching is shown in Fig. 3C and D, where after 30 minutes of maintained pulsed laser light illumination (far stronger than would be experienced in an in vivo experiment), the 100 nm nanoparticle has decreased 10% in maximum absorbance, similarly to AuNR, and the 50 nm decreased 60%. Both formulations remain more photostable than classical ICG. We conclude that the greater protection afforded the dye in the cores of larger nanoparticles enhances stability. It may be possible to increase the stability of smaller particles by co-encapsulating the QH2 with an antioxidant, such as tocopherol (vitamin E), which we have done previously. This would mean reducing the core loading somewhat, but the gain in stability may be significant. For this study the particles were kept in solution during formation, purification, and shipment between the USA and Germany. Putting the nanoparticles into a lyophilized form would decrease singlet oxygen attack, PEG-protected nanoparticles being easily lyophilized and redispersed back to their original size as we have already shown.65 This would be the next step in making commercial versions of these nanosized optoacoustic contrast agents.
Interestingly, the absorbance spectra of the QH2-nanoparticles present a very narrow absorbance peak with high molar extinction coefficient due to the choice of chromophores, compared to ICG and AuNR (Fig. 2F). This becomes particularly beneficial in complex media when spectral unmixing, i.e. identification of photoabsorbers according to their absorbance spectra, has to uncover the local concentration of contrast agents above tissue signal. In that case, very sharp changes in absorbance spectra allow for optimal results using different spectral unmixing techniques (wavelength subtraction, linear regression or statistical methods). By introducing the value χ (eqn (1)), representing the increase in signal between the minimum and the maximum absorbance wavelength in the near-infrared window used by in vivo MSOT, QH2-nanoparticles are revealed to provide an almost two-fold improvement over the MSOT gold-standard ICG, with significantly higher performance than AuNR.
Fig. 3E and F show that the nanoparticles have minimal toxicity at concentrations as high as 1.5 mg mL−1. For incubation with MDA MB 231 human breast cancer cells both the 100 nm and the 50 nm particles showed close to no toxicity at 1.5 mg mL−1. In contrast, introducing the dye in DMSO caused cell deaths of 60–70% in the two control experiments. By both measures of stability and toxicity the QH2 nanoparticles appear to be viable optoacoustic candidates. There is some preference to the larger particle size, which displays greater storage and photo-stability.
Step 4: biocompatibility. Biocompatibility has two components. The first is toxicity, which we have shown to be very low for these optoacoustic particles. The complete encapsulation of the very hydrophobic QH2 dye means that the cells see the dense PEG surface layer of the nanoparticle, and not the dye itself. We have shown that the PEG-protected nanoparticles are long circulating39 and do not produce a complement activation response regardless of the hydrophobic block being poly(styrene), poly(caprolactone), poly(lactide), or poly(lactide-co-glycolide).51 Recent work shows that they are mucus-penetrating, so the QH2 nanoparticles may be attractive for studying lung and GI tract nanoparticle delivery and fate. The second component of biocompatibility is long-time biological response. The polymers we have used in this proof-of-concept study are polystyrene-b-PEG. The polystyrene block will not biodegrade, and so in vivo clearance is unlikely. However, we have shown that equivalent nanoparticles can be made from biodegradable polylactic acid (PLA) or polycaprolactone (PCL) block copolymers and would be preferable for human application.43,45,47 So the transition from the PS-b-PEG to PLA-b-PEG or PCL-b-PEG would be straightforward. The ultimate clearance and long-term effects of the QH2 are unknown and would have to be evaluated to advance this platform to human subjects. For animal testing, both the PS-b-PEG/QH2 or a PLA or PCL-b-PEG/QH2 nanoparticle construct will be adequately biocompatible.
