DOI:
10.1039/C8AN02342E
(Paper)
Analyst, 2019,
144, 3843-3852
A novel label-free terbium(III)-aptamer based aptasensor for ultrasensitive and highly specific detection of acute lymphoma leukemia cells
Received
3rd December 2018
, Accepted 8th March 2019
First published on 17th May 2019
Abstract
Acute leukemia is a malignant clonal disease of hematopoietic stem cells with a high prevalence and mortality rate. However, there are no efficient tools to facilitate early diagnosis and treatment of leukemia. Therefore, development of new methods for the early diagnosis and prevention of leukemia, especially non-invasive diagnosis at the cellular level, is imperative. Here, a label-free signal-on fluorescence aptasensor based on terbium(III)-aptamer (Tb3+-apt) was applied for the detection of leukemia. The aptamer sensitizes the fluorescence of Tb3+ and forms the strong fluorescent Tb3+-apt probe. The target cells, the T-cell acute lymphoblastic leukemia cell line (CCRF-CEM) combined with the Tb3+-apt probe to form the Tb3+-apt–CEM complex, were removed by centrifugation, and the supernatant containing a small amount of the Tb3+-apt probe was detected using a fluorescence spectrophotometer. The logarithm of cell concentration showed a good linear relationship (R2 = 0.9881) with the fluorescence signal. The linear range for CCRF-CEM detection was 5–5 × 106 cells per ml, while the detection limit was 5 cells per ml of the binding buffer. Clinical samples were collected from 100 cases, and the specificity and positive rates detected by this method were up to 94% and 90%, respectively. Therefore, a single-stranded DNA-sensitized terbium(III) luminescence method diagnostic was developed which is rapid, sensitive, and economical and can be used for diagnosis of various types of leukemia at the early stage.
1. Introduction
Presently, the threat to human health and life due to high incidence and mortality of refractory cancers is rising annually.1 Acute lymphoblastic leukemia is a common and fatal cancer that usually begins in the bone marrow, and is characterized by the presence of a large number of immature white blood cells in children and adolescents.2,3 This disease not only demands a lot of medical and health resources, but also negatively affects the people's living standards and social welfare. Acute leukemia can lead to abnormal proliferation and accumulation of primary cells in the bone marrow and inhibit hematopoietic development, leading to anemia, thrombocytopenia and neutropenia. Moreover, the primary cells can also infiltrate the liver, spleen and other external tissues to cause corresponding lesions.2 Extracting peripheral blood cells and the bone marrow is one of the mainstay methods used to detect leukemia in clinics, although other testing methods are used,3 including fluorescent labeling,4–6 cytochemistry,7,8 flow cytometry,9–11 immunohistochemical12,13 and immunophenotype analysis, and aptamer-conjugated polymeric nanoparticles.14,15 However, most of these methods not only are costly, time-consuming, complicated and labor-intensive, but also require a combination of sophisticated instruments, which have low sensitivity and demand multiple step processing making them unsuitable for simple and rapid medical analysis. Therefore, there is a need to explore a highly specific, sensitive and cost-effective method to facilitate early diagnosis and treatment of leukemia. This especially demands for non-invasive diagnostic and prevention techniques targeted at the cellular level based on modern analytical chemistry to improve the treatment and survival rates of the patients.
In order to get highly specific targeting probes, scientists have used systematic evolution of ligands by exponential enrichment (SELEX) to screen for the new type of molecular recognition elements – nucleic acid aptamers.16–18 Due to their characteristic high affinity to target molecules, aptamers are often referred to as “chemical antibodies”.19 Compared with antibodies, aptamers are cheaper, less invasive and easily reproducible due to the unambiguous primary structure.20–22 Aptamers can bind not only to inorganic ions, small molecules, biological macromolecules, but also to supramolecular substances such as cells, viruses, and pathological tissue sections.23–25 Moreover, the synthesis of aptamers is easy and uses short cycles,26,27 and aptamers show good stability28 and are easy to modify.29,30 The emergence of aptamers has provided a new identification tool to the biochemistry and the biomedical communities. In recent years, nucleic aptamer-based biosensors have shown promising applications in the detection of cancer.31,32 In 2006, Tan et al. first selected the live tumor cell line,33 human acute lymphoblastic leukemia cell line CCRF-CEM, as target cells to screen for high specificity and affinity sgc8 aptamers. Subsequently, the sgc8 aptamer has found wide usage in the detection of acute lymphoblastic leukemia.34,35
Rare earth terbium ion (Tb3+) is a promising non-labeled fluorescent probe that has attracted a great deal of attention36 because of its unique optical properties, such as a long fluorescence lifetime, large Stokes shift and narrow emission band.37,38 Fu et al. reported that single-stranded oligonucleotides can enhance the fluorescence of rare earth terbium ions (Tb3+), but not the double-stranded DNA.39 Another study reported that G base-rich single-stranded oligonucleotides can effectively sensitize the fluorescence of Tb3+.40 Based on these reports, Wei et al. designed a G-base-rich hairpin probe which sensitizes the Tb3+ fluorescence. The heavy metal lead (Pb2+) competitively binds this hairpin probe to quench the fluorescence of Tb3+. This strategy allows a highly sensitive non-labeled detection of Pb2+ (detection limit of 0.1 nM).41
Inspired by the above studies, this study leveraged on the characteristics of nucleic acid aptamers and single-stranded DNA sensitized rare earth Tb3+ fluorescence to design a non-labeled signal-sensitive fluorescence method for the detection of CCRF-CEM cells. This method is based on the principle that the aptamer sensitizes the fluorescence of Tb3+ and forms a strong fluorescent Tb3+-apt probe. After the target cells CCRF-CEM combined with Tb3+-apt to form a Tb3+-apt–CEM complex, the supernatant is obtained by centrifugation, and then the supernatant was detected by fluorescence spectrophotometer. The concentration of CCRF-CEM cells in 5–5 × 106 cells per ml showed a good linear relationship between the logarithmic value and the fluorescence signal (R2 = 0.9881), and the detection limit was up to 5 cells per ml. This label-free signal sensitized fluorescence method has the advantages of high selectivity, easy operation and rapidity and is expected to provide a robust, simple, and inexpensive novel method for the ultrasensitive diagnosis of acute lymphoblastic leukemia.
