DOI:
10.1039/C8AY02013B
(Paper)
Anal. Methods, 2019,
11, 97-104
β-Cyclodextrin protected gold nanoparticle based cotton swabs as an effective candidate for specific sensing of trace levels of cyanide†
Received
13th September 2018
, Accepted 20th November 2018
First published on 20th November 2018
Abstract
In this paper, a rapid colorimetric and easy visual determination of cyanide ions (CN− ions) in water, based on gold nanoparticles (AuNPs) stabilized with β-cyclodextrin (β-CD), is described. Optical detection of CN− ions is carried out using β-CD-AuNPs by tracking the changes in the surface plasmon resonance band and colorimetric nature of the β-CD AuNPs. This colorimetric nanosensor easily and with ultra-sensitivity identifies the CN− ions because of their capacity to etch the surface of the β-CD AuNPs and form a stable water soluble Au–CN complex. Under experimental conditions, the colorimetric assay demonstrated a steady response for CN− ions over a concentration ranges from 4.50 × 10−6 to 99.00 × 10−6 mol dm−3 with R = 0.994. The lowest limit of detection of this inexpensive, visual with the naked eye, approach is 93.00 × 10−9 mol dm−3, which is under the tolerance limit value (1.90 × 10−6 mol dm−3) set by the World Health Organization for drinking water. The specificity of the β-CD-AuNPs based optical platform for the detection of CN− ions is superb when evaluated with various potential interfering anions. Furthermore, the proposed β-CD-AuNPs nanoprobe is effectively applied to quantify the CN− ions in tap and drinking water samples. For convenient use, a very stable cotton swab containing the β-CD-AuNPs has been designed and it revealed good sensitivity against CN− ions in the environment using the naked eye.
Introduction
In the 21st century, environmental pollution is one of the major dangerous subjects facing the Earth. In particular, water contamination is a serious issue because it is one of the most common categories of effluent that can affect numerous living organisms and ecological networks.1,2 Among a variety of sources of water contamination, the cyanide anions (CN− ions) are more perceptible because of their large occurrence in the environment, poisonous characteristics and highly dangerous nature.3 However, CN− ions are usually used in some types of industry, mostly in metallurgy, organic polymer production and electroplating4 which increases the possibility of its pollution of water in the environment. For mammals, CN− ions are extremely poisonous because of their effective binding with the ferric iron cations (Fe3+) in hemoglobin, then it is taken into the tissues in the body where it binds with cytochrome a3, thus stopping its oxygen transfer role.5 Furthermore, CN− ion poisoning is accompanied by convulsions, nausea, followed by loss of consciousness, and finally, death. In accordance with the World Health Organization (WHO) criteria, the highest tolerable amount of CN− ions is 1.90 × 10−6 mol dm−3 in drinking water.6,7 Therefore, it is necessary to build a low-cost nanosensor capable of working simply, efficiently, and quickly, to quantify CN− ion contamination in different environmental water resources to keep the environment and the public safe.
The severe deleterious nature of CN− ions to physiology, and the progress of ecological concern due to its extensive industrial usage has lead to significant investigations of, and growth of the number of, techniques available for the determination of CN− ions. To date, a variety of systematic analytical methodologies have been used for the quantification of CN− ions, including electrochemical,8 chromatographic,9 ratiometric detection,10 fluorimetric,11,12 chemiluminescence,13 Raman spectroscopic,14 surface enhanced Raman spectroscopy15 and spectrophotometric/colorimetric methods.15–17 Even though these analytical approaches (except optical methods) are ultra sensitive and have good specificity, unfortunately, they have a high cost, need qualified operators, and are non-portable and time-consuming to operate. Therefore, simple, fast and convenient analytical methodologies for the determination of CN− ions are urgently required. To overcome these issues, colorimetric methods are extremely useful because they require little effort to use, are low cost and they give fast results. In addition, naked eye visual-based sensing analysis is done simply by examination with the naked eye/absorption spectrophotometry, without requiring sophisticated apparatus.18,19 In modern times, metal nanostructural objects based on a visual approach are very interesting and are normally based on the alteration of optical characteristics such as: aggregation, the surface chemical reaction, size, and shape transition accompanied by a distinctive colour change.20 Due to the outstanding visual properties of gold (Au) and silver (Ag) nanostructures are the most appropriate for colorimetric applications.