Step 5: optoacoustic sensitivity in vitro and ex vivo. The performance of QH2-nanoparticles as optoacoustic contrast agents was evaluated by a series of studies performed on agar phantoms that mimic tissue. The optoacoustic signal directly correlated with the absorption spectrum measured by spectrophotometer, confirming that the optoacoustic signal amplitude is proportional to the amount of optical energy absorbed. In both formulations, a similar imaging pattern was observed, with high intensity of optoacoustic signals that decrease with decreasing NP concentration (i.e. OD's). For the same mass concentration of the sample, the optoacoustic signal of QH2-100 nm was higher than QH2-50 nm, which correlated with the higher optical absorbance of the larger particles, when compared at the same dye loading. The performance of both QH2 nanoparticles as optoacoustic contrast agents in agar phantom was promising, as they provided an intense signal directly dependent on the amount of particles present in solution in the concentration range used as well as a very narrow absorbance peak. QH2-100 nm, however, seemed to achieve better stability when exposed to pulsed laser light, a critical advantage when aiming at longitudinal quantitative studies, inherently requiring increased imaging (and thus illumination) time. MSOT was then used to examine the sensitivity of both optoacoustic reporter probes when injected into an animal. This experiment constitutes the proof-of-concept that these formulations could indeed serve as a nanocarriers for optoacoustic imaging. After s.c. injections of the differently diluted samples, 2D planar imaging (epi-fluorescence and MSOT) identified the doses injected. At the lowest concentrations (<50 μg mL−1) the MSOT signal was clearly visible, while no signal was observable by epi-florescence. While epi-fluorescent only generates planar 2D images, without depth information, MSOT has access to 3D information through transverse axis imaging and displacement of the sample along the third spatial axis. The high sensitivity of MSOT for the QH2-nanoparticles can be justified by the high χ value. Optoacoustic imaging also enables access to other endogenous absorbers after unmixing, such as hemoglobin, allowing for vascular mapping and simultaneous oxygenation studies.10 This allows deeper tissue imaging and thus a greater spatial accuracy in bio-distribution and pharmacological studies.
Recently, liposomes encapsulating ICG have also been engineered29 as an alternative, near-infrared fluorochromes utilizing strategy over metal nanoparticles. Liposomes offer similar advantages to hexacene-dye loaded particles; however the loading of ICG encapsulated in the liposome was only 1.5 mol% of the entire nanoparticle, while the FNP nanoparticles were produced at 80 wt% of hexacene. Flash NanoPrecipitation (FNP) provided a flexible and scalable way to develop nanoparticles with variable size and high dye loading. The nanoparticles produced offer an NIR absorption cross-section similar to that of gold nanorods but with increased photo stability, significantly improving their potential use in in vivo systems or other applications over ICG. The NIR QH2-nanoparticles exhibited a narrow absorption/excitation peak at long wavelength (730 nm), that makes them ideal for optoacoustic imaging. The larger nanoparticle displayed better photostability under NIR illumination compared to the smaller nanoparticle and to ICG. The combination of exceptional photostability and sharp absorbance spectra or high χ value represents a “best of both worlds” approach for optoacoustic imaging: narrow spectral peak greatly facilitates spectral identification of the contrast agent over complex background such as living tissue, while providing a long-term photostability enabling longitudinal studies, a property classically associated with metallic nanoparticles when used at low light fluence. Overall, our data indicate that the dye is not toxic, with both particles showing minimal toxicity over the range of concentrations tested after exposure to MDA MB 231 human breast cancer cells. Nevertheless, systemic toxicity studies will be required to establish long-term toxicity and fate of the hexacene dye in human applications due to potential long-term accumulation in adipose tissue. A detailed understanding of remaining photo-instability mechanisms is planned now as a future step to guide improved strategies to overcome this limitation. One approach may be the co-formulation with anti-oxidant compounds. An important next direction is the incorporation of ligands onto the PEG steric layer to effect targeting. Our experience with creating targeted nanoparticles for therapeutic applications by FNP38,64 give us confidence that this will be an achievable objective.
4. Experimental section
4.1. QH2-nanoparticles synthesis
Materials.
QH2 dye/LW2 dye was synthesized by John Anthony, Dept. of Chemistry, University of Kentucky according to previously reported procedures53,66 and the structure is shown in Fig. 1B. The dye is known as iBu-TSBSH5, a dioxolane-functionalized hexacene, as explained in Bruzek and Anthony (2014).66 Poly(styrene)-b-poly(ethylene glycol) (PS1600-b-PEG5000) with respective block molecular weights of 1.6k and 5k (P13141) was obtained from Polymer Source (Montreal, Canada). HPLC grade THF was obtained from Fisher Scientific (T425), ultrapure water was obtained from a Barnstead Nanopure system delivering >18.0 MΩ cm. Methyl-terminated poly(ethylene glycol) of molecular weight 5k (mPEG5000) (81323) and sodium phosphate monobasic anhydrous (S3139) were obtained from Sigma Aldrich and used without further modification.