2. Experimental
2.1. Materials
The label-Free Sgc8 aptamer (apt) with a sequence of 5′-ATCTAACTGCTGC GCCGCCGGGAAAATACTGTACGGTTAGA-3′ and the label-Free random aptamer (apt-) with a sequence of 5′-ATGTGGCTGCTGCGCCGCCGGGA AAATACTGT ACGGTTAGA-3′ were purchased from Shanghai Sangon Biological Engineering Technology & services (Shanghai, China). 4-(2-hydroxyethyl) piperazine-1-ethanesulfonic acid (HEPES, pH = 7.2–7.4, >99.5%) and the nitrate of metal salts (>99%), including potassium (K+), sodium (Na+), silver (Ag+), magnesium (Mg2+), calcium (Ca2+), copper (Cu2+), zinc (Zn2+), iron (Fe3+), and terbium (Tb3+), were purchased from Sigma-Aldrich, Inc. All solutions were produced with ultra-pure water of 18.2 MΩ purified from a Milli-Q purification system (Milli-Pore, Bedford, MA, USA). CCRF-CEM, Ramos (human Burkitt's lymphoma cell lines) cells, and K562 (chronic myelocytic leukemia cell lines), HL-60 (human promyelocytic leukemic cell lines), Thp-1 (mononuclear phagocyte system) and U937 (tissue cell lymphoma cells) cell lines were purchased from the Cell Bank of the Chinese Academy of Sciences (Shanghai, China).
2.2. Instruments
All fluorescence measurements were carried out in a 350 μL quartz cuvette. The excitation wavelength was 292 nm, and the emission spectra were recorded with both excitation and emission slits of 5 nm using an F-7000 fluorescence spectrophotometer (Hitachi Company, Tokyo, Japan). The peak intensities were obtained at 545 nm, and the sampling range was recorded from 460 nm to 560 nm. All the atomic force microscopy imaging was performed by fluorescence microscopy (Nikon DS-Ri1; Nikon, Tokyo, Japan). Cell Counting Kit-8 was bought from Dojindo, Japan.
2.3. Cell culture
CCRF-CEM, Ramos cells, and K562, HL-60, Thp-1 and U937 cell lines were cultured in 5% carbon dioxide (95% oxygen) at 37 °C for 3 days and the medium of 1640 which contained 10% fetal bovine serum (FBS, Gibco) and 100 U mL−1 penicillin–streptomycin (Gibco, Grand Island, NY, USA).
2.4. Preparation of the Tb3+-apt fluorescent aptasensor
A suitable aptamer (50 μM) diluted by 252 μl PBS (phosphate-buffered saline, 10 mM, pH 7.2) and Tb3+ solution diluted by the same volume HEPES buffer (200 mM, 1 mM magnesium nitrate, pH 7.2) were put in storage solution at 4 °C in the dark. Magnesium ions were used to stabilize the oligonucleotides’ conformation and decrease the non-specific binding between other metal ions and the oligonucleotides. The final concentrations of the oligonucleotides (5 μM oligonucleobases) and terbium nitrate (20 mM) were prepared in PBS. For example, an application-type mixture (5 μM oligonucleobases, 20 mM Tb3+) was formed by adding 160 μl PBS to 40 μl storage solution.
2.5. Optimization of the fluorescent aptasensor
To optimize the Tb3+ concentration and detection conditions, the probe preparation method is the same as the preparation of the Tb3+-apt fluorescent aptasensor above, just fixing one condition to optimize the others. Different concentrations of Tb3+ (5 mM, 10 mM, 15 mM, 20 mM, 25 mM, 30 mM) with oligonucleotides (5 μM) were detected. In order to explore the effect of pH value on fluorescence intensity of Tb3+, the fluorescence intensity of Tb3+ (20 mM) with oligonucleotides (5 μM) in the application-type mixture (pH 4.8, pH 6.0, pH 7.2, pH 8.4, pH 9.6, pH 11.0, pH 12.0, pH 13.0) was detected. And the fluorescence intensity of Tb3+ (20 mM) with oligonucleotides (5 μM) after incubation with CCRF-CEM (1 × 106 cells per 200 μl) at room temperature for different time periods (5 min, 10 min, 20 min, 30 min, 40 min, 60 min) was also detected. All cell samples were centrifuged at 1000 rpm for 3 min, then 160 μl of the supernatant was collected, and the pH value was adjusted to 9.6 for detection by fluorescence spectroscopy at the wavelength range of 460 nm–560 nm. Fluorescence intensity of Tb3+ (20 mM) with oligonucleotides (5 μM) after 10 min of incubation with CCRF-CEM (1 × 106 cells per 200 μl) at 4 °C, 25 °C and 37 °C was detected, too. Data are expressed as the mean ± SD of 3 independent experiments; the difference between fluorescence intensities obtained by the rank sum test is statistically significant (P < 0.05).
2.6. Probe for the detection of cells
A suitable aptamer (50 μM) diluted by 252 μl PBS (10 mM, pH 7.2) and a Tb3+ solution diluted by the same volume of HEPES buffer (200 mM, 1 mM magnesium nitrate, pH 7.2) were used to prepare the probe storage solution at 4 °C in the dark. 1 ml of CCRF-CEM cells were collected after centrifugation, the cells were washed three times with PBS, and then the supernatant was drained and re-suspended in 160 μl PBS. Finally, 40 μl of probe storage solution was added and mixed well. The different concentrations of CCRF-CEM cells (1 to 1 × 106 cells per 200 μl) were incubated with the Tb3+-apt fluorescent aptasensor at 4 °C in the dark for 10 min. The samples were centrifuged at 1000 rpm for 3 min, then 160 μl of the supernatant was collected, and the pH value was adjusted to 9.6 for detection by fluorescence spectroscopy at the wavelength range of 460 nm–560 nm.