Gold nanoparticles (AuNPs) have become one of the accepted nanomaterials for use in the detection of analytes in the last decade. AuNPs are widely used in numerous areas, specifically in analytical chemistry, owing to the unique electrical, optical, physiochemical and catalytic properties which are easily managed by changing its composition, surrounding environment and morphology.21 Furthermore, outstanding biocompatibility, easy preparation, extensive surface conjugation chemistry with the smart surface plasmon resonance (SPR) of AuNPs make them an ideal candidate for the growth of nanoprobes for several analytes with specifically required features.21–24 The SPR band of AuNPs is positioned in the visible area and gives bright colours. Furthermore, the SPR band of AuNPs is mainly influenced by interparticle distance, morphological transition and local environment.25 For example, the greatest SPR band is associated with the size of the AuNPs because of the increase of the AuNPs size caused by reduction of the distinct energy gaps.26 Having an enormously large molar extinction coefficient and a sturdy interparticle distance reliant SPR band, AuNPs are perfect for trouble-free, specific, highly sensitive and low-cost naked eye visual approaches for the detection of CN− ions in 100% aqueous solution. Additionally, the delivery of AuNP-based nanosensors are easily monitored using ultraviolet visible (UV-vis) spectrophotometry or the naked eye.27 CN− ions are able to be caught by suspended Au metal in the presence of oxygen gas leading to the creation of a water soluble Au–CN complex.28 Based on this chemical reaction, several research groups demonstrated that various molecules based on functionalised AuNPs can be applied to the detection of CN− ions.11–13,15 In addition, AuNPs can be applied in various environmental and biomedical applications.29,30
In this research, a simple colorimetric approach was attempted to construct an ultrasensitive detector for CN− ions with excellent selectivity. To attain this goal, a useful nanoprobe was created by disturbing the spectral and colorimetric properties of AuNPs with CN− ions. Among the numerous surface functionalizing agents applied previously in the synthesis of AuNPs, in this work an unmodified β-cyclodextrin (β-CD) molecule was chosen as a stabilizing and reducing ligand for the preparation of the AuNPs. The toroid structure of β-CD has an exclusive inner cavity, which is capable of acting as both a capping and reducing agent because of the method of chemical reduction used in the preparation of the AuNPs.30–32 During the reduction of the Au salt to the analogous AuNPs, the β-CD moieties are oxidized. The oxidation product of capping ligand such as β-CD molecules were fabricated using the carboxylic acid group acting as a negative surface charge present intense, covering the outsides of the AuNPs surface and supplying stabilization, which are responsible for preventing the aggregation of the NPs.33 When the β-CD stabilized AuNPs (β-CD AuNPs) are in the presence of CN− ions, the SPR band of β-CD AuNPs is decreased. The colour of the β-CD AuNPs also altered from wine red to colourless, demonstrating that β-CD AuNPs surfaces had been etched and that a stable water soluble Au–CN complex had formed after the addition of the CN− ions. Due to the etching mechanism, the β-CD AuNPs nanoprobe was highly sensitive to CN− ions. The selectivity of this sensing colorimetric assay was outstanding, as various potentially interfering common anions, did not obstruct the determination of the CN− ions using the colorimetric nature of the β-CD AuNPs. Furthermore, the colorimetric assay developed was successfully used to quantify the CN− ions in drinking water and tap water samples. In addition, the proposed optical strategy was used to create a cotton-based strip as an indicator for naked eye determination of CN− ions for portable usage.
Experimental
Chemicals
All the chemicals were of at least analytical reagent grade. Tetrachloroauric acid (HAuCl4) was purchased from Sigma-Aldrich, USA. Sodium hydroxide (NaOH) and β-CD were obtained from S D Fine-Chem, India. Sodium cyanide was purchased from Loba Chemie Pvt. Ltd., India. All the reagents were used as obtained without further purification. All aqueous solutions used in the analysis were prepared with doubly distilled water. A newly prepared solution of aqua regia (HNO3
:
HCl, 1
:
3) was used for washing the glassware which was rinsed thoroughly with doubly distilled water. Then, the glassware was dried in hot air oven at 130 °C.
Preparation of β-CD functionalised gold nanoparticles (β-CD AuNPs)
The β-CD stabilized AuNPs were prepared using an earlier method.34 In brief, a 7.08 × 10−3 mol dm−3 concentration of clear β-CD solution (48.5 mL) was mixed with a 10.00 × 10−3 mol dm−3 concentration of HAuCl4 solution (1 mL) and this solution was stirred well. After 3 min, a 1.00 mol dm−3 concentration of NaOH (0.5 mL) was added to the reaction mixture so that the pH of the solution reached ∼10–12. Next, the reaction mixture was stirred vigorously and heated on a water bath. Finally, the reaction mixture transformed from colourless to a wine red colour, which indicated that the β-CD AuNPs had formed.
Instrumentation
The UV-vis absorption spectral experiments were carried out using a double beam UV-vis spectrophotometer (V-630, JASCO, Japan) at ambient temperature. Fourier transform infrared (FT-IR) spectral measurements of solid β-CD and β-CD AuNPs were verified using a spectrophotometer (FT-IR-V 400 series, JASCO, Japan). Analysis of the β-CD capped AuNPs was carried out using high resolution transmission electron microscopy (HR-TEM, JEM 2100, Jeol) and the samples were prepared by the insertion of few drops of freshly prepared β-CD AuNPs on carbon pre-coated copper grids and then evaporating the water under vacuum. The zeta potential value and hydrodynamic diameter of the β-CD AuNPs with and without CN− ions in solution were measured using dynamic light scattering (DLS, Zetasizer Nano ZS, Malvern Panalytical) with a 633 nm helium–neon laser.