Formulation of QH2-nanoparticles and dye loaded micelles.
Two formulations of QH2-loaded nanoparticles were prepared: QH2-100 nm and QH2-50 nm. QH2 dye and PS1.6k-b-PEG5k were dissolved in THF at 6 mg mL−1 each (QH2-100 nm) and 0.25 mg mL−1 each (QH2-50 nm) and rapidly mixed against an equivalent volume of water in a confined impinging jets mixer (CIJ)67 which was quenched with 9 parts water to give an overall mixing ratio of 10
:
1 and nanoparticles containing about 80% core loading of dye. The nanoparticles were dialyzed against 5 mM phosphate buffer for 24 hours.
Lyophilization of QH2-nanoparticles.
Prior to lyophilization, the nanoparticles were 0.2 μm sterile filtered then 1
:
1 mPEG5000
:
NP mass was added to the suspensions. QH2-nanoparticle suspensions were transferred to sterile polypropylene centrifuge tubes (14-432-22, Fisher Scientific), frozen in dry ice for 30 minutes, then lyophilized at <100 mTorr on a Virtis Advantage Lyophilizer (SP Scientific) for 24–48 hours after which time a dry powder was obtained.
Concentration of QH2-nanoparticles.
QH2-100 nm and QH2-50 nm were concentrated following dialysis by placing each suspension in a 20 mL centricon centrifugal filter device (Z368253, Sigma Aldrich) and centrifuging for 20–25 minutes at 7000g. The concentrates were removed and the procedure repeated multiple times to process the entire suspension. At the end, the concentrates were collected. Absorbance measurements were taken before and after concentrating to determine the factor of concentration for each suspension.
4.2. QH2-nanoparticles characterization
Characterization of absorbance.
Dye loaded nanoparticles were diluted in THF to dissolve the particles (thus eliminating scattering effects) and keep the measured absorbance below 2 absorbance units. QH2 concentrations were quantified by the absorbance peak at 735 nm. Nanoparticle concentrations were quantified by the stoichiometry between the dye and total solids.
Characterization of fluorescence.
Fluorescence measurements were taken on QH2-nanoparticles in a glass cell with a Hitachi F-7000 Fluorometer (5 nm excitation and emission slits, 950 V PMT, 0.5 s response time). The excitation wavelength was 701 nm.
Optimization of QH2 fluorescence.
The optimal nanoparticle loading of QH2 in nanoparticle cores was determined by varying the loading of the dye and performing fluorescence measurements at multiple dilutions to ensure that the fluorescence vs. nanoparticle concentration was linear. The slope of the line was calculated as the intrinsic fluorescence of the nanoparticle system.
Spectroscopy studies.
Five different dilutions of each QH2-nanoparticles were prepared with optical densities ranging from 0.3 to 2.2. The wavelength-dependent absorption spectrum of each stock was then measured from 680 to 900 nm under Spectrophotometer USB4000 (Ocean Optics, Dunedin, FL, USA). Later the spectrum information was used as an input spectrum for multispectral post-processing of optoacoustic signals.
In vitro toxicity studies (MTT assay).
The cell viability was assessed using an 3-(4,5-dimethylthiazol-2-yl)-2,5-diphenyltetrazolium bromide (MTT) assay as previously reported.68 The MDA-MB-231 cell line, a human metastatic breast cancer cell line (HTB-26; American Type Cell Culture Collection, Manassas VA), was grown in 5% CO2 at 37 °C in DMEM medium (Sigma-Aldrich) containing 10% fetal bovine serum and antibiotics (penicillin and streptomycin, Sigma-Aldrich). Routine culture treatment was conducted twice a week. Cells were harvested and seeded in 96-well plates at 2.4 × 103 cell per well in a final volume of 100 μL. After overnight incubation for cell adhesion, the medium was removed, and the cells were incubated at 37 °C with 100 μL of medium containing QH2-100 nm (0.1–1.5 mg mL−1) or QH2-50 nm (0.1–1.5 mg mL−1), 10% dimethyl sulfoxide (DMSO) as a negative control and DMEM medium as a positive growth control. After 4 h incubation at 37 °C, the cells were washed once with 100 μL PBS followed by addition of DMEM medium. Cells were then allowed to grow for 5 days at 37 °C without changing the medium. The Cell viability was determined by a colorimetric cell viability assay (Cell Proliferation Kit 1 (MTT), Roche) used according to the manufacturer's instructions. The absorbance measurement was performed on a microplate reader (Infinite® 200 PRO, Tecan) and was determined at 595 nm, whereby, 651 nm was used as a reference wavelength. To quantify cell viability, the ratio of the absorbance of the samples to the absorbance of the non-treated control samples (=100%) was calculated. Normal cells such as NIH/3T3 were not utilized in this study as we sought to understand the effects of the pure nanoparticles without any therapeutic payload or targeting capabilities on tumor cells.
