Recent advances and strategies to tailor the energy levels, active sites and electron mobility in titania and its doped/composite analogues for hydrogen evolution in sunlight†
Received
4th July 2018
, Accepted 9th November 2018
First published on 12th November 2018
Abstract
Hydrogen energy is realized to be promising as a potential replacement for fossil fuels; it is expected to become a prominent source of energy in the foreseeable future. Therefore, straightforward and cost-effective methods of hydrogen production are urgently required and are considered the current state-of-the-art in the field. Accordingly, the strategy of hydrogen production is very important; it can be achieved through photocatalytic water splitting under irradiation of suitable light sources, where materials are key factors to efficiently conduct this process. In this direction, titanium dioxide/titania (TiO2) has been demonstrated to be an excellent photocatalytic semiconductor towards hydrogen production through water splitting. However, the wide band gap structure, faster carrier recombination and inadequate charge transfer characteristics of TiO2 photocatalysts greatly limit their efficiency and restrain their practical applications in the field. In this context, the size, morphology and chemical properties of TiO2 have been tailored through a range of modification strategies. Closer insights into these processes reveal that the key factors of these modifications essentially involve tuning the crystal structures and surface characteristics and, thereby, the energy structures of titania. Therefore, in this review, we focus on energy structure modifications of TiO2 through various strategies, such as (i) tuning of size and morphology, (ii) creating defect structures, (iii) elemental doping, (iv) composites and (v) surface decoration with metal nanoparticles and co-catalysts. The content of this review provides insight into understanding the energy structure of TiO2 that supports photocatalytic hydrogen generation and therefore endows it with prospects for real-time application in the field to achieve sustainable hydrogen production.
1. Introduction
The current massive worldwide consumption of energy threatens the availability of energy sources in the future. Next to fossil fuels, hydrogen has become the most important and promising source of energy; this importance is not exaggerated, as hydrogen is believed to have potential to even replace fossil fuels. Hydrogen is a potential clean energy because its consumption does not produce pollution or toxic gases. Currently, hydrogen is being produced through various techniques, as listed in Table S1 in the ESI;† it is mainly produced from petroleum and natural gas steam reforming processes. Although the consumption of hydrogen is green, its production through existing techniques results in release of CO2 and other greenhouse gases into the environment; this has become a major factor in the increase of global warming. Therefore, a green methodology is crucially needed to produce hydrogen. On account of this, the ability of producing hydrogen through water splitting using semiconductors, especially under irradiation of light (photocatalysis), represents a significant leap in the field of hydrogen production. In general, water splitting by semiconductors is analogous to the photosynthetic process in several ways; this process has been under constant investigation since 1980 (ref. 1 and 2) and has extensively been studied by many researchers worldwide.3–13 Although photon-to-energy (PTE) conversion through a range of photocatalysts has been found to have potential, it has not yet reached the stage of implementation for practical applications. However, massive research in this direction is rapidly progressing.
Because the photocatalytic phenomenon has potential for water splitting, the key challenge lies in finding and constructing suitable materials to accomplish this process by the most efficient means. Understanding photocatalytic water splitting provides insight into the construction of efficient materials. Fig. 1 shows an energy (E) vs. pH plot, also known as a Pourbaix diagram, which demonstrates the thermodynamic stability of water with increasing pH from 0 to 14; also, Table 1 provides the redox potentials of water under acidic (0), neutral (7) and basic (14) pH conditions. From these insights, in a photocatalytic semiconductor, the valence band (VB) edge potential should be more positive than the oxidation potential (+1.23 eV) and the conduction band (CB) edge potential should be more negative than the reduction potential (0.0 eV), as shown in Fig. 2. It should be noted that ideal positioning of the band edges at these potentials will lead to overall splitting of water molecules. Accordingly, the positioning of the CB edge potential is crucial to design hydrogen evolution photocatalysts, as shown in Fig. 3.
 |
| Fig. 1 Reduction–oxidation potential of water with increasing pH (reprinted from the web: https://chem.libretexts.org/Textbook_Maps/General_Chemistry/Book%3A_Chem1_(Lower)/16%3A_Electrochemistry/24.04%3A_The_Nernst_Equation). | |
Table 1 Redox potentials of water in acidic, neutral and basic solutions
Reaction |
Acidic (pH 0) |
Neutral (pH 7) |
Basic (pH 14) |
O2 evolution |
O2 + 4H+ + 4e− → 2H2O E° = +1.23 V |
O2 + 4H+ + 4e− → 2H2O E° = +0.81 V |
O2 + 2H2O + 4e− → 4OH− = +0.40 V |
H2 evolution |
2H+ + 2e− → H2 E° = 0 V |
2H+ + 2e− → H2 E° = −0.41 V |
2H2O + 2e− → H2 + 4OH− = −0.83 V |
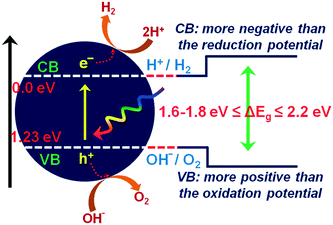 |
| Fig. 2 Ideal positioning of band edge positions in a photocatalyst with respect to the redox potential of water. | |
 |
| Fig. 3 Band edge positioning of O2 and H2 evolution photocatalysts.14 Reprinted with permission from ref. 14. Copyright 2014 RSC. | |
From a materials perspective, photochemical reactions of TiO2 open new possibilities in employing TiO2 for photocatalytic processes; it has gradually become a pioneering material in the field. In addition to the abovementioned band edge requirements; a photocatalyst should also possess properties such as decreased recombination rates and enhanced charge transportation towards achieving enhanced efficiency. On the other hand, current limitations of TiO2 include their wide band gap energy, which requires UV light to activate. Considering the photocatalytic process, because photons are driving forces to activate the photocatalyst, it is more convenient if the photocatalyst can be activated under visible light rather than UV light.15 In future, it will be more economical if sunlight can be employed to activate photocatalysts because the earth receives 5%, 45% and 50% UV, visible and NIR energies; these are called “full-sunlight”-driven photocatalytic materials. These mechanics of an ideal photocatalyst essentially suggest that TiO2 must be equipped with additional features and functions to overcome its present limitations. In this direction, the physical and chemical structures of TiO2 have been modified, which eventually alters the energy structure of TiO2 and improves its quantum efficiency and PTE conversion efficiency towards photocatalytic hydrogen production.
1.1 Scope of the review
This section provides a brief account of the present status of various materials for various photocatalytic applications and, thereby, the scope of this review.
Since the demonstration of photocatalytic (PC) hydrogen production, numerous reports have appeared in the literature.14–20 In this direction, in 2009, Kudo and Miseki comprehensively reviewed various heterogeneous catalysts16,17,21 for water splitting, where the PC water splitting abilities of elements are discussed groupwise as oxides, (oxy)nitrides and (oxy)sulphides. Similarly, in 2010, Maeida et al.18 reviewed developments in the water splitting process. In 2014, Ma et al. broadly reviewed fuel generation on TiO2-based photocatalysts, especially for production of hydrogen by biomass conversion and water splitting, as well as generation of carbon-based chemical fuels.19 Visible light water splitting is considered to be the holy grail of chemistry,20 and it has been widely explored through various strategies.21 In recent years, an increasing number of research papers have been published that explain the photocatalytic pathways for achieving enhanced solar-to-hydrogen (STH) conversion efficiency. More importantly, these studies have rapidly and vastly increased; more advances have been achieved since 2011,22–24 especially in the construction of visible-light active titania-based materials for hydrogen evolution under solar light. It is realized that TiO2 is a promising semiconductor due to its characteristic features, such as strong oxidizing power, chemical stability, optoelectronic properties, cost-effectiveness, environmental benignancy, and, importantly, its band edge potential with respect to the water oxidation potential for water splitting, as shown in Fig. 4.25–28 Consequently, interesting results have also been obtained in band gap engineering through the creation of defects and off-stoichiometry oxygen to essentially tune the band edge potential of TiO2 with respect to the redox potential of water.32 In addition, studies on the modification of TiO2 for visible light activity have been largely explored for various photocatalytic applications.33–41
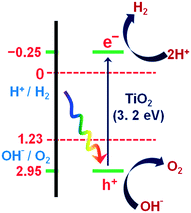 |
| Fig. 4 Band edge positions of TiO2 with respect to water redox potential towards H2 production. | |
The present review describes the strategies used and the developments achieved since 2011, with a neat focus on two main aspects of titania modification for hydrogen evolution in visible light. One is tailoring the energy structure of titania and the other is tweaking its crystal structure and surface character. Band gap reduction and band alignments induced by hydrogen doping, self-doping, codoping of cations, codoping of N-TiO2 with carbon materials, 2D materials and the formation of their composites are described. New strategies developed to form titania composites of specific oxides, such as Cu, Zn, Zr and heterojunctions, are reviewed in depth; in addition to engineering the energy structure, these strategies induce lattice and surface defects, create surface traps, and cause charge recombination/separation/migration, thus refining the electron pathways. Noble metal loading on titania has been used for many decades to enhance its water splitting activity; the mechanism by which it influences the energy structure and the SPR effect of Au and Ag loading are discussed. Finally, reports wherein well-established visible light-active sulphides are formed into composites with titania to overcome the limitation of photocorrosion are described. With regard to tweaking the crystal structure and surface character of titania, new and promising strategies used to obtain desirable shapes, size, surface areas, numbers of active sites and porosities as well as their effects on the energy structure and reactivity of titania are discussed.
1.2 Evaluation parameters of a photocatalyst towards hydrogen generation
The photocatalytic efficiency of a photocatalyst can be evaluated by various parameters that are essentially required for quantitative analysis of its efficiency. These parameters and their physical significance are discussed below.
(i) Turnover number (TON).
The TON is defined as the ratio of the number of reacted electrons to the number of atoms in the photocatalyst (eqn (1)) or on the surface of the photocatalyst (eqn (2)). The number of reacted electrons can be calculated from the amount of H2 evolved. | TON = No. of reacted electrons/No. of atoms in the photocatalyst | (1) |
| TON = No. of reacted electrons/No. of atoms on the surface of the photocatalyst | (2) |
(ii) Quantum yield (QY).
The QY can be generally defined as the number of times a specific “event” occurs per photon absorbed by the system. Accordingly, in a photocatalytic system, the QY can be calculated by determining the ratio of the number of reacted electrons to the number of incident photons. In general, the measurement of the real amount of photons absorbed by a photocatalyst in a dispersed system is difficult because of scattering effects. Therefore, the obtained quantum yield is always an apparent quantum yield (AQY) and is anticipated to be less than the real quantum yield because the absorbed photons are usually fewer than those of the incident light.21
AQY = [No. of reacted electrons/No. of incident photons] × 100 |
(iii) Solar-to-hydrogen (STH) conversion efficiency.
This is an essential parameter in evaluating the ability of photocatalysts towards their implementation in commercial solar photocatalytic water-splitting systems; it can be calculated as follows.