2.7. Specificity assay
The fluorescence spectrophotometer detected different blood-related cancer cells. To investigate the specificity of the Tb3+-apt fluorescent aptasensor, an F-7000 fluorescence spectrophotometer and a confocal fluorescence microscope were used to test different cells, including CCRF-CEM, HL-60 cells, Ramos cells, K562 cells, PBL cells (peripheral blood lymphocytes), Thp-1 cells and U937 cells. Each of the 200 μl reaction systems including 1 × 106 cells was centrifuged at 1000 rpm for 3 min to collect 160 μl (removing fluid that may contain cells at the bottom of 40 μl) of the supernatant, and the pH value was adjusted to 9.6 for detection using the F-7000 fluorescence spectrophotometer.
Immunofluorescence imaging was performed in different blood-related cancer cells. Cells were collected in a 1.5 ml centrifugation tube, washed three times with PBS, incubated with the Tb3+-apt fluorescent aptasensor at 4 °C in the dark for 10 min and then washed again with PBS. The cells were fixed with 4% paraformaldehyde (Sigma-Aldrich, St Louis, MO, USA) for 10 min, washed with PBS, and stained with 4′,6-diamidino-2-phenylindole dihydrochloride (DAPI; Life Technologies, Foster City, CA, USA) for 5 min in the dark. The cells were finally washed with PBS and examined by fluorescence microscopy.
2.8. Detection of clinical samples
Blood samples were collected from 20 ALL (acute lymphoblastic leukemia) patients, 60 non-ALL patients, and 20 normal subjects based on clinically diagnosed classifications. All samples were from the First Affiliated Hospital of Guangxi Medical University. These experiments were approved by the Ethics Committee of Guangxi Medical University, and patient's informed consent was obtained before the experiment. 5 ml of peripheral blood of each case was obtained with anticoagulants, and human peripheral blood lymphocyte separation solution (Solarbio, Cat. No. P8610) was added for gradient centrifugation at a rotation speed of 1000g for 30 min. The white film layer between plasma and separation solution was taken. 200 μl of the reaction systems (detection of the cell reaction system above) was added, incubated for 10 min, and then centrifuged at 1000 rpm for 3 min. 160 μl of the supernatant was obtained, the pH value was adjusted to 9.6, and the supernatant fluorescence was detected using the F-7000 fluorescence spectrophotometer.
2.9. Statistical analyses
Each experiment was carried out in triplicate. Data are expressed as the mean ± SD or as the median (range). All statistical analyses were performed using GraphPad Prism 6.02 (GraphPad Software, San Diego, CA, USA). P < 0.05 was considered statistically significant.
3. Results and discussion
3.1. Principle of the Tb3+-apt fluorescent aptasensor
Based on the characteristics of nucleic acid aptamers and single-stranded DNA sensitized rare earth Tb3+ fluorescence, a label-free signal sensitized fluorescence method was developed in this study for the detection of CCRF-CEM cells in acute lymphoblastic leukemia. The detection principle is shown in Scheme 1. The hairpin type single-chain aptamer (the yellow hairpin structure sequence in the figure) can sensitize Tb3+ (green particles in the figure) fluorescence. The Tb3+-apt probe can recognize the CCRF-CEM cells specifically to form the Tb3+-apt–CEM complex, and the supernatant of the Tb3+-apt–CEM complex collected by centrifugation may be used for fluorescence detection. Compared with the Tb3+-apt–nontarget cells complex, the fluorescence signal of the corresponding supernatant was significantly reduced. In contrast, in the absence of the CCRF-CEM cells, the Tb3+-apt probe was still present in the supernatant and there was no significant change in the fluorescence strength.
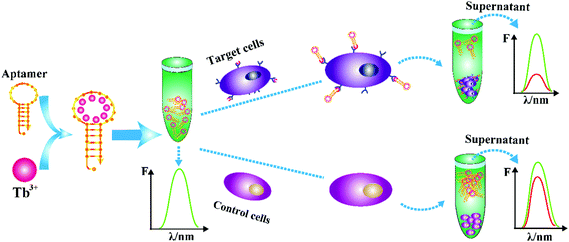 |
| Scheme 1 Schematic illustration of the Tb3+-apt fluorescent aptasensor for detection of CCRF-CEM cells. | |
3.2. Characterization of Tb3+ fluorescence
The feasibility of this method was first verified by fluorescence spectroscopy, and the results are shown in Fig. 1A. The fluorescence intensity of Tb3+-apt was the strongest. When the non-target Romas cells were added to the Tb3+-apt probe solution, there was barely any change in the fluorescence intensity, whereas the fluorescence intensity was significantly decreased when the CEM cells were added. And when the non-target G-base rich single stranded oligonucleotides Tb3+-apt(−) were incubated with the CEM cells, the fluorescence intensity was hardly attenuated. A statistical graph showed similar results (Fig. 1B). The PTK7 protein on the surface of the CEM cell membrane specifically binds to the Sgc8 aptamer, and the target cells then removed the probe by centrifugation, leaving almost no Tb3+-apt probe in the supernatant, leading to a rapid decrease of the fluorescence. While the individual Tb3+, apt, CEM, and Romas cells are almost non-fluorescent, the Tb3+-apt probe emits green fluorescence under ultraviolet light. In