4.3. Optoacoustic imaging
Optoacoustic imaging system.
All optoacoustic measurements were performed in a real-time whole-body mouse imaging MSOT inVision 256-TF (iThera Medical, Munich, Germany). A similar version of the system was described previously.69 Briefly, optical excitation was provided by a Q-switched Nd:YAG laser with a pulse duration of ∼10 ns and a repetition rate of 10 Hz and a tunable range of 680–900 nm. Light was delivered to the sample using a fiber bundle split into 10 output arms. The emitted ultrasound signal was detected using a 256 element transducer array cylindrically focused and having a central frequency of 5 MHz, allowing acquisition of transverse plane images. The fiber bundle and transducer array were stationary, and the sample could be moved to acquire different imaging planes using a moving stage. Measurements took place in a temperature controlled water bath at 34 °C for acoustic coupling, and the samples were kept dry using a thin clear polyethylene membrane attached to the sample holder.
Scattering phantom preparation.
Cylindrical phantoms of 2 cm diameter were prepared using a gel made from distilled water containing Agar (Sigma-Aldrich, St. Louis, MO, USA) for jellification (1.3% w/w) and an intralipid 20% emulsion (Sigma-Aldrich, St. Louis, MO, USA) for light diffusion (6% v/v), resulting in a gel presenting a reduced scattering coefficient of μ's ≈ 10 cm−1. A cylindrical inclusion containing the sample of approximately 3 mm diameter was put approximately in the middle of the phantom, along with a tube containing Indian ink (company) with an absorbance of around 0.3 at 800 nm for internal signal intensity measurement references.
In vitro optoacoustic imaging.
Imaging of the phantoms was executed at a single position, located approximately in the middle of the phantom. Data acquisition was performed in the wavelength range 680–900 nm in steps of 5 nm, using 10 averages per wavelength resulting in 1 s acquisition time per wavelength. For the photobleaching studies, the data was acquired at a single position at peak absorption of QH2-loaded nanoparticles with an absorbance of 1.3 (730 nm) during prolonged pulsed laser light illumination for 30 minutes. Indocyanine Green (ICG, Pulsion Medical Systems, Germany) and gold nanorods (AuNR D12M-780, Nanopartz, USA) were used for comparison purposes at similar absorbance for spectral an stability measurements, using 780 nm as the illumination wavelength for the stability assays. Image reconstruction of phantom studies was achieved by a model-based image reconstruction published previously.70,71 The algorithm delivers more accurate performance and quantification relative to conventional back-projection algorithms. Following image reconstruction, spectral unmixing was performed using a linear model assuming that the spectra acquired were a linear mix of the QH2-loaded nanoparticles spectrum and a background spectra. For each pixel in the image, the algorithm fitted the measured optoacoustic72 spectrum, normalized for variations in laser energy per wavelength, to the known absorption spectra of the chromophores assumed in the image.
Optoacoustic imaging of animals.
8-week old female CD-1 albino mice (HelmholtzZentrum München) were injected subcutaneously ex vivo with a mixture of matrigel (25 μL) and reporter probe (25 μL) in a total of 50 μL per injected solution. Each reporter probe was injected at 5 different concentrations (absorbance of 0.3, 0.5, 0.82, 1.3, 2.2) in 5 different locations on the back of the mouse. A solution with Indian Ink (absorbance of 0.3) was also injected subcutaneous as a reference control.
Optoacoustic imaging of the QH2-loaded nanoparticles injected subcutaneously was performed by recording optoacoustic data at multiple excitation wavelengths (680 nm, 710 nm, 730 nm, 740 nm, 770 nm, 800 nm, 830 nm, 860 nm and 890 nm) using 30 averaged signals per wavelength, resulting in an acquisition time of 70 s per slice. The wavelengths were selected to resolve the QH2-nanoparticles from the background absorption of the tissue. Multiple slices were acquired at 0.5 mm intervals to cover the region where subcutaneous injections were made.