STH (%) = [Output energy as H2/Energy of incident solar light] × 100 |
STH (%) = [H2(mmol−1) × 237 (kJ mol−1)/Pm (MW cm−2) × Area (cm2)] × 100 |
2. Crystallinity and crystal structure
Improving the crystalline properties of a photocatalyst plays an important role in enhancing its photocatalytic activity. Increasing the crystallinity essentially decreases the density of defects, the number of recombination centers, and the diffusion length of the excited charge carriers and increases the catalytic sites in the materials, leading to enhanced photo-activity of the catalyst. The active catalytic sites are the key to enhance the rate of water-splitting reactions. Further, improving the crystalline properties of a photocatalyst leads to fine dispersion with minimum agglomeration of particles and increases the porosity and surface area, which are very influential parameters toward achieving enhanced photocatalytic activity. As discussed, in addition to appropriate positioning of the band edge positions, structural and surface characteristics such as crystallinity, porosity, surface area, and particle shape and size are value-added parameters for enhanced performance of photocatalysts. In this context, this section provides insight into the effects of the crystal structures and morphologies of TiO2 and TiO2-based materials on their energy structures towards enhancing their photocatalytic hydrogen evolution properties. The catalytic sites directly favor light absorption (irrespective of the light source) and increase the number of electrons produced and transported for efficient photocatalytic activity. A larger surface area and smaller particle size considerably shorten the distance for the migration of excitons to the surface, thus decreasing the possibility of recombination.29
Among the three crystal phases of titania, the rutile and anatase phases are highly photoactive and have been largely explored for their photocatalytic activities.30 The discrepancy in the band gaps of rutile (3.0 eV) and anatase (3.2 eV) is due to the higher CB edge position; eventually they show significant differences in their photocatalytic activity.31 Many studies have demonstrated that anatase-TiO2 shows better activity compared to rutile phase.32 However, it has been reported that a mixture of these phases synergistically enhances their photocatalytic properties due to their modified energy structures and efficient electron transport and mobility.33 Melián et al.34 reported efficient hydrogen evolution due to the synergetic effects of an anatase
:
rutile (87
:
13) mixture of TiO2. In their study, the materials were prepared through a sol–gel process, followed by either (i) hydrothermal treatment (150 °C/24 h) or (ii) heat treatment between 400 °C and 900 °C; these materials showed enhanced activity. It was also found that balanced amounts of rutile and anatase phase (with a smaller percentage of rutile phase) with a polyhedral particle shape (truncated bipyramid obtained by calcination), along with high surface area and small crystal size, yielded maximum hydrogen production.34 A green preparation method involving solid–solid transformation of NH4TiOF3 nanoplates to TiO2 nanoplates with exposed (001) facets was evaluated for H2 generation; 10 μmol h−1 g−1 of catalyst could be used due to the comprehensive impact of the specific surface area, the size of the photocatalyst, the degree of crystallisation, and the exposed (001) facets.35 High-pressure columbite phase stabilized by incorporating a plastic strain of TiO2 with enormous amounts of oxygen vacancies showed absorption in the visible light range with a band gap of ∼0.7 eV, resulting in high photocatalytic hydrogen evolution.36,37 However, there are some contradictory reports; for example, Lin et al. reported that N-TiO2 with a smaller anatase crystallite size (∼8 nm) showed lower hydrogen production activity, which was attributed to high electron–hole recombination due to the poor crystalline quality of TiO2.38,39 Interestingly, in the photocatalytic Z-scheme water splitting process,40 two semiconducting materials with different band gaps are conventionally used (i) to absorb a larger portion of solar light and (ii) to drive the proton reduction reaction (hydrogen evolution) and the oxygen evolution reaction at different sites. In these approaches, hydrogen and oxygen separation can be easily achieved, resulting in overall lower hydrogen production costs. Further, in this system, the shuttle electron mediator leads to efficient utilization of visible light compared to the conventional single-step photocatalysis process because energy is needed to activate each photocatalyst, as depicted in Fig. 5.41
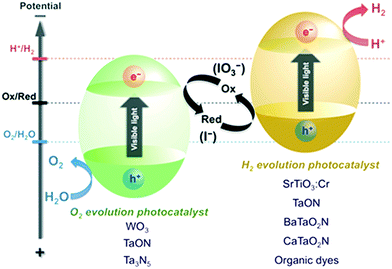 |
| Fig. 5 Schematic of a Z-scheme photosystem.45 | |
Xu et al. reported Z-scheme water splitting using anatase–rutile TiO2 nanofibers, which showed enhanced transportation of photo-exited electrons from anatase to rutile phase. Increasing the rutile-to-anatase ratio resulted in the formation of more Z-scheme heterojunctions; thus, higher photocatalytic H2-production activity was achieved.42 In another study, the effects of nano- and micro-rutile/anatase phases of TiO2 on hydrogen generation were studied; it was observed that the rate of hydrogen generation increases in the presence of nano-anatase/rutile phase compared to the micro-sized systems due to the better synergistic effects between the rutile and anatase phases in the nano-systems and their increased surface areas.43,255 In a study on overall water splitting into H2 and O2 using rutile TiO2, it was highlighted that rutile phase decreases the density of surface traps and the exposed crystal facets separate the redox active sites. Mixed phases of TiO2 were also studied for simultaneous photocatalytic hydrogen evolution and degradation of organic pollutants (by consuming photogenerated holes); it was found that the mixed phase facilitated better inhibition of recombination. It has been reported that the dominant (001) facets of mesoporous nanosheets of mixed phase TiO2 and nanorods converted into mesoporous nanosheets prominently enhance their light absorption in addition to improving their adsorption and diffusion of reactant molecules.44 Table S2† shows a list of different crystal phases/structures of TiO2 photocatalysts and their hydrogen evolution abilities.
3. Tweaking of surface characteristics
3.1 Porosity
High porosity provides a higher surface-to-volume ratio, which may result in augmentation of the surface reactivity and increase hydrogen production. Parida et al. demonstrated excellent photocatalytic activity of hierarchical and fibrous meso–macroporous N-doped TiO2 (as shown in Fig. 6), which they attributed to the interior macroporous channels interconnected with mesoporous networks in the catalyst. These provided an easy path for electrons for effectual surface charge transfer in the system. The macrochannels were also found to act as light transfer paths to transport the incident photon flux into the internal surface of TiO2 and allow photoabsorption, reflection and scattering in the system.45
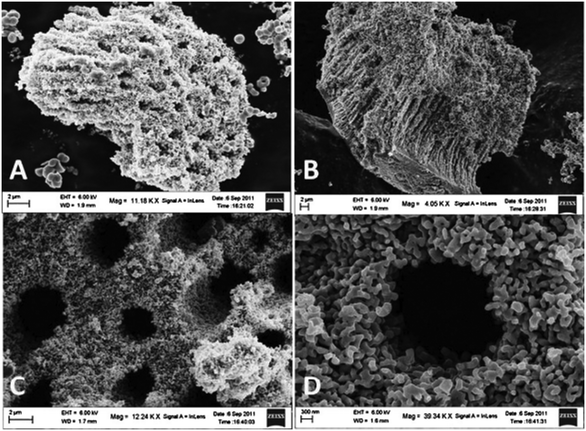 |
| Fig. 6 Meso–macroporous N-TiO2 calcined at (A and B) 400 °C (C and D) 800 °C. Reprinted with permission from ref. 23. Copyright 2013 Elsevier. | |
Niphadkar et al. obtained the best results for a mesoporous composite containing 60% TiO2 with siliceous MCM-41 (MTi60) to show enhanced hydrogen production (0.08805 mmol g−1 h−1) compared to other composite photocatalysts.46 The mesostructural feature of siliceous MCM-41 was found to be connected without pore blockages even with high TiO2 loading. The sheet-like morphology of TiO2 in MTi60 was reported to be favorable to maximize hydrogen generation. Wang et al. prepared three-dimensionally ordered macroporous (3DOM) N-doped anatase TiO2 (N-TiO2) that showed increased activity under visible light. The 3DOM structure demonstrated enhanced pore structure, periodicity, large pore volume and multiple scattering for better visible light harvesting and hydrogen evolution.47 Han et al. used graphitic-C3N4 nanosheet (g-C3N4 NSs) hybridized N-doped TiO2 nanofibers and demonstrated better visible light absorption due to the enhanced surface area and electron transport ability, resulting in increased photo-conversion and a better rate of hydrogen evolution.48 Sreethawong et al. synthesized nano-crystallite TiO2 with a high surface area of 80 m2 g−1 and a total pore volume of 0.12 cm3 g−1; it displayed enhanced hydrogen generation of 437.5 μmol h−1.49 The mesoporous crystalline anatase-TiO2 was prepared by utilizing mesoporous silica KIT-6 as the precursor; interestingly, this led to the production of mesoporous anatase TiO2 instead of rutile phase, even at a calcination temperature of 750 °C, which was essentially due to the template confinement effect of the mesoporous silica. Similarly, TiO2 with a surface area of 207 m2 g−1 and loading of 0.5% Pt showed enhanced H2 production of 2000 μmol h−1 g−1, which was found to be around 1.7 times greater than that of Degussa P25 TiO2.50 Table S3† shows various porous-structured TiO2 photocatalysts and their hydrogen evolution.
3.2 Particle shape
Researchers have demonstrated various morphologies of TiO2, such as sheets, rods, and hierarchical structures, and achieved increased hydrogen evolution compared to that of spherical particles. Teng et al. obtained sheet-based CuO/TiO2 composites and compared their hydrogen evolution capacity and stability with those of bare-TiO2 rods. It was found that the interface of CuO and TiO2 facilitated stable p–n heterojunctions due to the strong interaction of the facets of the TiO2 sheets with CuO, which eventually led to decreased recombination of the excited charge carriers.51
A butterfly wing architecture of TiO2 was synthesized,52,53 as shown in Fig. 7, using the wings of the butterfly Papilio nephelus boisduva as a template. Interestingly, this wing architecture of TiO2 decreased UV light reflection by about 40%. The structure was found to act as an optical trap wherein light was repeatedly reflected and absorbed within the system. As a result, remarkable improvements in the light absorption and harvesting properties were observed, and significantly enhanced water-splitting was achieved. Kiatkittipong et al. prepared sodium titanate and hydrogen titanate tunnel-like structures; they showed enhanced separation of charge carriers compared to TiO2, which was essentially due to their tunnel-like structures.54 Guo et al. prepared mesoporous titanium phosphates and tuned their morphologies from fibrous shapes to near-hexagonal shapes, as shown in Fig. 8, by optimizing the concentration of phosphoric acid. As a result, the presence of abundant hydroxyl groups, 2D layered structures, large specific surface areas and enormous amounts of exchangeable protons resulted in enhanced hydrogen evolution.55
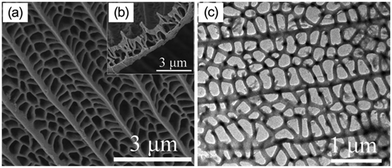 |
| Fig. 7 Image of WA-TiO2: (a and b) WA-TiO2 FESEM image; (c) TEM image of WA-TiO2. Reprinted with permission from ref. 63. Copyright 2013 Elsevier. | |
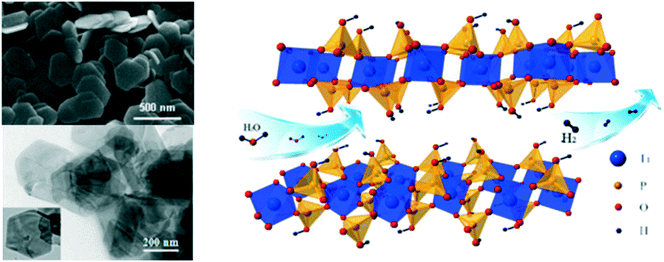 |
| Fig. 8 TEM image and molecular structure of layered α-titanium phosphate. Reprinted with permission from ref. 65. Copyright 2014 Elsevier. | |
1D/3D hybrid hierarchical TiO2 composite (HHC) and 3D hierarchical TiO2 (3D-HTiO2) structures were prepared by Haider et al. and produced 661 and 1310 μmol h−1 g−1 of hydrogen, respectively, which are around 1.72 and 3.4 times greater than the amount produced by Cryst-TiO2-Memb (titania nanotube membrane); this was essentially due to their enhanced specific areas. Similarly, lower and higher concentrations of NaOH (a morphology directing agent) in the reaction led to the formation of self-assembled loosely and densely packed hierarchical spheres, respectively, as shown in Fig. 9;56 they showed hydrogen evolution of up to 1996 μmol h−1 g−1, which was 5.18 times greater than that of Cryst-TiO2-Membrane. Table S4† shows a list of different particle shapes of TiO2 photocatalysts towards H2 evolution.