order to confirm the characteristics of Tb3+ sensitized specificity of DNA, Tb3+ and other different ions in the physical environment were reacted with aptamers. The results showed that when the aptamer binds to the terbium ion, the fluorescence is markedly enhanced, whereas the other metal ions (including K+, Na+, Ag+, Cu2+, Zn2+, Mg2+, Ca2+, Fe3+) show almost no fluorescence when they are bound to the aptamer (Fig. 1C), and a statistical graph of the reaction of Tb3+ and other different ions with aptamers showed a similar trend (Fig. 1D). This result also indicates that the hairpin type single-chain aptamer can sensitize Tb3+ fluorescence. However, silver, calcium, copper, iron, potassium, magnesium, and sodium ions do not have this function. The specific principle is similar to the hairpin structure of aptamers that may be affected by the coordination of strontium ions and aptamer polyguanine rings studied.41
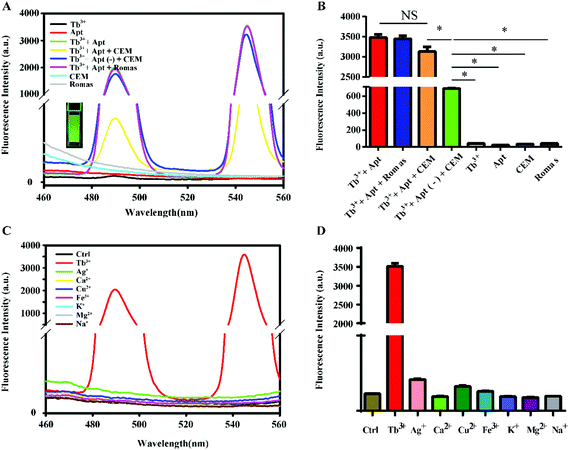 |
| Fig. 1 (A) Fluorescence emission spectra of Tb3+, apt and CCRF-CEM cells under different conditions: Tb3+; Apt (5 uM); Tb3+ + apt; Tb3+ + apt + CCFF-CEM; Tb3+ + apt(−) + CCFF-CEM; Tb3+ + apt + Romas; Tb3+ (20 mM); CCRF-CEM (1 × 106 cells); Romas (1 × 106 cells). Excitation: 290 nm. (B) Quantitative analysis of fluorescence emission spectra of Tb3+, apt, apt(−) and CCRF-CEM under different conditions. NS, not statistically significant, *P < 0.05, statistically significant. (C) Fluorescence emission spectra of the aptamer with different metal ions. When the Sgc8 aptamer combines with the terbium ion, the fluorescence is markedly enhanced, whereas the other metal ions barely show any fluorescence when mixed with the Sgc8 aptamer. (D) Statistical analysis of fluorescence emission spectra of the aptamer with different metal ions, showing significant differences between Tb3+ and other ions that mixed with the aptamer. | |
3.3. Experimental condition optimization
The experimental conditions were optimized, and the results are shown in Fig. 2. The aptamer concentration was fixed at 5 μM, and the Tb3+ concentration was then optimized. When the concentration of Tb3+ was 20 mM, the fluorescence intensity was the highest (Fig. 2A), so 20 mM Tb3+ was selected as the optimal concentration. By adjusting the pH of the probe (Tb3+-apt) solution, it was found that the fluorescence was strongest when the pH was 9.6; thus, pH = 9.6 was chosen (Fig. 2B). When the incubation time was in the range of 5 to 10 min, the fluorescence intensity increased with time, while the incubation time in the range of 10 to 30 min led to a plateau in the fluorescence intensity. Considering the time efficiency, 10 min was selected as the optimum incubation time for follow-up experiments (Fig. 2C). At the same time, the fluorescence was most intense when incubated at 4 °C (Fig. 2D); therefore, 4 °C was chosen as the optimal hybridization temperature.
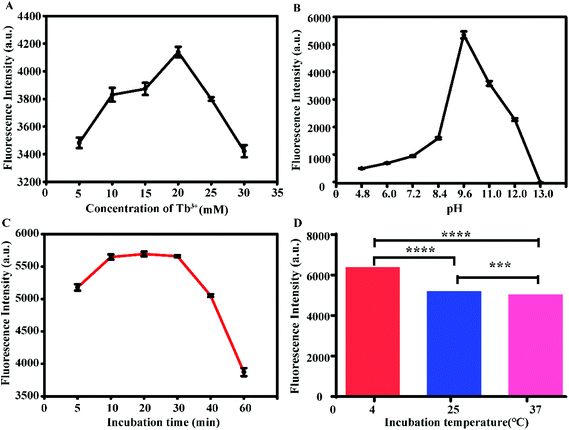 |
| Fig. 2 Optimization of Tb3+ concentration and detection conditions. (A) Concentration of Tb3+ (5 mM, 10 mM, 15 mM, 20 mM, 25 mM, 30 mM) with oligonucleotides (5 μM). (B) Fluorescence intensity of Tb3+ (20 mM) with oligonucleotides (5 μM) in the application-type mixture (pH 4.8, pH 6.0, pH 7.2, pH 8.4, pH 9.6, pH 11.0, pH 12.0, pH 13.0). (C) Fluorescence intensity of Tb3+ (20 mM) with oligonucleotides (5 μM) after incubation with CCRF-CEM (1 × 106 cells per 200 μl) at room temperature for different incubation periods. (D) Fluorescence intensity of Tb3+ with oligonucleotides in the application-type mixture (5 μM oligonucleobases, 20 mM Tb3+, pH 7.2) after 10 min of incubation with CCRF-CEM (1 × 106 cells per 200 μl) at 4 °C, 25 °C and 37 °C. Data are expressed as the mean ± SD of 3 independent experiments; ***P < 0.001. | |
3.4. Analytical performance of the aptasensor
Under the selected optimal conditions, the sensitivity of the fluorescence method was examined. As shown in Fig. 3A, the difference in the fluorescence intensity gradually decreases with the increase of the concentration of the CEM cells (a. Tb3+-apt; b. 100 cells per 200 μl, c. 101 cells per 200 μl, d. 102 cells per 200 μl, e. 103 cells per 200 μl, f. 104 cells per 200 μl, g. 105 cells per 200 μl, h. 106 cells per 200 μl). In the range of 1 to 106 cells per 200 μl, the logarithm of the concentration of the CEM cells showed a good linear relationship with the fluorescence signal, the fluorescence intensity as a function of Tb3+-apt minus logarithm of concentration of the cells. The results were the average of four repetitive experiments, with error bars indicating the standard deviation (Fig. 3B). The linear equation was F = −680.2C + 5750.6 (F is the fluorescence intensity, C is Tb3+-apt minus logarithm of concentration of the cells), R2 = 0.9881, and the detection limit is 5 cells per ml. Compared with other reported fluorescence methods in Table 1, this method can significantly reduce the detection limit, suggesting a better sensitivity.