Detection procedure for CN− ions using β-CD AuNPs
In 5 mL standard measuring flask, an appropriate amount of CN− ions were mixed with 5 mL of β-CD AuNPs. This solution was shaken well and left to stand for 10 min for the mixture to equilibrate. After 10 min, 2.5 mL of the reaction mixture was transferred to a quartz cuvette and the absorption spectra were measured against a blank in the range from 800 to 300 nm. For the naked eye visual determination, an optimal amount of various anions were suspended in the β-CD AuNPs colloidal dispersion, shaken well and left for 10 min for the mixture to equilibrate and the colour changes were visualized using the naked eye.
Real sample analysis
The convenient use of the resultant β-CD AuNPs colloidal solution in real samples analysis was assessed by using the method for the detection of CN− ions in tap and drinking water. Real water samples were taken from the tap and drinking water on the Bharathiar University campus, India and used as received. A known amount of CN− ions were mixed into the real water samples and the CN− ion concentration in spiked real water samples were determined using β-CD AuNPs. Then, the optical changes of the β-CD AuNPs were monitored using a standard addition procedure.
Cotton swab based colorimetric strip assay
For portable use, ear cleaning buds (cotton swabs) were used for the colorimetric determination of CN− ions. Cotton swabs were obtained from a local shop, in Coimbatore (India) and used as received. A cotton swab was soaked with β-CD AuNPs and air dried. Then, a β-CD AuNP coated cotton swab based strip was further soaked with a 99.00 × 10−6 mol dm−3 solution of CN− ions. The colour change of the cotton swab based strip was easily visualized using the naked eye.
Results and discussion
Absorption, FT-IR spectral and zeta potential characterization of β-CD AuNPs
The UV-vis absorption tool is an easy technique used to distinguish the metal nanoparticles, mostly Au and AgNPs because it demonstrated a solid SPR band in the visible region.35,36 A surface plasmon is a precise kind of plasma oscillation happening at lesser energies than that at a bulk plasmon, which occurs when light is combined to the consistent oscillation of free electrons at the surface of a conductor. This constant resonant oscillation of conduction electrons is described as an SPR band.35–38 The UV-vis absorption spectra of β-CD AuNPs are displayed in Fig. S1 (ESI†). As shown in Fig. S1 (ESI†), the resultant β-CD AuNPs exhibited a characteristic SPR band for such NPs, centred at 521 nm, and this illustrates the successful formation of β-CD AuNPs. Furthermore, β-CD AuNPs exhibit wine red colour (inset of Fig. S1, ESI†). Furthermore, the stability of the β-CD AuNPs was checked using absorption spectral measurements and the corresponding results are given in Fig. S2 (ESI†). As shown in Fig. S2 (ESI†), comparing the SPR band of a newly synthesized β-CD AuNPs, with ones obtained for an aged colloidal dispersion which was monitored for three months, no obvious SPR band alterations were noted. Furthermore, the wine red colour of the β-CD AuNPs colloidal solution remained identical (inset of Fig. S2, ESI†), which clearly revealed that the β-CD AuNPs were extremely stable for three months.
To determine the probable interaction between β-CD and AuNPs, FT-IR spectral studies of β-CD alone and the β-CD AuNPs were carried out and compared. The FT-IR spectra of β-CD alone and the β-CD AuNPs are presented in Fig. S3 (ESI†). As illustrated in Fig. S3 (ESI†), the β-CD AuNPs show two IR stretching frequencies at about 1404 and 1646 cm−1 which were attributed to the COO− groups acquired under this synthetic condition. In this research, the –OH groups of the β-CD act as the reducing agent for the reduction from Au3+ to Au0 and the β-CD molecules were self-oxidized into carboxylic acid.The carboxylic acid groups containing β-CD molecules would created negative surface charge and covering outer surface of the AuNPs, which are responsible for preventing aggregation and supplying stabilization of AuNPs.31,32 Almost all the IR frequencies of the spectra for β-CD alone and β-CD AuNPs were identical, which revealed that β-CD is present as an important part of the synthesised AuNPs. The obtained FT-IR results were consistent with those of the earlier reported β-CD stabilized metal NPs.34,39,40 To further confirm the negative surface of the β-CD AuNPs, zeta potential analysis was used and the analogous zeta potential data is given in Fig. S4 (ESI†). From Fig. S4 (ESI†), the zeta potential value of the β-CD AuNPs was found as −33.78 mV, revealing good stability of the AuNPs. These results were consistent with the results of the FT-IR spectral studies.