 |
| Fig. 9 a) SEM, b), c) TEM, and d) HRTEM images of 3D hierarchical protonated titanate microspheres. Reprinted with permission from ref. 66. Copyright 2014 ACS. | |
3.3 Core–shell structured titania
Core/shell structures are generally biphasic materials that consist of an inner core and an outer shell material, as depicted in Fig. 10. These core/shell structures include a wide range of combinations of organic and inorganic materials.57 The ‘core’ architecture supports the ‘shell’ material in terms of surface area, charge and porosity, where synergistic interactions between the core and shell materials enhances electron transfer and trapping in the system and increases its efficiency.58 Im et al. reported that a CuS–TiO2 core/shell photocatalyst liberated 10-fold more H2 than CuS; this was mainly because of the slower charge carrier recombination rates due to the core/shell architecture. TiO2 shells covered the core CuS completely, and the photoexcited electrons from the CB of TiO2 moved to the valence band of CuS because its reduction potential is higher than that of TiO2; also, the core CuS acted as a good electron collector.59
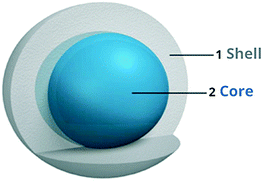 |
| Fig. 10 Schematic of the core–shell structure.56 | |
Fang et al. prepared Au@TiO2 core–shell structures, as shown in Fig. 11, and decorated them with CdS nanoparticles to demonstrate the absorption of longer wavelength light.60 This unique ternary design displayed improved photocatalytic activity, which was attributed to the facile electron transfer path from CdS to Au via TiO2 (CdS → TiO2 → Au); it eliminated the need for a co-catalyst by acting as an interior active catalyst for H+ reduction for H2 generation.61
 |
| Fig. 11 Schematic of H2 generation in Au@TiO2–CdS nanostructures. Reprinted with permission from ref. 48. Copyright 2013 ACS. | |
Yang et al. synthesized core–shell structured rutile TiO2-sulfide nanoparticles. It was found that the formation of Ti3+ and sulphide enhanced the visible light absorption (from 3.0 eV to 1.7 eV) of the system.62 The TiO2-coated iron-oxide core shell particles showed high photocatalytic activity, with release of 2700 μmol h−1 of H2 with a quantum yield of 3.86% at 550 nm due to efficient charge transfer from TiO2 to Fe2O3 and the suppression of e−/h+ pair recombination.63,64 Liu et al. synthesized TiO2 microspheres containing B dopant,65 and they created a gradient by migrating boron from the core to the shell of anatase TiO2 to modulate the hydrogen and oxygen generation reactions. As a result, 4 times higher H2 generation was observed in the boron-free shell (50 μmol h−1) than in the shell with boron because of the stronger reducing power of the boron-free shell, whereas the holes in the latter showed stronger oxidative power than those in the former, as depicted in Fig. 12. This is essentially because extra electrons caused downward band bending in the system;66 also, the Ti3+ formed by inducing interstitial boron into TiO2 contributed electrons to the neighboring Ti4+ ions and enhanced the photocatalytic properties of the system.67,68
 |
| Fig. 12 Boron-free and boron-rich shells of TiO2. Reprinted with permission from ref. 46. Copyright 2012 Wiley. | |
Kim et al. prepared core–shell structured NiFe2O–TiO2, as shown in Fig. 13, that exhibited remarkably higher H2 evolution than pristine TiO2. Upon UV-light irradiation of the system, the photoexcited electrons from the CB of the TiO2 shell migrated to the surface of the shell and were finally injected into the CB of the NiFe2O4 magnetic core. Fe ions in NiFe2O4 were found to be reduced after the photoreaction, which indicates that the core attracted electrons during the photocatalytic reaction and enhanced the hydrogen evolution efficiency.69 Lin et al. reported that a highly porous, three-dimensional well-connected thorough-pore structured TiO2 aerogel was a superior material for photocatalytic hydrogen generation (328 μmol g−1 h−1); it showed about a 9.63 times higher rate than commercial P25-TiO2 nanoparticles. The small arc-radius, highly porous, three-dimensional, well-connected thorough-pore structure of the aerogel provided highly effective charge carrier separation and transfer in the system.70 Further, Parayil et al. synthesized a TiO2 aerogel that produced 390 μmol h−1 g−1 of H2 in 4 h of irradiation. The enhancement was attributed to the decreased particle sizes, large pore diameters, high surface area and low density of the TiO2 aerogel foam.71,72
 |
| Fig. 13 Core–shell structure of NiFe2O4@TiO2. Reprinted with permission from ref. 46. Copyright 2014 Elsevier. | |
4. Tailoring of energy structure
It is known that the conduction band (CB) of TiO2 is composed of an empty orbital of the metal cation with a d0 configuration. The crystal structure of titania and the bonding character between the metal and oxygen strongly influence its valence band (VB) because it consists of an O 2p orbital and is placed at 3.0 eV.73,74 Therefore, doping of titania with different elements is a well-recognized method to modify its crystal structure, and in most cases, the host ion will be replaced by the dopant.75 The transition metal or non-metal cation dopant has a partially filled d orbital, so a donor or acceptor level will be formed in its forbidden energy gap; this essentially tends to decrease the band gap energy of TiO2 and enables visible light absorption.76 Therefore, it is crucial to have a new electron donor level (DL) other than O 2p to lower the CB, through which the band gap energy of TiO2 can be decreased.77–80 The process of doping in TiO2 essentially induces impurity levels in the semiconductor and modulates its electrical and optical properties,81,82 thereby increasing the charge carrier separation and efficiency to absorb 43% of visible light. These doped impurity-like atoms function as either acceptors or donors to the TiO2. The donors have more valence electrons and therefore donate their extra electrons to the CB of TiO2; this increases the electron carrier concentration in TiO2 and makes it n-type.83 More importantly, the nearest energy band to the CB and VB is generally referred to as the dopant d-band; on irradiation, it excites electrons from the dopant band to the CB or from the VB to the dopant band and thereby requires less photon energy, which facilitates visible light absorption of the photocatalyst.84
4.1 Photoexcited charge carrier trapping
The process of doping may lead to crystal losses by generating off-stoichiometricity through creating vacancies in TiO2 that can essentially act as traps or recombination centers. Therefore, the concentration of dopants is crucial when using the doping strategy to enhance the absorption range of titania, especially in the visible region. The recombination of the photoexcited e−/h+ pair must be delayed for a proficient charge transfer process on the photocatalyst surface. Accordingly, charge carrier trapping inhibits recombination and augments the lifetime of the excited e−/h+ pairs to more than a fraction of nanosecond. Further, the surface states created by the dopants are localized; therefore, the surface trap population depends on the energy dissimilarity between the traps and the bottom of the CB and there is a decrease in entropy when the electrons undergo the trapping process.85 Gratzel et al. reported electron paramagnetic resonance (EPR) spectroscopy of illuminated colloidal TiO2 at 4.2 K and showed the presence of trapped photoexcited electrons (to form Ti3+ defect sites) and holes in the bulk TiO2 semiconductor.86 Similarly, picosecond and nanosecond laser photolysis was employed to examine the charge carrier recombination and trapping in ∼6 nm colloidal particles of TiO2. It was observed that the CB electron trapping was very fast, occurring within 30 ns, and the hole trapping required an average time of 250 ns. It should be noted that recombination of trapped electrons with free or trapped holes occurs in 10−11 to 10−6 s, and the mean lifetime of a single electron–hole at a lower charge carrier concentration is around 10−10 s; meanwhile, at higher concentrations, recombination occurs in a fraction of a nanosecond. Holes are comparatively unreactive towards electrons in the trapped state.87 Thus, the carrier trapping should be rapid to achieve successful photochemical conversion. Charge carrier de-trapping is also important because the photocatalytic reaction will only be able to occur if migration of trapped electrons/holes to the surface occurs in the system. It has been realized that surface doping of TiO2 particles is better for charge transfer because deep-doped species can behave as recombination centers due to the difficulty of electron/hole transfer to the interface. This essentially signifies that it is pertinent to maintain the dopant concentration if TiO2 must behave as an extrinsic semiconductor; hence, optimum concentration plays a key role. As a result, co-doping and mixed metal oxide doping have been found to overcome the negative effects of doping.88
4.2 Metal ion/cation doping
The electronegativity, spatial distribution, chemical state and ionic radius of hetero-atoms are the basic parameters of this method. To date, numerous and encouraging advances have inspired the development of hetero-atom doping in semiconductor photocatalysts towards proficient utilization of visible (solar) light for photocatalytic applications.65,89–91