4.4. EpiFluorescent acquisition
Following the MSOT experiment, whole-body NIR fluorescence imaging was performed to confirm the presence of the different subcutaneous injections of both reporter probes within the mouse body. Imaging was performed ex vivo using epi-illumination fluorescence with a Leica Z16 macroscopic lens. Fluorescence images were captured at the peak emission wavelength of QH2 dye.
All experiments involving live animals were performed in accordance with relevant laws and institutional guidelines and policies. Approval was provided by the Institute for Biological and Medical Imaging, Neuherberg, Germany.
Conflicts of interest
There are no conflicts of interest to declare.
Acknowledgements
We would like to thank Dr Christina Tang (Department of Chemical Engineering – Virginia Commonwealth University) for obtaining TEM images of the nanoparticles. The authors also wish to acknowledge Uwe Klemm for his valuable technical support on animal handling. RKP acknowledges support from National Institutes of Health (Award No. 1RO1CA155061-1), The Princeton SEAS Old Guard Fund, The IP Accelerator Fund, the Essig Enright Fund and the Helen Shipley Fund. MJB acknowledges a fellowship from the Research Challenge Trust Fund of the University of Kentucky. Vasilis Ntziachristos acknowledges support from the Leibniz Prize from the German Ministry of Research, the Cluster of Excellence “Nanosystem Initiative Munich” as well as the ERC Advanced Grant (233161) “Next Generation in vivo imaging platform for post-genome biology and medicine MSOT”.
References
- A. Taruttis and V. Ntziachristos, Nat. Photonics, 2015, 9, 219–227 CrossRef CAS.
- J. A. Copland, M. Eghtedari, V. L. Popov, N. Kotov, N. Mamedova, M. Motamedi and A. A. Oraevsky, Mol. Imaging Biol., 2004, 6, 341–349 CrossRef PubMed.
- L. M. Nie and X. Y. Chen, Chem. Soc. Rev., 2014, 43, 7132–7170 RSC.
- V. Ntziachristos and D. Razansky, Chem. Rev., 2010, 110, 2783–2794 CrossRef CAS PubMed.
- V. Ntziachristos, Nat. Methods, 2010, 7, 603–614 CrossRef CAS PubMed.
- W. W. Li and X. Y. Chen, Nanomedicine, 2015, 10, 299–320 CrossRef CAS PubMed.
- M. L. Li, J. C. Wang, J. A. Schwartz, K. L. Gill-Sharp, G. Stoica and L. H. V. Wang, J. Biomed. Opt., 2009, 14, 010507 CrossRef PubMed.
- S. Kim, Y. S. Chen, G. P. Luke and S. Y. Emelianov, Biomed. Opt. Express, 2011, 2, 2540–2550 Search PubMed.
- C. C. Bao, N. Beziere, P. del Pino, B. Pelaz, G. Estrada, F. R. Tian, V. Ntziachristos, J. M. de la Fuente and D. X. Cui, Small, 2013, 9, 68–74 CrossRef CAS PubMed.
- E. Herzog, A. Taruttis, N. Beziere, A. A. Lutich, D. Razansky and V. Ntziachristos, Radiology, 2012, 263, 461–468 CrossRef PubMed.
- G. P. Luke, A. Bashyam, K. A. Homan, S. Makhija, Y. S. Chen and S. Y. Emelianov, Nanotechnology, 2013, 24, 455101 CrossRef PubMed.
- A. V. Liopo, A. Conjusteau, O. V. Chumakova, S. A. Ermilov, R. Su and A. A. Oraevsky, Nanosci. Nanotechnol. Lett., 2012, 4, 681–686 CrossRef CAS PubMed.
- M. O'Donnell, E. R. McVeigh, H. W. Strauss, A. Tanaka, B. E. Bouma, G. J. Tearney, M. A. Guttman and E. V. Garcia, J. Nucl. Med., 2010, 51, 38S–50S CrossRef PubMed.
- J. Zhong, L. Wen, S. Yang, L. Xiang, Q. Chen and D. Xing, Nanomedicine, 2015, 11, 1499–1509 CrossRef CAS PubMed.