Sensitivity of the colorimetric probe
The majority of the techniques using metal NPs as nanosensors are found to be used for monitoring the changes in the SPR band. These changes are caused by the morphological transition of the surface of the metal NPs being stimulated by the biologically important and toxic analytes.35,37,40 To evaluate the sensitivity of the present nanoprobe towards CN− ions, absorption titration experiments of the β-CD AuNPs in the absence and presence of various amounts of CN− ions were used and the observed, typical equilibrium process is shown in Fig. 1. As shown in Fig. 1, as the concentration of the CN− ions is increased, the SPR band of the β-CD AuNPs regularly decreased. It was proposed that depending upon the CN− ion concentrations, the morphological characteristics of the β-CD AuNPs were gradually changed. The considerable reduction in the SPR band intensity (Fig. 1) is because of the etching process involved between the CN− ions and β-CD AuNPs. This observation revealed that the CN− ions were simply etching on the surface of β-CD AuNPs and this is the reason for the alteration in the SPR band of the β-CD AuNPs. To confirm that the CN− ions only interacted with the β-CD AuNPs, the SPR band of the β-CD AuNPs was investigated by increasing the addition of a known amount of water and the results are given in Fig. S5 (ESI†). It can be observed from Fig. S5 (ESI†), that there was no major alteration in the SPR band intensity and absorption maxima were noted which clearly indicates that the changes in the SPR band wavelength were only because of the etching of the surface of the β-CD AuNPs with CN− ions.
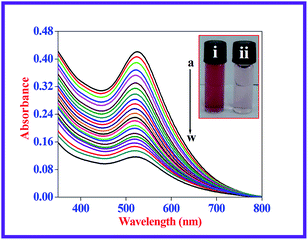 |
| Fig. 1 Absorption spectral responses of β-CD AuNPs in the absence and presence of different amounts of CN− ions. [CN− ions]: (a) 0.00, (b) 4.50 × 10−6, (c) 9.00 × 10−6, (d) 13.50 × 10−6, (e) 18.00 × 10−6, (f) 22.50 × 10−6, (g) 27.00 × 10−6, (h) 31.50 × 10−6, (i) 36.00 × 10−6 (j) 40.50 × 10−6 (k) 45.00 × 10−6 (l) 49.50 × 10−6 (m) 54.00 × 10−6 (n) 58.50 × 10−6 (o) 63.00 × 10−6 (p) 67.50 × 10−6 (q) 72.00 × 10−6 (r) 76.50 × 10−6 (s) 81.00 × 10−6 (t) 85.50 × 10−6 (u) 90.00 × 10−6, (v) 94.50 × 10−6 and (w) 99.00 × 10−6 mol dm−3. Inset: β-CD AuNPs before (i) and after (ii) the addition of 99.00 × 10−6 mol dm−3 of CN− ions. | |
One of the simplest techniques to determine the analyte is by the naked eye because it does not necessitate use of any instruments.37,40 In this investigation, CN− ions were detected using the naked eye approach by directly mixing the β-CD AuNPs with the CN− ions. The photographic images of the β-CD AuNPs in the absence and presence of CN− ions are shown in the inset of Fig. 1. The colour of the β-CD AuNPs instantly changes from wine red to colourless with the addition of 99.00 × 10−6 mol dm−3 CN− ions, which is effortlessly visualized using the naked eye. In addition, the colorimetric response of β-CD AuNPs with various concentrations of CN− ions was also further tested and the resultant photograph is displayed in Fig. 2. As increasing concentrations of the CN− ions were added to the β-CD AuNPs, the colour of the β-CD AuNPs steadily altered from wine red to colourless because of the etching of the β-CD AuNPs with the CN− ions (Fig. 2a). The noticeable spectral and colorimetric changes of β-CD AuNPs in the presence of CN− ions owing to the stable water soluble complex formation of CN− ions is shown by the chemical reaction shown in Scheme 1. Similar types of optical response of AuNPs with CN− ions have been previously reported.12,15,16,41–44
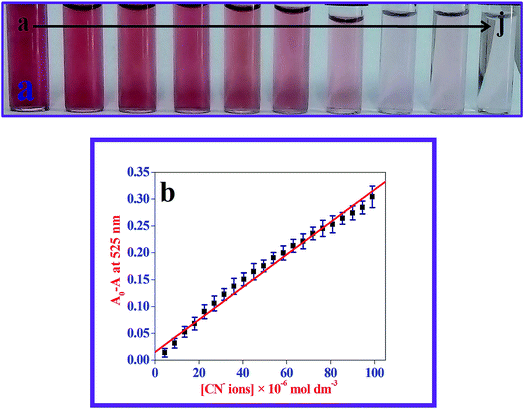 |
| Fig. 2 (a) Colorimetric changes of β-CD AuNPs before and after the addition of CN− ions. [CN− ions]: (a) 0.00, (b) 11.00 × 10−6, (c) 22.00 × 10−6, (d) 33.00 × 10−6, (e) 44.00 × 10−6, (f) 55.00 × 10−6, (g) 66.00 × 10−6, (h) 77.00 × 10−6, (i) 88.00 × 10−6 and (j) 99.00 × 10−6 mol dm−3 and (b) corresponding calibration curve for the detection of CN− ions based on the SPR band changes. | |
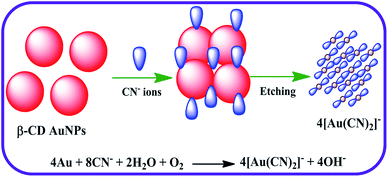 |
| Scheme 1 Schematic illustration of the interaction between CN− ions and β-CD AuNPs. | |