4.2.1 Lanthanides as dopants.
Doping of lanthanide ions into TiO2 potentially leads to active materials for photocatalytic reactions. Among the previously studied lanthanides, La, Ce, Pr, Nd, Sm, Er and Gd show promising activity because of their ability to transport charge carriers to the interface. Initially, in 2004, Zhang et al. reported that the exceptional spectral characteristics and electronic configuration of lanthanides can modify the crystal structure of TiO2,92 which was later validated by Zhou et al. in 2006.93 However, Zongyan et al. showed in 2008 that the 4f/5d states of lanthanides considerably contribute to induce impurity energy levels (IELs) and shift the absorption edges to the visible region while maintaining strong redox potentials in the system. The position of the IELs depends on the atomic number, and it moves from the CB to the VB with increasing atomic number of the lanthanide dopant. It has been proposed that a continuum in the VB or CB formation in TiO2 with the atomic orbital of the dopant atoms is more desirable than discrete energy levels.94 Further, it was also found that lanthanide doping can cause significant development in the photoresponse of TiO2, and it is sensitive to the electronic and atomic configurations and ionic radius of the dopant. Pristine TiO2 consists of VB O 2p and CB Ti 3d states, and the Ti 3d states can be split into t2g and eg states in an octahedral ligand field with Oh symmetry; hence, the CB is divided into upper and lower parts, and these effects lead to band gap narrowing in TiO2. Properly positioned IELs permit low energy photons to excite the electrons from the VB to the CB; they can also trap and detrap electrons, therefore extending the lifetime of charge carriers.95 Additionally, lanthanides tend to fill oxygen into the lattices of TiO2 and create surface oxygen vacancies for electron harvesting96 because the lanthanides have higher bond strengths and affinity for oxygen atoms than the Ti atoms. Kuvarega et al. also proved that doping of Sm, La, Eu, Yb, Nd, etc., is more suitable for TiO2 to act as a photocatalyst, which also aids the separation of photo-generated excitons and prolongs their lifetime for better photocatalytic performance.97,98
Peng et al. have particularly studied (amongst lanthanides) the effects of rare earth (RE)-doped (La3+, Eu3+, Er3+, Gd3+) Pt/RE/TiO2–CdS (with 1.0 wt% deposited Pt) systems. They found that photocatalytic hydrogen evolution was enhanced by 62.0%, 40.4%, 34.7% and 30.0%, respectively, compared to bare Pt/TiO2–CdS. RE ions tend to enter the TiO2 lattice; this causes crystal lattice stress, escalating the Fermi level and causing a negative shift of the flat band potential in TiO2. The negative shift in the CB position of TiO2, as shown in Fig. 14, allows it to attain higher reduction power or increases the driving force for electron transport to the H+/H2O redox level, competently contributing to hydrogen generation. Accordingly, the order of enhancement was found to be La3+ > Eu3+ > Er3+ > Gd3+, which was opposite to the order of the shift in flat band potential (Gd3+ > Er3+ > La3+ > Eu3+); this indicates the existence of a necessary exchange between the reduction power and electron injection. However, the energy difference in the CB of CdS and the CB of TiO2 decreases with increasing negative shift, which was found to be a disadvantage in this case.99 Yttrium-doped TiO2 nanoparticles were reported to show high photocatalytic activity due to oxo-bridge formation, which favors the photocatalytic process by re-designing the charge separation and transfer processes.100,101
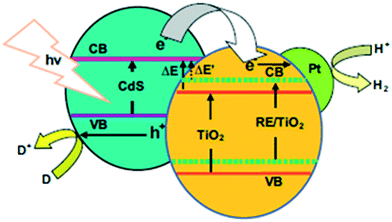 |
| Fig. 14 Rare earth material-doped TiO2 band structure. Reprinted with permission from ref. 73. Copyright 2013 Elsevier. | |
4.2.2 Cations as co-dopants.
4.2.2.1 Fe and Ni as co-dopants.
Enormous numbers of studies have been performed on doping of almost all possible metal ions to date.102–107 The most extensively studied dopant is iron; since its first study in 1983 as a mono-dopant of TiO2, it has been proven to exhibit visible light activity, which has been reported to decrease the work function and induce good redox capability and cause oxidization and reduction at the interface. Co-doping is an up-and-coming research area; therefore, we examined the effects of co-doping Fe, Ni and Rh into TiO2.108 Fan et al. reported an enhanced visible light hydrogen generation of 515.45 μmol h−1 g−1 when Ag nanoparticles (Ag NPs) were doped into Fe 3d-doped TiO2 nanoparticles (Fe/TiO2 NTs) arrays.109 In addition to obtaining a Fe impurity level (which can maintain and prolong the lifetimes of excited electrons and allow transfer again to the CB of TiO2), the coating of Ag0 metal particles (with strong electron-accepting ability) on the surface acted as electron traps because the Fermi level of Ag is lower than that of TiO2. This causes the excited electrons to transfer themselves easily to Ag0 (and, subsequently, to the 3d level of Fe3+), leading to electron hopping and decreased recombination of excitons.
Fe, Ni co-doped110 TiO2 nanoparticles prepared by Sun et al. using a thermal method showed enhanced charge carrier separation and also extended the absorption of visible light to 514 nm.111 The observed visible light absorption indicated that the decreased band gap of TiO2 can be attributed to the impurity level and decreased charge carrier recombination rates, which resulted from the creation of defects that trapped and detrapped electrons for efficient charge separation and transfer during the photocatalytic process.112 Overall, appropriate co-doping largely facilitates the favorable alignment of energy levels and allows efficient electron transfer, visible light absorption and charge separation for photocatalytic hydrogen evolution. Ni(OH)2 decorated on TiO2 nanotubes (TNT) in aqueous glycerol solution resulted in high H2 generation of 4719 μmol h−1 gcat−1, which is 12 times higher than that of pristine TNT. The improved activity is due to the high electronegativity of the Ni(OH)2 quantum dots present on the surface of TNT, which facilitates efficient shuttling of photoexcitons, resulting in decreased electron–hole recombination in TiO2 during photocatalysis.113,114
4.2.2.2 Multi-cations as co-dopants.
It has been found that through co-doping of multiple cations, one can tune the optical, electronic and photocatalytic properties of TiO2 with enhanced thermo-dynamical stability. However, achieving good crystallinity is an enormous challenge in multi-cation co-doped systems. Allured et al. studied a pyrochlore A2B2O7-type material that was synthesized using a simple fluorite-type structure (AO2 and its multiples)115 and its photoactive counterpart.116,117 Upon the introduction of Fe in the Bi2Ti2O7 (BTO) lattice, they observed increased absorption of visible light in the system. Further, density functional theory (DFT) demonstrated that the introduction of Fe enhances the visible light absorption more than other elements in the 3d group118,119 because the inter-band created with Fe doping is closer to the VB.115 However, the Fe 3d band is probably more prominent with increasing concentration of Fe; it may substitute Bi and Ti atomic sites in BTO, which can lead to electron photoexcitation between the 3d bands of Fe (valence band) and Ti (conduction band) and enhance the hole effective mass due to the increased Fe 3d-band presence.
Multi-metal co-doped titania films were prepared by Li et al. using dopants such as Mn and W. They reported that the entry of Mn and W into the TiO2 lattice occurs by partial substitution of Ti. Mn and W induce new electronic states above the VB edge of TiO2 and lead to enhanced solar light absorption. The red shift with increasing concentration of dopants is ascribed to the charge-transfer transition between the d electrons of Mn/W and the CB of titania. Accordingly, their XPS studies revealed the existence of greater oxygen content than the required stoichiometry. This further revealed that this oxygen content consists of interstitial oxygen atoms that are present due to the titanium vacancies which originated from the valence difference in W6+ and Ti4+. This allowed efficient detrapping of charge carriers to the surface; as a result, hydrogen evolution up to 900 μmol h−1g−1 (from 300 μmol h−1 g−1) was achieved for the optimal dopant concentration.120 Huang et al. reported that Rh, Nb-codoped TiO2 nanorods (with Ti0.996Nb0.002Rh0.002O2 as an example) exhibited both absorption of visible light and efficient charge carrier separation. Montoya studied the UV photodeposition of Co, Ni, or Cu onto TiO2, resulting in visible light absorption with improved H2 evolution of 8500 μmol h−1 g−1 (7% QY) for Cu (1%)
:
TiO2versus 500 μmol h−1 g−1 (0.4% QY) for P25-TiO2. TiO2–Ni(OH)2/CNT/CdS hybrid showed H2 generation of 12 mmol g−1 h−1, which was attributed to the combined effects of Ni(OH)2 and CNTs.121
The effects of Rh co-modification on Sn-grafted TiO2 (aggregated Sn species were reported122 to induce a downshift of the LUMO, decreasing the reducing power of the photogenerated electrons for H2 evolution, unlike isolated species) were studied by Sheng et al. The photon energy required to photoexcite the isolated oxide is higher than that for the extended oxide of the same metal; therefore, the oxidation and reduction potentials of the holes and electrons are also much higher for these systems.123 Therefore, no obvious change in the absorption was observed, which was also in agreement with theoretical calculations. However, the enhancement of hydrogen evolution from 0.05 mmol to 1.72 mmol was observed. Their XPS findings revealed the presence of Rh0 and Rh3+ species in the co-modified Sn-grafted TiO2,124 where it was proposed that the isolated Sn species acted as mediators for the electron transfer from TiO2 to Rh (Fig. 15) and also aided in decreasing the charge recombination, thus enhancing the photocatalytic activity.
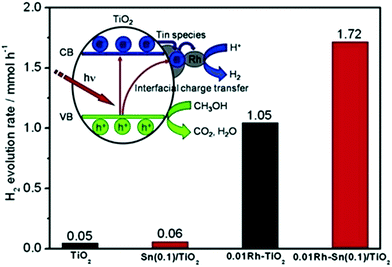 |
| Fig. 15 Photocatalytic hydrogen evolution (inset shows a schematic of H2 evolution) over Rh-loaded Sn/TiO2. Reprinted with permission from ref. 88. Copyright 2014 Elsevier. | |
This design was further proposed to have enormous value if it could be extended to other metal ions (to replace Sn) that could also extend the visible light activity of TiO2.122 Accordingly, many combinations of these co-dopants can be found, and we have selectively reported the most recent and promising examples. Extensive studies can also be found on the usage of various metals for co-doping with Pt-doped TiO2 to enhance its visible light activity; hawse have also reviewed these in a separate section under noble metal modifications of TiO2.