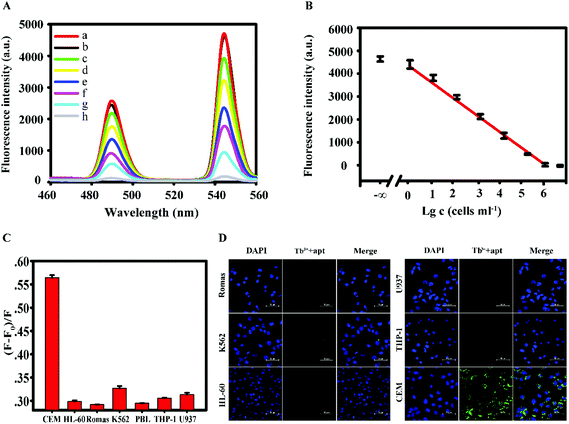 |
| Fig. 3 (A) Fluorescence emission spectra of the Tb3+-apt fluorescent aptasensor in the presence of different concentrations of CCRF-CEM cells (a. Tb3+-apt, b. 100 cells per 200 μl, c. 101 cells per 200 μl, d. 102 cells per 200 μl, e. 103 cells per 200 μl, f. 104 cells per 200 μl, g. 105 cells per 200 μl, h. 106 cells per 200 μl). (B) Linear relationship between the fluorescence intensity and the concentration of CCRF-CEM cells. (C) Specificity of the fluorescent aptasensor for CEM. The fluorescence intensity rate (F − F0)/F of the Tb3+-apt fluorescent aptasensor in the presence of CEM, HL-60, Ramos, K562, PBL, THP-1, and U937 cells, respectively (1 × 106 cells per 200 μl), where F and F0 are the fluorescence intensity without and with detection cells at 545 nm. Excitation: 290 nm. (D) Fluorescence micrographs of six different cells after mixing with Tb3+-apt. Nuclei were stained with DAPI. Scale bars indicate 50 μm. | |
Table 1 Comparison of analytical properties of CCRF-CEM cytosensors
Detection method |
Linear range |
Detection limit/mL |
Ref. |
Colorimetric |
3.30 × 103–2.69 × 103 |
214 |
42
|
Quartz crystal microbalance |
8.00 × 103–1.00 × 105 |
8000 |
43
|
Aptamer-conjugated magnetic beads |
1.00 × 104–1.50 × 105 |
8000 |
44
|
Flow cytometry |
7.50 × 103–6.25 × 105 |
750 |
45
|
Fluorescence |
5.00–5.00 × 106 |
5 |
This work |
Under the selected optimal conditions, the specificity of the fluorescence method was investigated. According to the results shown in Fig. 3C, the intensity of fluorescence of the CEM cells was significantly higher than that of other control cells. In addition, as shown in Fig. 3D, the probes bound well to the target cell CEM as detected using the confocal microscope, emitting green fluorescence, whereas the probes did not bind to other cells, as only blue nuclei could be seen. These experimental results show that this method has strong anti-interference and high specificity for CEM cells.
3.5. The cytotoxicity of the probe
In order to investigate whether the apt-Tb3+ probe is destructive to the sample during the assay, in vitro toxicity assessment was performed at the cellular level using the Cell Counting Kit-8. The CEM cells were co-cultured with different concentrations of probes, and the cell viability was measured after co-culture for a certain period. Fig. 4 shows that as the probe concentration increases (10 mM, 20 mM, 40 mM, 80 mM, 160 mM), the viability of the cells is reduced, but even at a maximum concentration of 160 mM, the probe is incubated with the CEM cells for 4 h; therefore, the vitality can be preserved above 90%. This result indicates that the probe has good biocompatibility with no toxicity to cells during a short period.
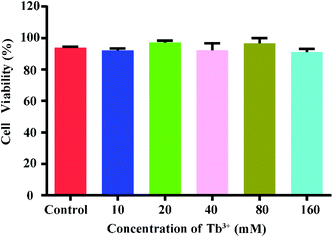 |
| Fig. 4 Relative viability of CCRF-CEM cells incubated with Tb3+-apt at different concentrations for 24 h. | |
3.6. Analysis of clinical samples
In order to evaluate the applicability of this method, 100 clinical samples were collected, of which 20 were from clinically diagnosed ALL patients, 60 were from non-acute lymphoblastic leukemia patients, and 20 were from normal healthy volunteers. Table 3 shows a specificity of 94% and a positive rate of 90%, while clinical sample specific information is shown in Table 2. Obviously, the ALL group is significantly different from the non-ALL group and the normal group as shown in Fig. 5. These results indicate that the fluorescence method can be applied for the detection of acute leukemia in blood samples of clinical patients.
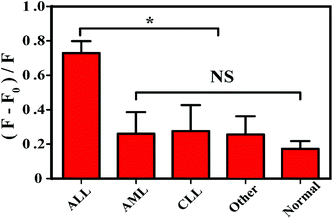 |
| Fig. 5 Specificity of the fluorescent aptasensor for clinical samples. The fluorescence intensity rate (F − F0)/F of the Tb3+-apt fluorescent aptasensor in the presence of ALL (acute lymphoblastic leukemia), AML (acute myelocytic leukemia), CLL (chronic lymphocytic leukemia), other (including myelodysplastic histiocytosis, Hodgkin's lymphoma, non-Hodgkin's lymphoma, thrombocytopenic purpura, iron deficiency anemia, the Mediterranean anemia), normal, where F and F0 are the fluorescence intensity without and with detection cells at 545 nm. Excitation: 290 nm. | |
Table 2 General clinical information
Number |
Gender |
Age (years) |
Sample |
Diagnosis |
|
|
|
Acute lymphoblastic leukemia patients (ALL) |
|
1 |
Female |
28 |
Peripheral blood/bone marrow |
T-ALL-L1 |
2 |
Female |
13 |
Peripheral blood |
T-ALL-L2 |
3 |
Male |
6 |
Peripheral blood/bone marrow |
T-ALL-L1 |
4 |
Male |
8 |
Peripheral blood/bone marrow |
T-ALL-L1 |
5 |
Female |
9 |
Peripheral blood/bone marrow |
T-ALL-L2 |
6 |
Male |
20 |
Peripheral blood/bone marrow |
T-ALL-L2 |
7 |
Male |
12 |