Relative SPR band absorption intensity changes were plotted against different amounts of CN− ions and are displayed in Fig. 2b. It can be seen from Fig. 2b that there was an excellent linear dependence of relative SPR band absorption intensity changes on CN− ion concentrations ranges from 4.50 × 10−6 mol dm−3 to 99.00 × 10−6 mol dm−3 with R = 0.994. Relative standard deviation (RSD) was less than 3% for five repeated experiments, implying that there was good reproducibility of the present optical approach. The lowest limit of detection (LOD) was estimated from the following expression: LOD = 3 × standard deviation (σ)/slope of the calibration (S). The LOD value of the CN− ions was calculated to be: 93.00 × 10−9 mol dm−3, which was much less than the highest level of CN− ions (1.90 × 10−6 mol dm−3) in drinking water allowed by WHO. This indicated that the present β-CD AuNPs based, naked eye visual approach is ultrasensitive and sufficient to determine the CN− ion concentration in drinking water. The LOD value obtained using the present method and other different naked eye visual approaches for the quantification of CN− ions are listed in Table S1 (ESI†).11–13,16,41–48 Compared with the previously reported approaches, the β-CD AuNP based colorimetric assay achieved a reasonable LOD value. In comparison, use of the present naked eye visual approach shows that it is fairly quick and easy to use to determine CN− ions.
The effects on the β-CD AuNPs morphological characteristics by the CN− ions were further described using HR-TEM and DLS measurements. HR-TEM images of β-CD AuNPs alone and effects on β-CD AuNPs by CN− ions are shown in Fig. 3. Before the addition of CN− ions, the β-CD AuNPs were mono dispersed, roughly spherical and had an average diameter of around 15 ± 2 nm (Fig. 3a). As shown in Fig. 3b, the average diameter of the AuNPs was obviously reduced from 15 ± 2 nm to 9 ± 3 nm upon the addition of the CN− ions. To confirm this hypothesis, DLS measurements were recorded for the β-CD AuNPs with and without addition of CN− ions solution and these are shown in Fig. S6 (ESI†). In the absence of CN− ions, the β-CD AuNPs have a mean hydrodynamic diameter of about ∼25 nm, which sustained the stability and size of the β-CD AuNPs (Fig. S6a, ESI†). After the addition of CN− ions, the average diameter was reduced to ∼13 nm, which was in good agreement with the HR-TEM images. The HR-TEM images combined with the DLS results proved that the CN− ions were successfully etching the AuNPs surface, in the presence of oxygen, which directed the alteration of the morphological properties, which influenced the optical colour alterations and assessable spectral deviation.12,15 These results were in good agreement with those previously reported in the literature.12
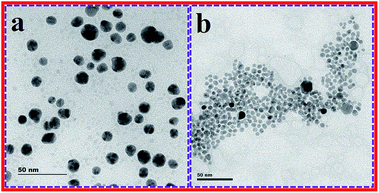 |
| Fig. 3 HR-TEM images of β-CD AuNPs alone (a) and β-CD AuNPs with a 50.00 × 10−6 mol dm−3 concentration of CN− ions (b). | |
Selectivity of the optical and colorimetric assay
For use as an outstanding naked eye visual nanoprobe, high specificity is a requirement. Considering the prospects of using the β-CD AuNPs based colorimetric nanosensor strategy in practical applications, the colorimetric specificity of the β-CD AuNPs nanoprobe toward CN− ions (99.00 × 10−6 mol dm−3) was investigated using other potentially interfering anions (Br−, Cl−, ClO4−, CO3−, C2O42−, F−, I−, NO2−, NO3−, PO4−, S2−, SO32−, SO42−, and SCN−, each at a concentration of 500.00 × 10−6 mol dm−3). A photographic image of the β-CD AuNPs colloidal solutions in the presence of a concentration of 99.00 × 10−6 mol dm−3 CN− ions and after the addition of a concentration of 500.00 × 10−6 mol dm−3 of the various interferant anions were taken and is displayed in Fig. 4a. The colorimetric nature of the β-CD AuNPs was changed only upon the mixing of the 99.00 × 10−6 mol dm−3 CN− ions whereas β-CD AuNPs in the presence of different potential interfering anion solutions such as Br−, Cl−, ClO4−, CO3−, C2O42−, F−, I−, NO2−, NO3−, PO4−, S2−, SO32−, SO42−, and SCN−, did not display any colour change, which was easily monitored by the naked eye. The observed result clearly indicates that the β-CD AuNPs based nanoprobe is highly colorimetric specific for CN− ions. In addition, to determine whether the colorimetric probe was