4.2.2.3 Ti3+ as dopant.
The visible-light photocatalytic activity of Ti3+ self-doped anatase is generally attributed to two main factors.125 The presence of Ti3+ in TiO2 generates anion vacancies in the lattice, which induces additional anion vacancies below the CB. Meanwhile, the increment in these vacancies leads to a decreased band gap in TiO2. Thus, visible light can induce the electron transition from the VB of TiO2 to the Ti3+ states and OV states.126 Crystalline anatase phases of nitrogen-doped TiO2 (N-TiO2), boron–nitrogen co-doped TiO2 (B–N-TiO2), and undoped TiO2 (TiO2) were synthesized by Sayed et al. using a facile xerogel process followed by reduction in a glycol medium; this process forcibly created Ti3+ ions in the phases (R-N-TiO2, R-B–N-TiO2, and R-TiO2, where R stands for reduced) and enhanced their visible light activity, as observed in Fig. 6, where the extension of the absorption range appears to reach a maximum in self-doped TiO2.127 Accordingly, they found that self-doped TiO2 showed hydrogen evolution of 2.5 μmol/50 mg, in contrast with the nil activity of bare TiO2. As observed in Fig. 16, the extension of the absorption range appears to reach a maximum in self-doped TiO2.127
 |
| Fig. 16 Diffuse reflectance spectra of TiO2, N-TiO2, B–N-TiO2 and pristine TiO2. Reprinted with permission from ref. 91. Copyright 2012 ACS. | |
Furthermore, the same group worked on combining self-doped TiO2 with graphene. They synthesized reduced self-doped TiO2–graphene nanoplatelets (TiO2R–G) that exhibited a decrease in their band gap energy, resulting in enhanced visible light absorption; this was largely promoted by the Ti3+ dopant. It was also found that the enhanced electron transport properties of graphene increased the lifetime of the excited charge carriers.128 Further, their findings were well supported by the theoretical calculations performed by Mozos et al.129 Both the theoretical and experimental results showed that band gap decrease was possible due to the existence of oxygen vacancies owing to the formation of Ti3+. Silva et al. prepared Ti3+/Ti2+ reduced states in TiO2 which showed visible light activity, with a solar-to-hydrogen energy conversion efficiency of 0.89% and a quantum yield of 43% at 655 nm.130 Kong et al. induced defects such as oxygen vacancies and Ti3+ through plasma treatment, which decreased the band gap from 3.13 eV to 2.88 eV.131 Wang et al. also confirmed enhanced visible light response due to the significant red shift and band gap decrease in these systems.44 These observations were mainly attributed to the presence of oxygen vacancies and Ti3+; this was also confirmed by EPR signals and Raman spectra. O-Vacancies/carbon layer coated single crystal TiO2 with absorption at 415 nm showed 69.7 μmol h−1 g−1 H2 evolution (quantum efficiency ∼2.3%); this enhancement can be attributed to efficient separation of charge carriers by O-vacancies/carbon coating.132
4.2.2.4 Hydrogen-doped TiO2.
Hydrogen doping in titania essentially leads to black coloration of TiO2, which is called black titania (B-TiO2). Structurally, B-TiO2 possesses oxygen vacancies (Ti3+),133–135 surface disorders and surface hydrogenations,136,137 which play roles in its visible light absorption and photocatalytic activity. The first report on black titania (TiO2 nanocrystals hydrogenated at 20 bar H2 atmosphere) by Chen et al. in 2011 successfully demonstrated large solar light absorption (∼30%).91 Zhou et al. reported a new method using hydrogen plasma to produce black titania (TiO2@TiO2−xHx) that showed ∼83% solar light absorption; this was attributed to localized surface plasmon resonance, similar to Ag.138 Elbanna et al. confirmed that reduced or black TiO2 shows higher photocatalytic activity compared to white TiO2 due to the presence of Ti3+, oxygen vacancies and disorder layers, which improves light absorption.139 Further, the treatment was also found to convert surface Ti4+ (3d0) into Ti3+ (3d1) by introducing H doping (TiO2−xHx) and/or oxygen vacancies (TiO2−x) and to amplify the concentration of carriers (electron) in the system. The Raman spectral peak-shifting and broadening of TiO2−xHx indicated the breaking of the original symmetry of the TiO2 lattice, which is essentially due to the disordered surface of B-TiO2. The decreased intensity of the PL signal revealed the decreased possibility of recombination in the black TiO2−xHx system. They further claimed that the H2 treatment reduced the localized Ti3+ states. As a result, the black TiO2−xHx sample showed remarkable hydrogen evolution (≈10 mmol h−1 g−1) and is categorized among the best catalysts.
4.3 Anions as co-dopants
The doping of titania using non-metals, such as N, S, P, F and C, was also found to enhance the photocatalytic properties of TiO2. Among non-metals, carbon, nitrogen and sulfur have been found to be highly promising for hydrogen evolution. For example, the system of C-TiO2, N-TiO2 and S-TiO2 with triethanolamine as a sacrificial agent produced significantly increased hydrogen evolution amounts of 2947 μmol h−1 g−1, 1008 μmol h−1 g−1 and 1269 μmol h−1 g−1, respectively; this is ascribed to their enhanced visible light absorption due to the formation of electronic states above the VB edge of TiO2.140 Doping of various single anions into TiO2 has been greatly studied; herein, we have examined recent and promising strategies such as combining various carbon forms into N-doped TiO2 and co-doping of anions (S, Ce and P) into TiO2.
4.3.1 Co-doping of N-TiO2.
In the system of nitrogen-doped TiO2, the interstitial N or NOx species intercalate into the TiO2 structure and largely facilitate photocatalytic hydrogen generation. Further, this activity was found to be dependent on the amount of nitrogen species loaded into the TiO2.19 It has been claimed that N doping replaces oxygen atoms in TiO2 and produces localized energy states above the O 2p VB at 0.73 eV, which considerably reduces the band gap energy of the host titania.141 To account for this, Mountjoy et al. performed XANES analysis; they reported that these impurity states located in the band structure of TiO2 lead to narrowing of its band gap.142 In another study, it was observed that the increase in the exposed high surface energy facets upheld the formation energy of oxygen vacancies, which can function as reaction centers to enhance the photocatalytic activity.143 Hence, the effects of co-doping in N-doped TiO2 can be versatile and result in overall positive or negative effects depending upon the doping strategies and dynamics.
4.3.2 P, Ce, S as co-dopants for N-doped TiO2.
Nitrogen doping was also found to have negative effects on TiO2 by inducing long-range distortion in the TiO2 crystal structure and maximizing the carrier recombination. Oxygen vacancies in N-TiO2 act as recombination centers that further decrease the photocatalytic activity.85 However, the mechanics of overall hydrogen generation by N-doped TiO2 have not yet been realized to date.21,144,145 Interestingly, Zheng et al. found that P–N co-doping into TiO2 efficiently decreases the long-range defects and recombination induced by N doping.146 Notably, the N-TiO2 and P–N-TiO2 microstructures were systematically examined by TEM, as shown in Fig. 17 and 18. Fig. 17 shows long-range defects in the N-TiO2 sample, while the features of P–N-TiO2 include very negligible amounts of local distortions. Appropriate mole ratios of P to N can drastically minimize these long-range defects.146 Accordingly, the hydrogen and oxygen generation rates for P–N-TiO2 were found to be 11.2 μmol g−1 h−1, in contrast to zero μmol g−1 h−1 for N-TiO2. However, it was further found that their photocatalytic hydrogen evolution depended on the concentration of dopants and the reaction temperatures during the synthesis of P–N doped TiO2. These observations provided new strategies to repair the structural damage in semiconductors that arises from the hybrid-heteroatom-doping process.
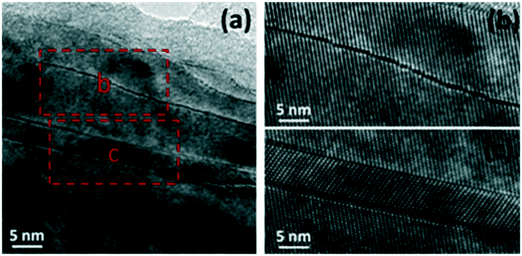 |
| Fig. 17 (a–c) N-TiO2 microstructures: (a) long-range defects, (b) anti-phase boundary in the lattice image and (c) lattice image depicting [110] twins. Reprinted with permission from ref. 121. Copyright 2014 Elsevier. | |
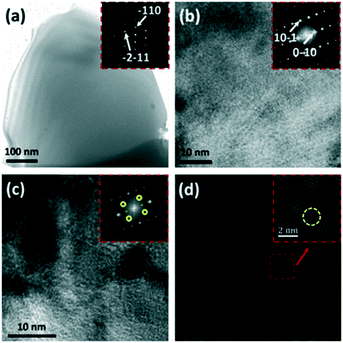 |
| Fig. 18 (a–d) P–N-TiO2 microstructures: (a) TEM image of a grain with an inset SAED pattern; (b) lattice image with an inset FFT image; (c) lattice image depicting the inhomogeneous crystal structure with an inset FFT image; and (d) IFFT image from two sets of [111] reflections. Reprinted with permission from ref. 121. Copyright 2014 Elsevier. | |
Sun et al. reported cerium co-doped N-TiO2 and showed that Ce doping hinders the growth of crystallites and also prevents the phase transition. Further, the controlled effects of Ce and N largely contributed to the red shift; as a result, the hydrogen generation was found to be best for 0.6% (mole ratio) Ce co-doping. A precise amount of Ce ions was found to act as hole traps, whereas a surplus amount of Ce considerably decreased the light absorption by covering the surface of TiO2 and decreased the photocatalytic activity by behaving as recombination centers.147
2D nanosheets of N–S-TiO2 with exposed (001) facets were synthesized via a hydrothermal process by Xiang et al. using thiourea as a precursor.149 Their XPS analysis confirmed the incorporation of S and N into TiO2 through cationic substitution-type S-doping and interstitial N-doping, respectively. The absence of anionic N (O–Ti–N (BE = 397.0 eV)) was observed; this indicated the presence of interstitial nitrogen in the lattice of TiO2, which generally occurs in O atmosphere, unlike substitutional nitrogen, which is likely to occur in the absence of oxygen.150 Most of the S species are in the S6+ state, and doping of anionic sulfur is difficult due to the larger ionic radius of S2− (1.7 Å) than of O2− (1.22 Å) and the higher required activation energy. In contrast, the replacement of Ti4+ by S6+ was found to be more favorable through chemical modification. The observed visible light activity was ascribed to the synergetic effect of doping and exposure of (001) facets of the 2D nanosheets, which enhanced the adsorption of water molecules. However, the application of this photocatalyst for hydrogen generation did not appear to be significant. Moreover, the anatase S-doped TiO2 with a high surface area showed better activity than N-TiO2;151 the red shift shown in Fig. 19 was mainly responsible for the enhanced visible light activity.152
 |
| Fig. 19 UV-visible spectra of TiO2, S-TiO2 and N-TiO2. Reprinted with permission from ref. 140. Copyright 2008 Hindawi. | |
Bai et al. reported the co-doping of C and S into hierarchical meso/nanoporous TiO2 and achieved enhanced H2 generation efficiency.153 The calcination temperature was found to promote the crystallization of TiO2 and enhance its light absorption ability. Co-doping of C and S on TiO2 were also found to accelerate the electron transfer and create active sites to trap charge carriers. Further, the hierarchical meso/nanoporous structure increased the light absorption and reflection, which increased the accessibility for the reactants to reach their desired reaction sites.