Peripheral blood/bone marrow |
T-ALL-L1 |
8 |
Male |
19 |
Peripheral blood/bone marrow |
T-ALL-L1 |
9 |
Male |
29 |
Peripheral blood/bone marrow |
T-ALL-L2 |
10 |
Female |
5 |
Peripheral blood/bone marrow |
T-ALL-L1 |
11 |
Female |
34 |
Peripheral blood/bone marrow |
T-ALL-L1 |
12 |
Male |
8 |
Peripheral blood/bone marrow |
T-ALL-L2 |
13 |
Male |
9 |
Peripheral blood/bone marrow |
T-ALL-L1 |
14 |
Female |
15 |
Peripheral blood/bone marrow |
B-ALL |
15 |
Female |
12 |
Peripheral blood/bone marrow |
T-ALL-L1 |
16 |
Male |
54 |
Peripheral blood/bone marrow |
T-ALL-L2 |
17 |
Male |
2 |
Peripheral blood/bone marrow |
T-ALL-L2 |
18 |
Male |
9 |
Peripheral blood/bone marrow |
T-ALL-L1 |
19 |
Male |
6 |
Peripheral blood/bone marrow |
T-ALL-L1 |
20 |
Female |
6 |
Peripheral blood/bone marrow |
T-ALL-L2 |
|
|
|
Acute myelocytic leukemia patients (AML) |
|
1 |
Male |
59 |
Peripheral blood/bone marrow |
AML-M1 |
2 |
Male |
33 |
Peripheral blood/bone marrow |
AML-M3 |
3 |
Female |
65 |
Peripheral blood/bone marrow |
AML-M0 |
4 |
Male |
45 |
Peripheral blood/bone marrow |
AML-M1 |
5 |
Female |
58 |
Peripheral blood/bone marrow |
AML-M1 |
6 |
Male |
13 |
Peripheral blood/bone marrow |
AML-M1 |
7 |
Male |
10 |
Peripheral blood/bone marrow |
AML-M1 |
8 |
Female |
5 |
Peripheral blood/bone marrow |
AML-M1 |
9 |
Female |
5 |
Peripheral blood/bone marrow |
AML-M3 |
10 |
Male |
8 |
Peripheral blood/bone marrow |
AML-M4 |
11 |
Male |
54 |
Bone marrow |
AML-M3 |
12 |
Male |
7 |
Peripheral blood/bone marrow |
AML-M3 |
13 |
Female |
31 |
Bone marrow |
AML-M3 |
14 |
Male |
21 |
Peripheral blood/bone marrow |
AML-M3 |
15 |
Female |
54 |
Peripheral blood/bone marrow |
AML-M1 |
16 |
Male |
23 |
Peripheral blood/bone marrow |
AML-M1 |
17 |
Female |
5 |
Peripheral blood/bone marrow |
AML-M1 |
18 |
Female |
18 |
Bone marrow |
AML-M3 |
19 |
Male |
17 |
Peripheral blood/bone marrow |
AML-M1 |
20 |
Male |
17 |
Peripheral blood/bone marrow |
AML-M4 |
|
|
|
Chronic lymphocytic leukemia (CLL) |
|
1 |
Male |
58 |
Peripheral blood/bone marrow |
B-CLL |
2 |
Male |
45 |
Peripheral blood/bone marrow |
B-CLL |
3 |
Male |
57 |
Peripheral blood/bone marrow |
B-CLL |
4 |
Female |
6 |
Peripheral blood/bone marrow |
B-CLL |
5 |
Female |
8 |
Bone marrow |
B-CLL |
6 |
Female |
9 |
Peripheral blood/bone marrow |
B-CLL |
7 |
Female |
12 |
Bone marrow |
B-CLL |
8 |
Female |
7 |
Peripheral blood |
B-CLL |
9 |
Male |
5 |
Peripheral blood/bone marrow |
B-CLL |
10 |
Male |
16 |
Peripheral blood/bone marrow |
B-CLL |
11 |
Male |
23 |
Peripheral blood/bone marrow |
T-CLL |
12 |
Male |
18 |
Bone marrow |
B-CLL |
13 |
Male |
17 |
Peripheral blood/bone marrow |
B-CLL |
14 |
Female |
25 |
Peripheral blood/bone marrow |
B-CLL |
15 |
Female |
29 |
Peripheral blood/bone marrow |
B-CLL |
16 |
Female |
5 |
Peripheral blood/bone marrow |
T-CLL |
17 |
Male |
6 |
Peripheral blood |
B-CLL |
18 |
Male |
6 |
Peripheral blood |
B-CLL |
19 |
Female |
8 |
Bone marrow |
B-CLL |
20 |
Female |
59 |
Peripheral blood/bone marrow |
B-CLL |
|
|
|
Other |
|
1 |
Male |
56 |
Peripheral blood/bone marrow |
Myelodysplastic histiocytosis |
2 |
Male |
34 |
Peripheral blood/bone marrow |
Myelodysplastic histiocytosis |
3 |
Female |
26 |
Peripheral blood/bone marrow |
Myelodysplastic histiocytosis |
4 |
Male |
21 |
Peripheral blood/bone marrow |
Myelodysplastic histiocytosis |
5 |
Male |
5 |
Peripheral blood/bone marrow |
Thrombocytopenic purpura |
6 |
Female |
14 |
Peripheral blood/bone marrow |
Thrombocytopenic purpura |
7 |
Male |
12 |
Peripheral blood/bone marrow |
Non-Hodgkin's lymphoma |
8 |
Male |
52 |
Peripheral blood/bone marrow |
Non-Hodgkin's lymphoma |
9 |
Female |
51 |
Peripheral blood/bone marrow |
Thrombocytopenic purpura |
10 |
Female |
25 |
Peripheral blood/bone marrow |
Iron deficiency anemia |
11 |
Female |
18 |
Peripheral blood/bone marrow |
Iron deficiency anemia |
12 |
Male |
15 |
Peripheral blood/bone marrow |
Iron deficiency anemia |
13 |
Female |
14 |
Peripheral blood/bone marrow |
The Mediterranean anemia |
14 |
Male |
16 |
Peripheral blood/bone marrow |
The Mediterranean anemia |
15 |
Male |
29 |
Peripheral blood/bone marrow |
The Mediterranean anemia |
16 |
Female |
38 |
Peripheral blood/bone marrow |
The Mediterranean anemia |
17 |
Male |
45 |
Peripheral blood/bone marrow |
The Mediterranean anemia |
18 |
Female |
56 |
Peripheral blood/bone marrow |
Hodgkin's lymphoma |
19 |
Male |
29 |
Peripheral blood/bone marrow |
Hodgkin's lymphoma |
20 |
Male |
37 |
Peripheral blood/bone marrow |
Hodgkin's lymphoma |
|
|
|
Healthy volunteers Normal |
|
1 |
Female |
18 |
Peripheral blood |
Normal |
2 |
Male |
20 |
Peripheral blood |
Normal |
3 |
Male |
25 |
Peripheral blood |
Normal |
4 |
Female |
24 |
Peripheral blood |