extremely specific to CN− ions, the SPR band changes of the β-CD AuNPs with the addition of various common anions such as Br−, Cl−, ClO4−, CO3−, C2O42−, F−, I−, NO2−, NO3−, PO4−, S2−, SO32−, SO42−, and SCN−, were also monitored and the results are displayed in Fig. 4b. As shown in Fig. 4b, the collected absorption spectral data revealed that the proposed method was highly specific for the determination of CN− ions. This high specificity towards CN− ions is generally because of the ability of the CN− ions to create an Au–CN bond, which permits the CN− ions to etch the β-CD AuNPs surface, leading to aggregation. Alternatively, various general anions should be resisted by the negatively charged β-CD AuNPs, and no spectral and colorimetric changes were detected with the various potentially interfering anions. Because of the non-existence of the etching process, no changes in SPR band and colorimetric nature of β-CD AuNPs were observed after the addition of common anions. A similar type of AuNPs based detection of CN− ions was previously reported.12,41
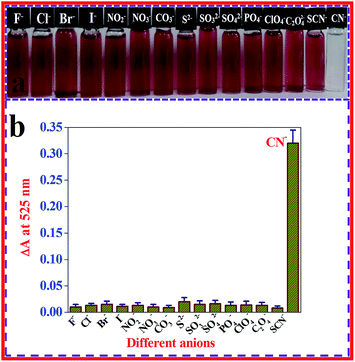 |
| Fig. 4 Colorimetric (a) and SPR band changes (b) of β-CD AuNPs after the addition of a concentration of 99.00 × 10−6 mol dm−3 of CN− ions and a 5-fold higher concentration of various anions. | |
Analytical utility of the present method
The use of the β-CD AuNPs in real water sample investigations were tested using the quantification of CN− ions in drinking and tap water samples. For the β-CD AuNPs in the presence of real water samples, CN− ions were not identified in the collected real water samples. To corroborate the strength of the results, known amounts of CN− ions were spiked into the real water samples and quantified using a standard addition procedure. The CN− ion spiked water samples were mixed to interact with the β-CD AuNPs. The average SPR band intensity of the β-CD AuNPs with CN− ions in real water samples were compared with those values obtained during the experimental change and the recoveries (%) were considered for every case. Furthermore, the real sampling analyses were replicated at least three times and the mean value obtained is noted as the result (n = 3). The real sample analysis results were extremely close to the experimental results with deionized water, which clearly shows that the β-CD AuNPs based colorimetric assay works well as a systematic methodology for the identification of CN− ions in real water samples (Table 1).
Table 1 Detection of spiked CN− ions in tap and drinkable watersa
Real samples |
CN− ions added × 10−6 mol dm−3 |
CN− ions estimated × 10−6 mol dm−3 |
Recovery (%) |
RSD (%), (n = 3) |
RSD: relative standard deviation.
|
Drinking water |
30.00 |
29.71 |
99.03 |
1.83 |
60.00 |
59.16 |
98.60 |
2.29 |
90.00 |
88.97 |
98.86 |
3.11 |
Tap water |
30.00 |
29.37 |
97.90 |
2.48 |
60.00 |
59.42 |
99.03 |
2.09 |
90.00 |
89.33 |
99.26 |
1.64 |
To convert the analytical investigation outcomes for use in daily life, a β-CD AuNPs coated cotton swab was designed as a strip, for the visual detection of CN− ions. After coating the β-CD AuNPs onto the cotton swab it becomes wine red in colour [Fig. 5a(i)]. Furthermore, the β-CD AuNPs coated cotton swab was characterized using field effect-scanning electron microscopy (FE-SEM) and energy dispersive X-ray spectroscopy (EDX) measurements and the results are shown in Fig. 5b and S7 (ESI†). Both FE-SEM and EDX measurements clearly show that AuNPs were embedded in the cotton swab [Fig. 5b (red circles) and Fig. S7 (ESI†)]. The β-CD AuNPs coated cotton swab was used for detection of CN− ions based on the realistic colorimetric approach. When AuNPs surrounded the cotton swab was soaked in the solution with a concentration of 99.00 × 10−6 mol dm−3 CN− ions, the cotton swab colour changed from wine red to colourless within 15 min, which indicates that a highly sensitive identification of CN− ions. This colorimetric change was easily monitored by naked eye [Fig. 5a(ii)]. Furthermore, the AuNPs coated cotton swab was highly stable for more than 20 days and sensing nature remains unchanged. These interpretations show that the cotton swab based test strip can be effectively used for the colorimetric identification of CN− ions.