- D. Pan, M. Pramanik, A. Senpan, J. S. Allen, H. Y. Zhang, S. A. Wickline, L. V. Wang and G. M. Lanza, FASEB J., 2011, 25, 875–882 CrossRef CAS PubMed.
- D. P. J. Pan, M. Pramanik, A. Senpan, S. Ghosh, S. A. Wickline, L. V. Wang and G. M. Lanza, Biomaterials, 2010, 31, 4088–4093 CrossRef CAS PubMed.
- A. Taruttis, N. Lozano, A. Nunes, D. A. Jasim, N. Beziere, E. Herzog, K. Kostarelos and V. Ntziachristos, Nanoscale, 2014, 6, 13451–13456 RSC.
- C. Kim, E. C. Cho, J. Y. Chen, K. H. Song, L. Au, C. Favazza, Q. A. Zhang, C. M. Cobley, F. Gao, Y. N. Xia and L. H. V. Wang, ACS Nano, 2010, 4, 4559–4564 CrossRef CAS PubMed.
- M. Goetz and T. D. Wang, Gastroenterology, 2010, 138, 828–833 CrossRef CAS PubMed.
- S. J. Yoon, A. Murthy, K. P. Johnston, K. V. Sokolov and S. Y. Emelianov, Opt. Express, 2012, 20, 29479–29487 CrossRef CAS PubMed.
- Y.-S. Chen, W. Frey, S. Kim, K. Homan, P. Kruizinga, K. Sokolov and S. Emelianov, Opt. Express, 2010, 18, 8867–8878 CrossRef CAS PubMed.
- L.-C. Chen, C.-W. Wei, J. S. Souris, S.-H. Cheng, C.-T. Chen, C.-S. Yang, P.-C. Li and L.-W. Lo, J. Biomed. Opt., 2010, 15, 016010 CrossRef PubMed.
- J. M. Tam, A. K. Murthy, D. R. Ingram, R. Nguyen, K. V. Sokolov and K. P. Johnston, Langmuir, 2010, 26, 8988–8999 CrossRef CAS PubMed.
- K. Kostarelos, Nat. Biotechnol., 2008, 26, 774–776 CrossRef CAS PubMed.
- P. P. Simeonova, Nanomedicine, 2009, 4, 373–375 CrossRef CAS PubMed.
- X. D. Zhang, D. Wu, X. Shen, P. X. Liu, N. Yang, B. Zhao, H. Zhang, Y. M. Sun, L. A. Zhang and F. Y. Fan, Int. J. Nanomed., 2011, 6, 2071–2081 CrossRef CAS PubMed.
- A. Nunes, K. T. Al-Jamal and K. Kostarelos, J. Controlled Release, 2012, 161, 290–306 CrossRef CAS PubMed.
- A. Nunes, K. Al-Jamal, T. Nakajima, M. Hariz and K. Kostarelos, Arch. Toxicol., 2012, 86, 1009–1020 CrossRef CAS PubMed.
- N. Beziere, N. Lozano, N. Nunes, J. Salichs, D. Queiros, K. Kostarelo and V. Ntziachristos, Biomaterials, 2015, 37, 415–424 CrossRef CAS PubMed.
- J. T. Alander, I. Kaartinen, A. Laakso, T. Patila, T. Spillmann, V. V. Tuchin, M. Venermo and P. Valisuo, Int. J. Biomed. Imaging, 2012, 2012, 940585 Search PubMed.
- K. Y. Pu, A. J. Shuhendler, J. V. Jokerst, J. G. Mei, S. S. Gambhir, Z. N. Bao and J. H. Rao, Nat. Nanotechnol., 2014, 9, 233–239 CrossRef CAS PubMed.
- B. K. Johnson and R. K. Prud'homme, Aust. J. Chem., 2003, 56, 1021–1024 CrossRef CAS.
- M. Akbulut, S. M. D'Addio, M. Gindy and R. K. Prud’homme, Expert Rev. Clin. Pharmacol., 2009, 2, 265–282 CrossRef CAS PubMed.
- M. Akbulut, P. Ginart, M. Gindy, C. Theriault, K. Chin, W. Soboyejo and R. K. Prud'homme, Adv. Funct. Mater., 2009, 19, 1–8 CrossRef.
- S. M. D'Addio and R. K. Prud'homme, Adv. Drug Delivery Rev., 2011, 63, 417–426 CrossRef PubMed.