Normal |
5 |
Male |
24 |
Peripheral blood |
Normal |
6 |
Male |
48 |
Peripheral blood |
Normal |
7 |
Female |
45 |
Peripheral blood |
Normal |
8 |
Male |
34 |
Peripheral blood |
Normal |
9 |
Male |
35 |
Peripheral blood |
Normal |
10 |
Male |
28 |
Peripheral blood |
Normal |
11 |
Male |
28 |
Peripheral blood |
Normal |
12 |
Male |
27 |
Peripheral blood |
Normal |
13 |
Female |
26 |
Peripheral blood |
Normal |
14 |
Male |
26 |
Peripheral blood |
Normal |
15 |
Male |
26 |
Peripheral blood |
Normal |
16 |
Male |
27 |
Peripheral blood |
Normal |
17 |
Male |
25 |
Peripheral blood |
Normal |
18 |
Male |
25 |
Peripheral blood |
Normal |
19 |
Female |
24 |
Peripheral blood |
Normal |
20 |
Male |
24 |
Peripheral blood |
Normal |
Table 3 Comparison of clinical diagnosis rate and detection rate of this experimental method
Group |
Number of clinical diagnoses |
Number of diagnoses by this experimental method |
ALL, acute lymphoblastic leukemia. |
ALL group |
20 |
18 |
Non-ALL group and the normal group |
80 |
5 |
4. Conclusion
In this work, we successfully developed a non-labeled signal sensitized fluorescence method for the detection of leukemia based on the characteristics of nucleic acid aptamer sensitized rare earth Tb3+ fluorescence. Compared with known cancer cell assay methods, this strategy has some superior features. First, the strategy of detection is inexpensive and convenient because no labels are required. Second, the affinity, stability, and specificity of the aptamer bound by Tb3+ are greatly enhanced under optimal conditions. Lastly, it is applicable for many types of cancer cells that use different aptamers to identify probes. Therefore, this simple, rapid, sensitive, universal and specific cancer cell detection strategy provides a new approach for the detection of cancer cells by the label-free fluorescence method. Compared with the antigen–antibody sandwich technique, this method shortens the detection time, enabling rapid analysis and formulation of treatment plans for patients as it provides a detailed classification of leukemia in real-time samples.
Conflicts of interest
There are no conflicts of interest to declare.
Acknowledgements
This work was supported by the Programs for National Natural Scientific Foundation of China (No. 81430055); Changjiang Scholars and Innovative Research Team in University (No. IRT_15R13); Guangxi Science and Technology Base and Talent Special Project (No. AD17129003).
All experiments were performed in accordance with the Guidelines of Declaration of Helsinki Principles and approved by the Ethics Committee at Guangxi Medical University. Informed consents were obtained from human participants of this study.
References
- H. M. Meng, H. Liu, H. Kuai, R. Peng, L. Mo and X. B. Zhang, Chem. Soc. Rev., 2016, 45, 2583–2602 RSC.
- J. Munoz, N. Shah, K. Rezvani, C. Hosing, C. M. Bollard, B. Oran, A. Olson, U. Popat, J. Molldrem, I. K. McNiece and E. J. Shpall, Stem Cells Transl. Med., 2014, 3, 1435–1443 CrossRef PubMed.
- R. L. Siegel, S. A. Fedewa, K. D. Miller, A. Goding-Sauer, P. S. Pinheiro, D. Martinez-Tyson and A. Jemal, CA-Cancer J. Clin., 2015, 65, 457–480 CrossRef PubMed.
- S. C. Chan, W. L. Yau, W. Wang, D. K. Smith, F. S. Sheu and H. M. Chen, J. Pept. Sci., 1998, 4, 413–425 CrossRef CAS PubMed.
- S. Boddington, T. D. Henning, E. J. Sutton and H. E. Daldrup-Link, J. Visualized Exp., 2008, 13, 685 Search PubMed.
- S. Choi, J. Yu, S. A. Patel, Y. L. Tzeng and R. M. Dickson, Photochem. Photobiol. Sci., 2011, 10, 109–115 RSC.
- H. Shi, Z. Tang, Y. Kim, H. Nie, Y. F. Huang, X. He, K. Deng, K. Wang and W. Tan, Chem. – Asian J., 2010, 5, 2209–2213 CrossRef CAS PubMed.
- K. Pulford, N. Lecointe, K. Leroy-Viard, M. Jones, D. Mathieu-Mahul and D. Y. Mason, Blood, 1995, 85, 675–684 CAS.
- M. Doan, I. Vorobjev, P. Rees, A. Filby, O. Wolkenhauer, A. E. Goldfeld, J. Lieberman, N. Barteneva, A. E. Carpenter and H. Hennig, Trends Biotechnol., 2018, 36, 649–652 CrossRef CAS PubMed.
- G. Rymkiewicz, B. Grygalewicz, M. Chechlinska, K. Blachnio, Z. Bystydzienski, J. Romejko-Jarosinska, R. Woroniecka, M. Zajdel, K. Domanska-Czyz and D. Martin-Garcia, Mod. Pathol., 2018, 31, 732–743 CrossRef CAS PubMed.
- L. M. Neckers, W. K. Funkhouser, J. B. Trepel, J. Cossman and H. G. Gratzner, Exp. Cell Res., 1985, 156, 429–438 CrossRef CAS PubMed.
- I. B. Rozenvald, M. D. Richardson, L. Brock and R. L. Maiese, Arch. Pathol. Lab. Med., 2017, 141, 837–840 CrossRef CAS PubMed.
- E. A. Morgan, H. Yu, J. L. Pinkus and G. S. Pinkus, Am. J. Clin. Pathol., 2013, 139, 220–230 CrossRef PubMed.
- L. C. Ho, W. C. Wu, C. Y. Chang, H. H. Hsieh, C. H. Lee and H. T. Chang, Anal. Chem., 2015, 87, 4925–4932 CrossRef CAS PubMed.