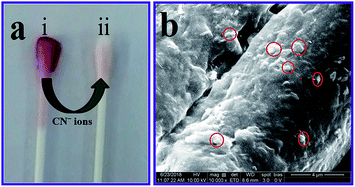 |
| Fig. 5 (a) β-CD AuNPs coated cotton swab (i), reaction with a concentration of 99.00 × 10−6 mol dm−3 of CN− ions changes the colour from wine red to colourless (ii), and (b) FE-SEM image clearly showing that the AuNPs were embedded in the cotton swab (red circles). | |
Conclusions
In summary, CN− ions are a lethal toxic poison to humanity. So a quick, specific and highly sensitive assay for the identification of CN− ions from natural water samples is particularly vital for the protection of human life. Focused by these necessities, an easy, trouble-free and fast colorimetric assay is proposed for the determination of CN− ions. The β-CD stabilized AuNPs nanoprobe was synthesized and applied as a colorimetric nanoprobe for the detection of CN− ions. The colorimetric nature and SPR band intensity of the β-CD AuNPs were reduced drastically after the addition of the CN− ions. Upon the addition of the CN− ions to the β-CD AuNPs, the CN− ions successfully etch the AuNPs surface in the presence of oxygen, which also causes the alteration of the morphological properties, influences the optical colour alterations and the spectral deviation. Such an alteration is openly associated with the concentration of the CN− ions and is able to operate for the highly sensitive and selective determination of CN− ions with an LOD value of 93.00 × 10−9 mol dm−3, which is much less than the highest level of CN− ions (1.90 × 10−6 mol dm−3) in drinking water allowed by the WHO. In addition, a β-CD AuNPs coated cotton swab based test strip was designed which can be applied as a colorimetric indicator of the CN− ions. These outstanding features create the present colorimetric assay which is appropriate for the reliable and rapid on-site determination of the concentration of CN− ions in real water samples, which is valuable for direct assessment of unpredicted danger and threat.
Conflicts of interest
There are no conflicts to declare.
Acknowledgements
R. Rajamanikandan gratefully acknowledges his award of a University Research Fellowship (URF) from Bharathiar University, Coimbatore, India. M. Ilanchelian wishes to thank the University Grants Commission [UGC-MRP, Project No. 41-309/2012 (SR)] of Bharathiar University, Coimbatore, India for the financial support, which is gratefully acknowledged. The authors gratefully acknowledge the staff of the PSG Institute of Advanced Studies, Coimbatore, India for their help with the HR-TEM analysis.
References
- R. Bagatin, J. J. Klemes, A. P. Reverberi and D. Huisingh, J. Cleaner Prod., 2014, 77, 1–9 CrossRef.
- E. P. Randviir and C. E. Banks, Trends Anal. Chem., 2015, 64, 75–85 CrossRef CAS.
- A. B. Archana, V. B. Ankush, S. Tiffany, C. Yung and H. Xiaoxi, Sens. Actuators, B, 2016, 222, 112–119 CrossRef.
- Z. Xu, X. Chen, H. N. Kim and J. Yoon, Chem. Soc. Rev., 2010, 39, 127–137 RSC.
- J. L. Way, Annu. Rev. Pharmacol. Toxicol., 1984, 24, 451–481 CrossRef CAS.
- D. Shan, C. Mousty and S. Cosnier, Anal. Chem., 2004, 76, 178–183 CrossRef CAS.
- J. L. Liu, Y. Liu, Q. Liu, C. Y. Li, L. N. Sun and F. Y. Li, J. Am. Chem. Soc., 2011, 133, 15276–15279 CrossRef CAS PubMed.
- A. Safavi, N. Maleki and H. R. Shahbaazi, Anal. Chim. Acta, 2004, 503(2), 213–221 CrossRef CAS.
- B. Desharnais, G. Huppe, M. Lamarche, P. Mireault and C. D. Skinner, Forensic Sci. Int., 2012, 222(1–3), 346–351 CrossRef CAS.
- S. Zahra, H. Bahram and S. Mojtaba, ACS Appl. Mater. Interfaces, 2016, 8, 15177–15186 CrossRef.
- L. Dongtao, L. Lili, L. Fengxia, S. Shaomin, L. Yingfu, M. M. F. Choi and D. Chuan, Spectrochim. Acta, Part A, 2014, 121, 77–80 CrossRef.
- S. Li, J. Lihua and D. Shaojun, Chem. Commun., 2009, 3077–3079 Search PubMed.
- W. J. Jin, M. T. F. Argüelles, J. M. C. Fernández, R. Pereiro and A. S. Medel, Chem. Commun., 2005, 883–885 RSC.
- J. Gao, L. Guo, J. Wu, J. Feng, S. Wang, F. Lai, J. Xie and Z. Tian, J. Raman Spectrosc., 2014, 45, 619–626 CrossRef CAS.
- S. Dulal, S. R. D. Samuel, K. S. Anant, S. Tapas, Y. Hongtao and C. R. Paresh, Chem.–Eur. J., 2011, 17, 8445–8451 CrossRef.
- L. Yiran, W. Qianru, Z. Xiaomeng, W. Cong-ying, Y. Jianfeng, H. Xiguang, L. Xiyou, Y. Zi-feng and Z. Jingbin, Sens. Actuators, B, 2016, 228, 366–372 CrossRef.
- L. Sujin, N. Yun-Sik, C. Sung-Hee, L. Yeonhee and L. Kang-Bong, Microchim. Acta, 2016, 183, 3035–3041 CrossRef.
- Y. W. Lin, C. C. Huang and H. T. Chang, Analyst, 2011, 136, 863–871 RSC.