4.3.3 Carbon as co-dopant for N-doped TiO2.
It is observed from literature reports that similar to N-doping, C-doping can also involve either substitution148,154–156 or interstitial157,158 species in TiO2 systems. Chen et al. prepared C–N-TiO2 through a sol–gel method and observed enhanced activity. Carbon doping tends to form layers of a complex mixture of active carbon and carbonate species, which retards the crystal growth and enhances the light absorption of TiO2. The carbonaceous species act as a photosensitizer and enhance the electron transport rate. Nitrogen doping induces visible light absorption and shifts the flat band potential to a higher level, which eventually enhances the driving force for electron transport from the carbonaceous species and accelerates interfacial electron transport. Many groups have explored different methods of preparing these co-doped species; however, their application in hydrogen evolution did not yield satisfactory results.159
Liu et al. reported the codoping of carbon and nitrogen into mesoporous TiO2; a high surface area of 100 m2 g−1 from 54 m2 g−1 was achieved. The mesoporous structure of TiO2 linked by carbon species enabled uniform and enhanced light distribution inside the pore channels and increased the light absorption. Further, it was found that the synthesized photocatalyst was 37 times more efficient than commercial P25-TiO2. It was proposed that moderate C, N-codoping of 0.75% was favorable for the photocatalytic evolution of H2 (81.8 μmol g−1 h−1).160 Jia et al. synthesized carbon-coated N-doped TiO2 nanotube films; they showed increased carrier mobility and lower recombination rates because of the good electronic properties of graphitized carbon, which acted as a photosensitizer for the TiO2 system.161
4.3.4 Graphene with N-doped TiO2.
It is known that the graphene is a 2D carbon nanomaterial with a zero band gap that possesses excellent electrical conduction in two dimensions.162 It was found that incorporation of graphene in TiO2 increases the photocatalytic activity and H2 evolution163–166 because of its excellent electron transport nature and suppression of the recombination possibilities in TiO2.167,168 Graphene oxide (GO) with N-doped TiO2 showed higher hydrogen evolution of 112.0 μmol h−1 g−1 under a xenon lamp, which was about 13.6 times higher than that of P25 photocatalyst. This was ascribed to the synergetic effects of N-doping and GO incorporation, which enabled fast electron transport; also, the electron collection by GO inhibited the recombination of charge carriers.169 However, the oxygen-containing groups on the rGO sheets acted as recombination centres, which affected the PC activity. To overcome this and to obtain good interfacial contact, Mou et al. deposited TiO2 onto N-doped graphene (NGR) and achieved enhanced visible light photocatalytic hydrogen generation.170 The rGO/g-C3N4-TiO2 ternary hybrid layered photocatalyst showed an increased H2 yield of 23
143 μmol g−1 h−1, which was attributed to the visible light activity and decreased recombination of charge carriers by rGO.171 The doped nitrogen provided positive sites for the incorporation of TiO2 nanocrystals on the NGR sheets and created close interfacial contacts between the TiO2 nanoparticles and NGR. Further, NGR was found to have superior electrical conductivity compared to that of reduced graphene oxide (rGO), which was attributed to the recovery of the sp2 graphite network and decreased defects,172 leading to effectual charge separation and charge transfer in NGR/TiO2. The strong coupling between NGR and TiO2 increased the interfacial connection of the two moieties for better electron separation and transfer, as shown in Fig. 20.
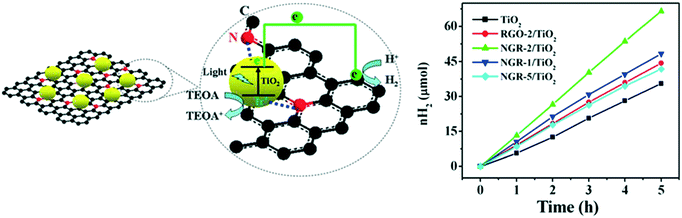 |
| Fig. 20 Schematic of the increased charge transfer in TiO2 and NGR sheets. Reprinted with permission from ref. 144. Copyright 2014 ACS. | |
4.4 Effect of both cations and anions as dopants
4.4.1 Ga, In and La as Co-dopants for N-doped TiO2.
Co-doping of TiO2 with both cationic and anionic species appropriately results in a significant extension of the absorption range of TiO2 into the visible region. Li et al. used Ga and N as co-dopants to passivate the carrier recombination centers (inside TiO2) generated by mono-doping.173–175 Similarly, they enhanced the doping concentrations by the columbic attraction between the oppositely charged dopants.176–178 In these systems, in addition to the introduction of oxygen vacancies, the new N 2p band of the N dopant formed over the O 2p states, where the N impurities were found to associate with Ti3+ and decrease the band gap energy of N-doped TiO2. Gallium was introduced as Ga3+ to enhance the oxygen vacancies introduced by the hole doping of N, which was found to be p-type doping.90,174,175,179–187 Therefore, the TiO2 co-doping led to synergetic band gap realignment and good response to visible light for efficient hydrogen evolution.
Sasikala et al. compared the effects of In-N co-doped-TiO2 to those of In or N-doped TiO2. The In-N co-doped TiO2 showed decreased band gap energy, which was attributed to the addition of the 5s, 5p orbitals of In to the CB and N 2p orbitals in the VB. As a result, the hydrogen evolution was found to increase to 14 μmol/12 h for the co-doped sample and 07 μmol/12 h for the mono-doped TiO2 sample.188 Fanke Meng et al. reported the co-doping of nitrogen into La2Ti2O7 (LTO) nanosheets synthesized by a hydrothermal process; they showed visible-light absorption up to 560 nm and enhanced solar light-mediated photocatalytic hydrogen generation.189 Table S5 in the ESI† presents a list of recently reported doped photocatalysts for hydrogen production.
5. Composite systems
5.1 Carbon-based TiO2 composites
Parayil et al. synthesized TiO2-carbon hybrids using 2,3-dihydroxynaphthalene and TiO2 by a ligand to-metal charge transfer complexation process. It was observed that the carbonization under N2 pyrolysis modified the physical properties of TiO2 by increasing the surface area from 6.5 m2 g−1 to 12.5 m2 g−1 and resulted in fluorescence quenching of the TiO2 emission. A noticeable improvement in hydrogen evolution of 2.6 μmol g−1 h−1 was observed; this was attributed to the C on the TiO2 surface, which efficiently transported electrons190 and improved the photocatalytic proton reduction to produce hydrogen.191 Further, it was reported that carbon-modified TiO2 composite materials showed an improvement from no H2 evolution to 0.21 mL g−1 h−1 with a carbon loading of 3.62%. Parayil et al. suggested that the interaction of carbon with rutile TiO2 facilitated the superficial trapping of electrons in the oxygen vacancies and minimized the charge carrier recombination.192 A similar study reported that decoration of a thin carbon layer (0.3%) on TiO2 acted as an electron trapping center and thereby enhanced charge carrier separation and achieved four-fold improvement compared to the pristine TiO2 nanoparticles.193 Similarly, brookite TiO2 (br-TiO2) integrated with g-C3N4 exhibited enhanced photocatalytic activity due to the more negative CB edge position of g-C3N4 with respect to that of br-TiO2. This chemical bonding of the br-TiO2/g-C3N4 hybrid allowed the rapid movement of electrons from the CB of g-C3N4 to the CB of br-TiO2.194
TiO2 with carbon fiber (CF) and CuO as a co-catalyst was found to show enhanced activity due to the strong electronic conductivity of CF, which significantly decreased the charge transfer resistance in the material. Higher activity was found in the 1 wt% CuO/CF/TiO2 system, where CuO enhanced the electron conductivity and largely decreased the recombination possibilities in the system.195 Similarly, a TiO2 photocatalyst loaded with 5 wt% CuS–5 wt% NiS as co-catalysts showed high photocatalytic H2 evolution of 800 μmol h−1 g−1, which was around twenty-eight times higher than that of TiO2. The dual NiS–CuS co-catalysts rapidly accepted the excited electrons from the conduction band of TiO2, thereby creating more surface active sites for H2 evolution to take place.196
In another study, graphene and Cu were used as co-catalysts to synergistically enhance the photocatalytic activity of TiO2; the system showed 5 times more hydrogen generation than the pristine graphene. In this system, Cu loading shifted the absorption peak towards longer wavelengths, which is attributed to the formation of new energy levels in the band structure of TiO2. However, it was found that at increased concentrations, the co-catalysts began to function as recombination centers; hence, the optimum concentration of the cocatalyst was proposed for higher photocatalytic activity.197
Graphene based-TiO2 composites.
Reduced graphene oxide-TiO2 hybrids show substantial increments in H2 evolution due to the enhanced mobility of electrons and decreased recombination. The photogenerated electrons can efficiently migrate from the CB of TiO2 to graphene because the CB minimum of TiO2 is higher than the Fermi level of graphene; this facilitates enhanced photocatalytic activity.198 Cheng et al. claimed that graphene acts as a electron acceptor due to its two-dimensional π-conjugated structure;199 their nanocomposite showed hydrogen evolution up to 668 μmol h−1 g−1, which was increased by 68% compared to that of P25-TiO2 (397 μmol h−1 g−1) loaded with 0.5 wt% Pt.
Interestingly, Ag nanoparticles were assembled onto the surface of TiO2–graphene nanocomposite, as shown in Fig. 21; this system showed increased light absorption and more competent separation of charge carriers compared to the bare TiO2–graphene nanocomposite. The enhanced performance was mainly attributed to the surface plasmon resonance of the Ag NPs, which led to enhanced visible light absorption, longer lifetimes of the excited electron–hole pairs and rapid charge transport in the system; this resulted in the improved photoactivity of the nanocomposite.200
 |
| Fig. 21 (A) TEM images of TiO2 and graphene; (B) Ag–TiO2–graphene. Reprinted with permission from ref. 171. Copyright 2014 Elsevier. | |
CNT-TiO2 composites.
Multi-walled carbon nanotubes (MWCNTs) show higher surface areas when they are combined with nanostructured metal oxides such as TiO2 and ZnO; they significantly improve the charge transportation and optical and electrical properties of metal oxides.201,202 Eventually, the MWCNTs-TiO2 composite nanostructures create numerous active sites for the photocatalytic reactions. For example, the functionalized MWCNT-TiO2 nanocomposites showed improved surface electric charge and led to stronger electronic transitions in the composite. Further, the MWCNTs acted as photosensitizers and more actively absorbed visible light and effectively transported charge carriers to the surface of TiO2; this significantly improved the H2 generation in the system, as shown in Fig. 22, and electron microscopy images of the prepared nanocomposites are shown in Fig. 23. This MWCNTs–TiO2 nanocomposite showed enhanced hydrogen production of 8 mmol g−1 h−1 due to the synergistic interaction between MWCNTs and TiO2.203
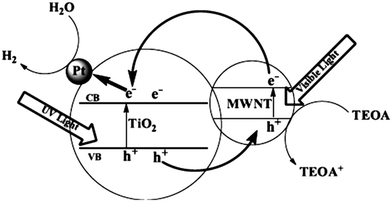 |
| Fig. 22 Schematic of the photocatalytic mechanism of MWNT–TiO2. Reprinted with permission from ref. 174. Copyright 2009 IOP. | |
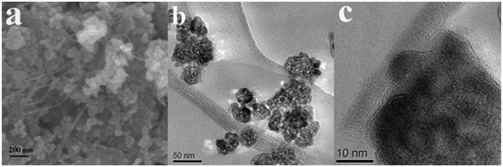 |
| Fig. 23 SEM (a) and HRTEM (b and c) images of the MWNT–TiO2 nanocomposite. Reprinted with permission from ref. 174. Copyright 2009 IOP. | |
Similarly, Zhang et al. produced a significant amount of hydrogen (37.6 mmol h−1 g−1) and achieved a high apparent quantum efficiency (over 95%) by constructing carbon/TiO2/carbon nanotube (CTCNT) hybrid structures,204 and similar CTCNT structures showed red shifts in their optical properties;205,206 this was attributed to the effects of non-stoichiometricity in TiO2 due to the formation of oxygen vacancies and Ti3+ species. The nanocomposite possessed decreased band gap energy, as shown in Fig. 24, and the smallest gap between the band tail ends was anticipated to be about 0.88 eV.