- L. Yan, H. Shi, X. He, K. Wang, J. Tang, M. Chen, X. Ye, F. Xu and Y. Lei, Anal. Chem., 2014, 86, 9271–9277 CrossRef CAS PubMed.
- D. Shangguan, L. Meng, Z. C. Cao, Z. Xiao, X. Fang, Y. Li, D. Cardona, R. P. Witek, C. Liu and W. Tan, Anal. Chem., 2008, 80, 721–728 CrossRef CAS PubMed.
- M. Ye, J. Hu, M. Peng, J. Liu, J. Liu, H. Liu, X. Zhao and W. Tan, Int. J. Mol. Sci., 2012, 13, 3341–3353 CrossRef CAS PubMed.
- A. Ganji, A. Varasteh and M. Sankian, J. Drug Targeting, 2016, 24, 1–12 CrossRef CAS PubMed.
- Q. Shen, C. Peng, Y. Zhan, L. Fan, M. Wang, Q. Zhou, J. Liu, X. Lv, Q. Tang, J. Li, X. Huang and J. Xia, Int. J. Nanomed., 2016, 11, 2133–2146 CrossRef CAS PubMed.
- B. Mondal, S. Ramlal, P. S. Lavu, B. N and J. Kingston, Front. Microbiol., 2018, 9, 179 CrossRef PubMed.
- M. Mascini, I. Palchetti and S. Tombelli, Angew. Chem., Int. Ed., 2012, 51, 1316–1332 CrossRef CAS PubMed.
- S. Philippou, N. P. Mastroyiannopoulos, N. Makrides, C. W. Lederer, M. Kleanthous and L. A. Phylactou, Mol. Ther.–Nucleic Acids, 2018, 10, 199–214 CrossRef CAS PubMed.
- X. Li, Y. Peng, Y. Chai, R. Yuan and Y. Xiang, Chem. Commun., 2016, 52, 3673–3676 RSC.
- Y. Tan, X. Wei, Y. Zhang, P. Wang, B. Qiu, L. Guo, Z. Lin and H. H. Yang, Anal. Chem., 2015, 87, 11826–11831 CrossRef CAS PubMed.
- J. Ni, W. Yang, Q. Wang, F. Luo, L. Guo, B. Qiu, Z. Lin and H. Yang, Biosens. Bioelectron., 2018, 105, 182–187 CrossRef CAS PubMed.
- Y. Wang, Z. Li, H. Li, M. Vuki, D. Xu and H. Y. Chen, Biosens. Bioelectron., 2012, 32, 76–81 CrossRef CAS PubMed.
- H. Li, M. Wang, C. Wang, W. Li, W. Qiang and D. Xu, Anal. Chem., 2013, 85, 4492–4499 CrossRef CAS PubMed.
- S. Feng, C. Chen, W. Wang and L. Que, Biosens. Bioelectron., 2018, 105, 36–41 CrossRef CAS PubMed.
- C. Chen, Z. Yang and X. Tang, Med. Res., 2018, 38, 829–869 Search PubMed.
- Y. Yao, X. Wang, W. Duan and F. Li, Analyst, 2018, 143, 709 RSC.
- P. Mallikaratchy, Z. Tang, S. Kwame, L. Meng, D. Shangguan and W. Tan, Mol. Cell. Proteomics, 2007, 6, 2230–2238 CrossRef CAS PubMed.
- Y. X. Zou, S. Huang, Y. Liao, X. Zhu, Y. Chen, L. Chen, F. Liu, X. Hu, H. Tu and L. Zhang, Chem. Sci., 2018, 9, 2842–2849 RSC.
- D. Shangguan, Y. Li, Z. Tang, Z. C. Cao, H. W. Chen, P. Mallikaratchy, K. Sefah, C. J. Yang and W. Tan, Proc. Natl. Acad. Sci. U. S. A., 2006, 103, 11838–11843 CrossRef CAS PubMed.
- S. M. Taghdisi, K. Abnous, F. Mosaffa and J. Behravan, J. Drug Targeting, 2010, 18, 277–281 CrossRef CAS PubMed.
- N. M. Danesh, P. Lavaee, M. Ramezani, K. Abnous and S. M. Taghdisi, Int. J.
Pharm., 2015, 489, 311–317 CrossRef CAS PubMed.
- A. Fang, H. Chen, H. Li, M. Liu, Y. Zhang and S. Yao, Biosens. Bioelectron., 2017, 87, 545–551 CrossRef CAS PubMed.
- J. Yuan and G. Wang, J. Fluoresc., 2005, 15, 559–568 CrossRef CAS PubMed.
- Z. Ye, J. Chen, G. Wang and J. Yuan, Anal. Chem., 2011, 83, 4163–4169 CrossRef CAS PubMed.
- K. L. Fu and C. Turro, J. Am. Chem. Soc., 2009, 121, 1–7 CrossRef.
- M. K. Johansson, R. M. Cook, J. Xu and K. N. Raymond, J. Am. Chem. Soc., 2004, 126, 16451–16455 CrossRef CAS PubMed.
- W. Yueteng, L. Ru, W. Yaling, Z. Yuliang, C. Zhifang and G. Xueyun, Analyst, 2013, 138, 2302–2307 RSC.
- H. Shi, D. Li, F. Xu, X. He, K. Wang, X. Ye, J. Tang and C. He, Analyst, 2014, 139, 4181–4184 RSC.
- Y. Pan, M. Guo, Z. Nie, Y. Huang, C. Pan, K. Zeng, Y. Zhang and S. Yao, Biosens. Bioelectron., 2010, 25, 1609–1614 CrossRef CAS PubMed.
- J. Zhu, T. Nguyen, R. Pei, M. Stojanovic and Q. Lin, Lab Chip, 2012, 12, 3504–3513 RSC.
- J. Yin, X. He, K. Wang, F. Xu, J. Shangguan, D. He and H. Shi, Anal. Chem., 2013, 85, 12011–12019 CrossRef CAS PubMed.
Footnote |
† These authors contributed equally to this work. |
|
This journal is © The Royal Society of Chemistry 2019 |
Click here to see how this site uses Cookies. View our privacy policy here.