- M. E. Gindy and R. K. Prud'homme, Expert Opin. Drug Delivery, 2009, 6, 865–878 CrossRef CAS PubMed.
- A. L. Klibanov, K. Maruyama, V. P. Torchilin and L. Huang, FEBS Lett., 1990, 268, 235–237 CrossRef CAS PubMed.
- M. Akbulut, P. Ginart, M. E. Gindy, C. Theriault, K. H. Chin, W. Soboyejo and R. K. Prud'homme, Adv. Funct. Mater., 2009, 19, 718–725 CrossRef CAS.
- S. M. Ansell, S. A. Johnstone, P. G. Tardi, L. Lo, S. Xie, Y. Shu, T. O. Harasym, N. L. Harasym, L. Williams, D. Bermudes, B. D. Liboiron, W. Saad, R. K. Prud’homme and L. D. Mayer, J. Med. Chem., 2008, 51, 3288–3296 CrossRef CAS PubMed.
- S. J. Budijono, J. Shan, N. Yao, Y. Miura, T. Hoye, R. H. Austin, Y. Ju and R. K. Prud’homme, Chem. Mater., 2010, 22, 311–318 Search PubMed.
- T. Chen, S. M. D’Addio, M. T. Kennedy, A. Swietlow, I. G. Kevrekidis, A. Z. Panagiotopoulos and R. K. Prud’homme, Nano Lett., 2009, 9, 2218–2222 CrossRef CAS PubMed.
- M. E. Gindy, S. Ji, T. R. Hoye, A. Z. Panagiotopoulos and R. K. Prud’homme, Biomacromolecules, 2008, 9, 2705–2711 CrossRef CAS PubMed.
- M. E. Gindy, A. Z. Panagiotopoulos and R. K. Prud'homme, Langmuir, 2007, 24, 83–90 CrossRef PubMed.
- B. K. Johnson and R. K. Prud'homme, Aust. J. Chem., 2003, 56, 1021–1024 CrossRef CAS.
- V. Kumar, D. H. Adamson and R. K. Prud’homme, Small, 2010, 6, 2907–2914 CrossRef CAS PubMed.
- V. Kumar, S. Y. Hong, A. E. Maciag, J. E. Saavedra, D. H. Adamson, R. K. Prud’homme, L. K. Keefer and H. Chakrapani, Mol. Pharmaceutics, 2009, 7, 291–298 CrossRef PubMed.
- V. Kumar, L. Wang, M. Riebe, H.-H. Tung and R. K. Prud’homme, Mol. Pharmaceutics, 2009, 6, 1118–1124 CrossRef CAS PubMed.
- V. Pansare, M. Bruzek, D. Adamson, J. Anthony and R. Prud’homme, Mol. Imaging Biol., 2014, 16, 180–188 CrossRef PubMed.
- S. M. D'Addio, S. Baldassano, L. Shi, L. L. Cheung, D. H. Adamson, M. Bruzek, J. E. Anthony, D. L. Laskin, P. J. Sinko and R. K. Prud'homme, J. Controlled Release, 2013, 168, 41–49 CrossRef PubMed.
- M. E. Gindy, S. X. Ji, T. R. Hoye, A. Z. Panagiotopoulos and R. K. Prud'homme, Biomacromolecules, 2008, 9, 2705–2711 CrossRef CAS PubMed.
- S. M. D'Addio, W. Saad, S. M. Ansell, J. J. Squiers, D. H. Adamson, M. Herrera-Alonso, A. R. Wohl, T. R. Hoye, C. W. Macosko, L. D. Mayer, C. Vauthier and R. K. Prud'homme, J. Controlled Release, 2012, 162, 208–217 CrossRef PubMed.
- G. P. Luke, D. Yeager and S. Y. Emelianov, Ann. Biomed. Eng., 2012, 40, 422–437 CrossRef PubMed.
- J. E. Anthony, Chem. Rev., 2006, 106, 5028–5048 CrossRef CAS PubMed.
- J. E. Anthony, D. L. Eaton and S. R. Parkin, Org. Lett., 2001, 4, 15–18 CrossRef.
- O. L. Griffith, J. E. Anthony, A. G. Jones, Y. Shu and D. L. Lichtenberger, J. Am. Chem. Soc., 2012, 134, 14185–14194 CrossRef CAS PubMed.