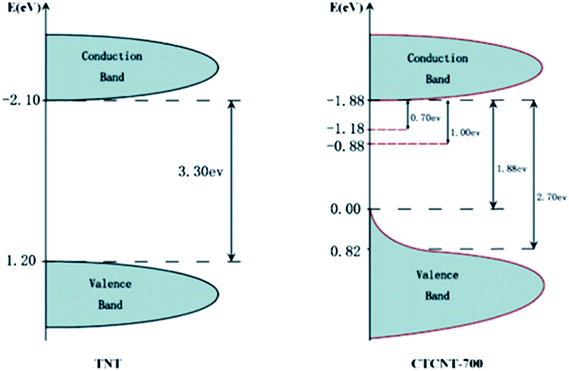 |
| Fig. 24 DOS of pure TNT and CTCNT-700. Reprinted with permission from ref. 175. Copyright 2014 RSC. | |
On the other hand, semiconducting SWCNT (s-SWCNT), as shown in Fig. 25, displayed better photocatalytic activity compared to metallic single-walled carbon nanotubes (m-SWCNTs) when combined with a TiO2 photocatalyst. The higher permissible transitions from the van Hove singularities of s-SWCNTs are realized to be more beneficial for charge transfer in s-SWCNTs compared to SWCNTs. Also, the van Hove singularities were found to act as mid-gap bands and cause the generation of multi-excitons under UV light.207
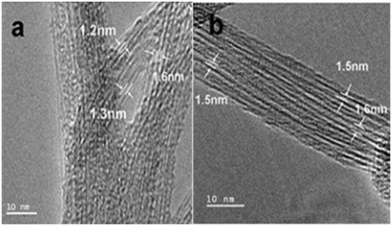 |
| Fig. 25 HRTEM images of SWCNTs. (a) Pristine SWCNTs synthesized in pure He, C0 and (b) the pristine SWCNTs synthesized in 25 vol% CO2, C25. Reprinted with permission from ref. 207. Copyright 2011 Elsevier. | |
5.2 Metal oxide-based composites
Copper oxide-TiO2 composites.
Due to the high cost of noble metals, researchers are focusing more on non-noble metals, such as Cu and Ni, either as dopants or oxides to form composite materials with enhanced properties.208–210 In this direction, composites of Cu2O and CuO (with narrow band gap energies of 1.7 eV and 2.0 eV, respectively) with TiO2 afforded visible light-active photocatalytic systems for hydrogen evolution.208 Accordingly, the CuO/TiO2 composite showed enhanced performance towards hydrogen generation under visible light211–213 because of its significant advantages in photon harvesting effectiveness due to the effective charge carrier separation through interfacial charge transfer.214–217 It was found that the synthesis methods play important roles in the formation of these nanomaterials. Accordingly, wet chemical methods mostly favor formation of interfacial copper oxide–TiO2 composites, while techniques such as sputtering create Cu/TiO2 systems.218 However, in all these cases, the absorption spectra display significant red shifts (up to 700 nm) and extended absorption (400 and 800 nm) of visible light due to the presence of Cu species. It should be noted that different Cu species, such as metallic copper [225 to 510 nm], 3p of Cu2+ clusters [44 to 500 nm], and CuO excite electrons from the VB to the exciton level [<330 nm], and the d-d transitions of Cu2+ [600 to 800 nm] display different optical activities in different regions of the UV-visible window.219,220
Interestingly, the Cu doping obtained by Wang et al. using RF reactive magnetron sputtering was found to modify the orientation of TiO2 from 101 to 001, where the 001 facets were found to be more active because of their higher surface energy;218 water molecules can easily dissociate on the 001 facets, which is beneficial for hydrogen generation. They further proposed that the presence of Cu1+ can trap photoexcited electrons for effective electron–hole separation. Yu et al. reported the preparation of Cu2+-doped mesoporous TiO2 by wet impregnation, where they revealed that the doped Cu2+ ions facilitated the creation of oxygen vacancies in TiO2. Accordingly, Besenbacher's group reported the dissociation of adsorbed H2O by photon transport into closer oxygen atoms through surface oxygen vacancies in a Cu/TiO2 system.221 CuS nanoflower/TiO2 nanospindle heterostructured nanocomposites showed H2 generation of 1262 μmol h−1 g−1.222 Copper-supported TiO2 and zirconium titanate were evaluated for H2 generation, and the presence of additional metallic copper during H2 evolution was detected through EPR analysis.223 Fluorinated TiO2 powder with Cu (NO3)2·H2O forms crystalline and mesoporous materials where copper exists in the form of CuO, resulting in four times greater activity than TiO2 and a fluorinated TiO2 support.224 Xu et al. incorporated CuO particles by impregnation/adsorption/calcination methods on TiO2 nanotubes (TNTs) prepared by a hydrothermal process.215 The obtained 1D tubular structures of TiO2 were found to exhibit superior electron transfer compared to that of Pt/Ni-incorporated TNTs.225 In addition, the larger surface area of the CuO–TNT nanocomposites provided active sites for reduction of protons to obtain hydrogen evolution of up to 20 mmol h−1 g−1. The calcination method was found to be superior to wet chemical impregnation and adsorption to generate a highly dispersed active CuO–TNTs photocatalyst.
Yu et al. reported hydrogen evolution of 3418 μmol h−1 g−1 with a quantum efficiency of 13.9% in a Cu(OH)2/TiO2 system, which was attributed to its enhanced charge separation and transportation, as depicted in Fig. 26.226 Similarly, Zhang et al. used TiO2 nanotube arrays (TNAs)217 instead of titania particles to form a similar interface and studied its hydrogen evolution properties.
 |
| Fig. 26 (a) and (b) Representations of charge carrier separation and transfer in a Cu(OH)2–TiO2 system. Reprinted with permission from ref. 191. Copyright 2011 RSC. | |
The common mechanism199–226 of water splitting that occurs on these interfaces is depicted in Fig. 27.213 The photoexcited electrons migrate to the CB of CuO through the CB of TiO2, which is lower than the CB of TiO2 (−0.26 eV), in contrast with O2 electron transfer. Subsequently, the transferred electrons cause partial reduction of Cu2+ to Cu0 and finally form Cu clusters that act as co-catalysts to trap electrons from TiO2, promote separation, and enable H2 production. The accumulated electrons on CuO lead to a shift of the CB potential towards a more negative value, which supports inter-particle electron transfer from CuO to the trapped states of TiO2 and results in Fermi level equilibration. The potential of CuO is −0.224 eV; hence, it can reduce protons efficiently (E0H+/H2 = 0 vs. SHE pH = 0), as suggested by Lee et al.213 in 2010 through the decreased PL intensity, indicating greatly decreased recombination possibilities. Rawool et al. studied lab scale optimization of the photocatalyst Cu0.02Ti0.98O2−δ with an apparent quantum efficiency of 7.5%. The relative standard deviation was studied, with higher deviation of the H2 yield on cloudy days (±3.82%) compared to sunny days (±4.53%).227
 |
| Fig. 27 Mechanism of H2 generation from TiO2/CuO. Reprinted with permission from ref. 189. Copyright 2013 Elsevier. | |
In another case, Xiong et al.228 reported that Cu2O produces charge carriers under visible light irradiation and that when it interfaces with titania, the Ti4+ ions capture electrons and are reduced to Ti3+; these Ti3+ cations can sufficiently improve photocatalytic activity under visible light, as discussed earlier in this review. It was observed that the concentration of CuO is crucial for these interfacial reactions, and monolayer deposition of CuO was reported to be optimal for improved interfacial reactions with TiO2. It was reported176 that increasing the mole ratio of Cu(OH)2 to TiO2 from 1 to 7 significantly reduces the hydrogen evolution from 3718 to 87.1; this was attributed to the screening effect of Cu(OH)2 or electron transfer to the valence band of TiO2, which induces recombination along with CuO reduction to Cu+1 and Cu0. It was also reported that the excess CuO can scavenge electrons229 to form Cu+1 and Cu0, as given in eqn (3) and (4).
| Cu2+ + e− → Cu+, E0 = 0.153 eV | (3) |
| Cu2+ + 2e− → Cu, E0 = 0.341 eV | (4) |
ZnO–TiO2 composites.
ZnO–TiO2 composites are being used for hydrogen generation; they also show enhanced hydrogen generation abilities under UV and visible light irradiation.230–232 Guo et al.230 reported a ZnO–TiO2 microporous composite with a crystalline/amorphous heterostructure (with a large surface area of 311.9 m2 g−1) and a tunable atomic arrangement, which led to facile adsorption and rapid surface reactions in the system. Additionally, the different CB positions of ZnO and TiO2 were found to drive the charge carriers from ZnO to TiO2. This was also verified by Wang et al.,183 who explained the charge transport and separation as shown in Fig. 28.233 The CBs of TiO2 and ZnO are at −4.15 and −4.21 eV, respectively, with respect to Evac. On the other hand, the stability of Zn nanorod arrays was improved by intercalation with the (001) facets of titania sheets, and better charge separation and transport were also achieved in this system. Similarly, a mixed oxide of TiO2–ZnO was prepared by Pérez-Larios et al.; they observed six-fold improved hydrogen evolution (1300 μmol h−1 g−1) compared than that of the pristine TiO2 (190 μmol h−1 g−1).231
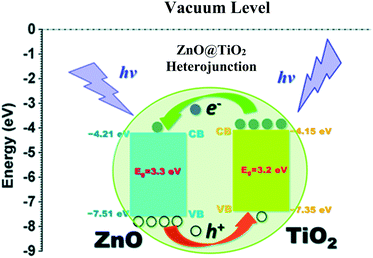 |
| Fig. 28 Energy levels of the heterojunction of ZnO–TiO2. Reprinted with permission from ref. 216. Copyright 2014 RSC. | |
Barakat et al. decreased the band gap energy of titanium oxide nanofibers to 1.89 eV by incorporating Fe2O3 and ZnO and observed effective hydrogen generation (an exceptional value of 0.12 ml min−1 mg−1 cat) under visible light radiation.232 Hematite (Fe2O3) possesses a narrow band gap energy (2.2 15 eV);234,235 however, it shows poor absorptivity,236 very short exciton lifetimes (∼10 to 12 s),237 decreased oxygen evolution,238 and a smaller hole diffusion length (2 to 4 nm),239 and thus is generally coupled with other metal oxides. Accordingly, ZnO and Fe2O3 were dispersed in the matrix of TiO2 fibers, where it was found that p–d repulsion (e.g., O 2p–Zn 3d) shifted the VB maximum uphill in the semiconductor without altering the CB minimum, which led to a band gap decrease, as shown in Fig. 29.240 The charge separation, transport and enhanced visible light absorption of the system can be understood from Fig. 30(a). Further, the IV characterization, as shown in Fig. 30(b), indicated a linear increase with the applied voltage, indicating high electron transfer of the nanofiber composites.
 |
| Fig. 29 TiO2, ZnO and Fe2O3 band gap positions. Reprinted with permission from ref. 203. Copyright 2005 ACS. | |
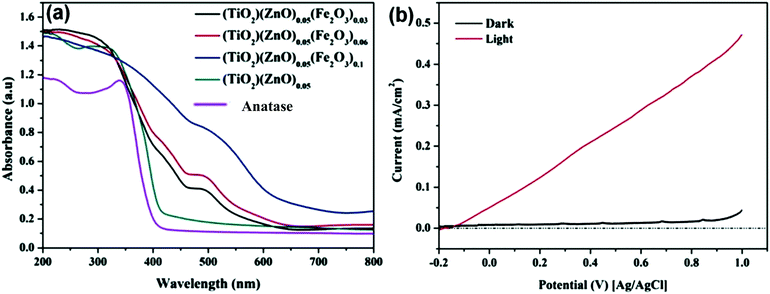 |
| Fig. 30 (a) UV-absorption spectra of TiO2/ZnO/Fe2O3; (b) IV characterization of a thin film of TiZn0.05Fe0.06O2.14 nanofibers. Reprinted with permission from ACS ref. 203. | |
ZrO2–TiO2 composites.