- S. Gulsu, U. Lokman and D. Adil, ACS Appl. Mater. Interfaces, 2014, 6, 18395–18400 CrossRef.
- M. Liu and P. Guyot-Sionnest, J. Phys. Chem. B, 2004, 108, 5882–5888 CrossRef CAS.
- M. Grzelczak, J. Perez-Juste, P. Mulvaney and L. M. LizMarzan, Chem. Soc. Rev., 2008, 37, 1783–1791 RSC.
- Y. W. Lin, C. C. Huang and H. T. Chang, Analyst, 2011, 136, 863–871 RSC.
- H. Jans and Q. Huo, Chem. Soc.
Rev., 2012, 41, 2849–2866 RSC.
- Y. Sun and Y. Xia, Analyst, 2003, 128, 686–691 RSC.
- K. Saha, S. S. Agasti, C. Kim, X. Li and V. M. Rotello, Chem. Rev., 2012, 112, 2739–2779 CrossRef CAS.
- W. Haiss, N. T. K. Thanh, J. Aveyard and D. G. Fernig, Anal. Chem., 2007, 79, 4215–4221 CrossRef CAS.
- Y. Zhiqin, H. C. Cho, C. T. Huan and L. Chao, Analyst, 2016, 141, 1611–1626 RSC.
- J. Li, T. Zhao, T. Chen, Y. Liu, C. N. Ong and J. Xie, Nanoscale, 2015, 7, 7502–7519 RSC.
- Q. Yao, X. Yuan, T. Chen, D. T. Leong and J. Xie, Adv. Mater., 2018,(1–23), 1802751 CrossRef.
- H. Zhu, N. Goswami, Q. Yao, T. Chen, Y. Liu, Q. Xu, D. Chen, J. Lu and J. Xie, J. Mater. Chem. A, 2018, 6, 1102–1108 RSC.
- R. Rajamanikandan and M. Ilanchelian, New J. Chem., 2018, 42, 9193–9199 RSC.
- R. Rajamanikandan and M. Ilanchelian, Mater. Today Commun., 2018, 15, 61–69 CrossRef CAS.
- C. Liu and W. L. Tseng, Chem. Commun., 2011, 47, 2550–2552 RSC.
- S. Pande, S. K. Ghosh, S. Praharaj, S. Panigrahi, S. Basu, S. Jana, A. Pal, T. Tsukuda and T. Pal, J. Phys. Chem. C, 2007, 111, 10806–10813 CrossRef CAS.
- N. Vasimalai and S. Abraham John, Talanta, 2013, 115, 24–31 CrossRef CAS PubMed.
- R. K. Bera, A. K. Das and C. R. Raj, Chem. Mater., 2010, 22, 4505–4511 CrossRef CAS.
- R. Rajamanikandan and M. Ilanchelian, Sens. Actuators, B, 2017, 244, 380–386 CrossRef CAS.
- N. E. Motl, A. F. Smith, C. J. DeSantisa and S. E. Skrabalak, Chem. Soc. Rev., 2014, 43, 3820–3822 RSC.
- T. Huang, F. Meng and L. Qi, J. Phys. Chem. C, 2009, 113, 13636–13642 CrossRef CAS.
- T. Premkumar and K. E. Geckeler, New J. Chem., 2014, 38, 2847–2855 RSC.
- N. Vasimalai and M. T. F. Arguelles, Nanotechnology, 2016, 27, 475505 CrossRef.
- C. W. Wang, Y. N. Chen, B. Y. Wu, C. K. Lee, Y. C. Chen, Y. H. Huang and H. T. Chang, Anal. Bioanal. Chem., 2016, 408, 287–294 CrossRef CAS.
- X. Lou, Q. Zeng, Y. Zhang, Z. Wan, J. Qin and Z. Li, J. Mater. Chem., 2012, 22, 5581–5586 RSC.
- R. Rajamanikandan and M. Ilanchelian, ACS Omega, 2018, 3, 14111–14118 CrossRef CAS.
- J. B. Zeng, Y. Y. Cao, J. J. Chen, X. D. Wang, J. F. Yu, B. B. Yu, Z. F. Yan and X. Chen, Nanoscale, 2014, 6, 9939–9943 RSC.
- S. Hajizadeh, K. Farhadi, M. Forough and R. E. Sabzi, Anal. Methods, 2011, 3, 2599–2603 RSC.
- M. H. Kim, S. Kim, H. H. Jang, S. Yi, S. H. Seo and M. S. Han, Tetrahedron Lett., 2010, 51, 4712–4716 CrossRef CAS.
- C. Cheng, H. Y. Chen, C. S. Wu, J. S. Meena, T. Simon and F. H. Ko, Sens. Actuators, B, 2016, 227, 283–290 CrossRef CAS.
Footnote |
† Electronic supplementary information (ESI) available. See DOI: 10.1039/c8ay02013b |
|
This journal is © The Royal Society of Chemistry 2019 |
Click here to see how this site uses Cookies. View our privacy policy here.