- M. M. Payne, J. H. Delcamp, S. R. Parkin and J. E. Anthony, Org. Lett., 2004, 6, 1609–1612 CrossRef CAS PubMed.
- M. M. Payne, S. R. Parkin and J. E. Anthony, J. Am. Chem. Soc., 2005, 127, 8028–8029 CrossRef CAS PubMed.
- M. A. Wolak, J. S. Melinger, P. A. Lane, L. C. Palilis, C. A. Landis, J. E. Anthony and Z. H. Kafafi, J. Phys. Chem. B, 2006, 110, 10606–10611 CrossRef CAS PubMed.
- M. A. Wolak, J. S. Melinger, P. A. Lane, L. C. Palilis, C. A. Landis, J. Delcamp, J. E. Anthony and Z. H. Kafafi, J. Phys. Chem. B, 2006, 110, 7928–7937 CrossRef CAS PubMed.
- S. Tzoumas, A. Nunes, N. C. Deliolanis and V. Ntziachristos, J. Biophotonics, 2014, 9999, 629–637 Search PubMed.
- M. M. Payne, S. R. Parkin and J. E. Anthony, J. Am. Chem. Soc., 2005, 127, 8028–8029 CrossRef CAS PubMed.
- B. K. Johnson and R. K. Prud'homme, AIChE J., 2003, 49, 2264–2282 CrossRef CAS.
- B. K. Johnson and R. K. Prud'homme, Aust. J. Chem., 2003, 56, 1021–1024 CrossRef CAS.
- S. M. D'Addio, S. Baldassano, L. Shi, L. Cheung, D. H. Adamson, M. Bruzek, J. E. Anthony, D. L. Laskin, P. J. Sinko and R. K. Prud'homme, J. Controlled Release, 2013, 168, 41–49 CrossRef PubMed.
- C. E. Figueroa, P. Reider, P. Burckel, A. A. Pinkerton and R. K. Prud’homme, Ther. Delivery, 2013, 3, 1269–1279 CrossRef.
- M. J. Bruzek and J. E. Anthony, Org. Lett., 2014, 16, 3608–3610 CrossRef CAS PubMed.
- J. Han, Z. Zhu, H. Qian, A. R. Wohl, C. J. Beaman, T. R. Hoye and C. W. Macosko, J. Pharm. Sci., 2012, 101, 4018–4023 CrossRef CAS PubMed.
- J. Reber, H. Struthers, T. Betzel, A. Hohn, R. Schibli and C. Muller, Mol. Pharmaceutics, 2012, 9, 1213–1221 CrossRef CAS PubMed.
- A. Buehler, E. Herzog, D. Razansky and V. Ntziachristos, Opt. Lett., 2010, 35, 2475–2477 CrossRef PubMed.
- A. Buehler, A. Rosenthal, T. Jetzfellner, A. Dima, D. Razansky and V. Ntziachristos, Med. Phys., 2011, 38, 1694–1704 CrossRef PubMed.
- A. Rosenthal, D. Razansky and V. Ntziachristos, IEEE Trans. Med. Imaging, 2010, 29, 1275–1285 CrossRef PubMed.
- D. Razansky, M. Distel, C. Vinegoni, R. Ma, N. Perrimon, R. W. Koster and V. Ntziachristos, Nat. Photonics, 2009, 3, 412–417 CrossRef CAS.
Footnote |
† Electronic supplementary information (ESI) available: Fig. S1: optimization of QH2 core loading for maximum fluorescent per particle. The fluorescence per particle is represented by the slope of fluorescence vs. concentration of nanoparticles. Fig. S2: spectrophotometer analysis of QH2 nanoparticles. Spectrophotometer measurements at five different concentrations for (A) QH2-100 nm and (B) QH2-50 nm. Fig. S3: performance of QH2 loaded nanoparticles as optoacoustic contrast agents. Optoacoustic spectra of QH2-100 nm (A) and QH2-50 nm (B) at different concentrations. Fig. S4: optoacoustic signal generation of QH2-100 nm at OD2.2, revealing the narrow optoacoustic band of QH2 nanoparticles. See DOI: 10.1039/c7tb02633a |
|
This journal is © The Royal Society of Chemistry 2018 |
Click here to see how this site uses Cookies. View our privacy policy here.