ZrO2 has been found to be a potential semiconductor; while its large band gap of 5.0 eV largely limits its application, it can be used in a mixed oxide with TiO2.191 Accordingly, Hussein et al. synthesized mesoporous ZrO2 on ZnO/TiO2 nanocomposites (prepared by a surfactant-assisted sol–gel method) and showed improved H2 generation. Specifically, it was found that 0.5 wt% ZrO2 produced 30.78 μmol h−1 g−1, while 3.55 μmol h−1 g−1 was obtained for a ZnO/TiO2 composite.241 They claimed that TiO2, ZnO, and ZrO2 possess suitable band structures and satisfy the thermodynamic requirements to overcome the reductive and oxidative potentials for water splitting. As per studies by Navío et al.,242 the electrons in the CB of ZrO2 are more negative and the holes in the VB are more positive compared to the reduction and oxidation potentials of H2/H2O and O2/H2O, respectively, resulting in a higher driving force for H2 production. Further, the observed enhanced H2 production by the system was attributed to the multiple absorbance thresholds evidenced at 435 nm and 417 nm, corresponding to band gap energies of 2.85 eV and 2.97 eV, together with its high surface area, large pore volumes, uniform crystallite sizes, enhanced light harvesting and charge separation. Surakerk Onsuratooma et al. loaded Ag onto TiO2–ZrO2, which showed enhanced photocatalytic H2 evolution in the presence of UV light. The TiO2–ZrO2 (93
:
7) mixed oxide nanocrystal photocatalyst showed enhanced H2 generation that increased further with 0.5 wt% Ag loading. Hydrogen generation rates of 1.18 cm3 h−1 cat and 0.51 cm3 h−1 cat were obtained for the 0.93 TiO2–0.07 ZrO2 mixed and pure TiO2 oxides, respectively.243 This study claimed that the surface properties of the ZrO2–TiO2 composite are responsible for its enhanced activity rather than their electronic structures.
5.3 Heterojunction formation with TiO2
Semiconductor junctions of various metals and oxides with TiO2 promote electron mobility, charge separation and visible light absorption if their energy levels are favorably aligned. Accordingly, Shwetharani et al. reported an Fe2O3-coupled TiO2 system and achieved well-matched band alignment for effective transfer of energy.244 In this study, it was found that the Fe in Fe2O3 acted as an electron acceptor due to its potential of 2.4 eV and the Fe3+ ions served as shallow traps in TiO2; this resulted in decreased recombination, and improved H2 evolution up to 850 μmoles/40 min was thus obtained. It was also proposed that the mid-bands created by dopant Fe3+/defects/oxygen vacancies in titania led to alignment of the band edges in the synthesized heterojunctions.
Vinothkumar et al. formed a ternary heterojunctioned titanium dioxide–indium oxide–copper oxide catalyst by co-precipitation; they achieved enhanced hydrogen evolution of 1829 μmol g−1 h−1 for the binary catalyst and 2149 μmol g−1 h−1 for the ternary catalyst.245 The visible light absorption and red shift in the ternary oxide was due to copper ions,246,247 and the band gap for the ternary oxide was measured to be 2.01 eV. The more negative CB potentials of Cu2O and CuO (ref. 248 and 249) allowed mobility of electrons from Cu2O/CuO to In2O3 followed by TiO2, as shown in Fig. 31.
 |
| Fig. 31 Band structure of Cu2O/In2O3/TiO2. Reprinted with permission from ref. 208. Copyright 2014 Elsevier. | |
Tantalum oxide is completely inactive for hydrogen generation; however, heterojunctions of Ta2O5–TiO2 with mesoporous structures and relatively high surface areas (∼130 m2 g−1) showed a much lower bandgap than pristine250 Ta2O5 (bandgap ∼4.0 eV) and showed good activity for H2 evolution.251 Wu et al. prepared TiO2–SiO2 composite materials via a sol–gel method; this led to the emergence of different amounts of Ti4+ ions and different textural properties. Among the series of composites, the hydrothermally synthesized system was found to produce the most hydrogen (1.35 mmol h−1 g−1). The observed higher activity of the hydrothermally synthesized system was attributed to (i) the increased number of Ti4+ ions in tetrahedral coordination, which shifts the CB towards a more negative potential and facilitates effective production of H2, (ii) decreased particle sizes and (iii) non-radiative energy transfer.252 Further, Rungjaroentawon et al. sensitized a TiO2–SiO2 mixed oxide with eosin-y on a mesoporous-assembled structure and observed enhanced activity.253
Hu et al. reported that a Ni/NiO/N-TiO2−x heterojunction exhibits improved H2 production (1210 μmol h−1 g−1) with an apparent quantum yield of 7.5%, which is 250 times higher than that of the pristine TiO2. The Ni in the system decreased the surface free energy of the nanocomposites, thereby decreasing the thermodynamic driving force for particle growth and leading to the formation of smaller particles. NH3 plasma treatment was found to reduce partial NiO to metallic Ni, which led to the formation of higher concentrations of oxygen vacancies; thus, improved charge separation was observed. Because the potential of Ni2+/Ni (Ni2+ + 2e− = Ni, −0.23 V) is lower than the CB (−0.26 V) of TiO2 and higher than the reduction potential of H+/H2 (2H+ + 2e− = H2, 0.00 V),24 the photoinduced electrons in the CB of TiO2 transferred to the Ni/NiO clusters; thus, metallic Ni acted as a cocatalyst. Furthermore, the valence band electrons of the R-Ni(x)N(y)Ti series catalysts can be excited to the oxygen vacancies; the electrons are then transported from the oxygen vacancies to the CB by absorbing other photons and finally to the Ni/NiO clusters, leading to more effective visible light utilization.254 The Bi2O3–TiO2 composite photocatalysts showed an H2 evolution rate of 920 μmol h−1 g−1, exceeding that of pure TiO2 by 73 times, with an apparent quantum efficiency (QE) of 3.7%. The improved activity is due to the shift of the CB towards more negative potential, which is attributed to energy level splitting.255
Yan et al. reported the formation of p–n heterojunction Pt–Sr(Zr1−xYx)O3−δ–TiO2, which showed increased photocatalytic activity of H2 evolution. When the composite was irradiated, effective charge separation and transportation occurred due to the heterojunction of the p-type Sr(Zr1−xYx)O3−δ and n-type TiO2 semiconductors and due to the presence of Pt in the system.256 Li et al. formed composites of SiC (1 wt%)–NiOx/TiO2 (three-component) and four-component IrO2/SiC (1 wt%)–NiOx/TiO2 materials that evolved 30% and 100% more H2, respectively, than the NiOx/TiO2 catalysts. NiOx/TiO2 was not found to be active under visible light irradiation, while SiC (1 wt%)–NiOx/TiO2 was active because of the narrow bandgap energy of SiC. NiOx acted as electron collectors and as active sites for hydrogen evolution, as shown in Fig. 32.257
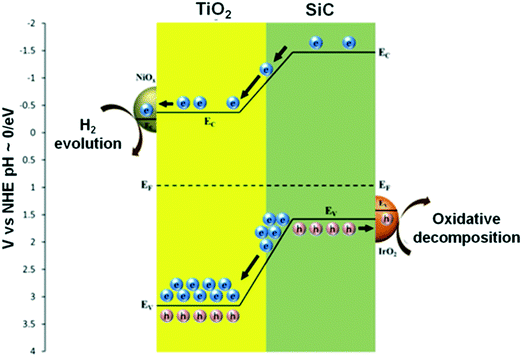 |
| Fig. 32 Schematic of band energy alignment in IrO2/SiC-NiOx/TiO2. Reprinted with permission from ref. 218. Copyright 2013 Elsevier. | |
Fan et al. synthesized mesoporous TiO2-pillared titanoniobate (TiO2/HTiNbO5) with a surface area of 171 m2 g−1; it exhibited enhanced hydrogen evolution of 219 μmol h−1, which was about 12 times that of HTiNbO5 (protonic titanoniobate) (18 μmol h−1 g−1) and 24 times that of TiO2 (9 μmol h−1 g−1). The mechanism of decreased recombination in the heterojunction and the band gaps and band edges of this heterojunction are displayed in Fig. 33. It was proposed that band bending by the internal electric field and effective separation of charge carriers resulted in the higher photocatalytic activity. Further, 5 wt% loading of Pt was found to increase the hydrogen evolution to 4735 μmol h−1 g−1.258
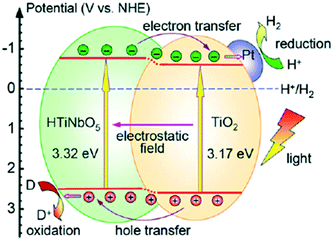 |
| Fig. 33 Schematic of photoexcitation in a TiO2/HTiNbO5 semiconductor–semiconductor pillared photocatalyst. Reprinted with permission from ref. 219. Copyright 2013 Elsevier. | |
5.4 Sulfide-TiO2 composites
Water splitting by pristine sulphide-based semiconductors is not possible because their VBs contain S 2p energy levels; sulphide-based photocatalysts also suffer from photocorrosion during the photocatalytic process. During the reaction, instead of H2O, the S2− in CdS tends be to oxidized by the photogenerated holes with elution of Cd2+, as represented in CdS + 2h+ → Cd2+ + S,208,209 which is the major disadvantage of metal sulfide semiconductors. Therefore, sacrificial reagents (such as Na2S and Na2SO3) are required to attain photocatalytic hydrogen generation, which is not an ideal solution for enhancement of stability in sulphides. In this context, the incorporation of metal sulfides into titania has been studied extensively, which has resulted in enhanced photocatalytic activities and efficient resistance to photocorrosion. Consequently, metal sulphides such as CuS-loaded TiO2 (ref. 259) and CdS/TiO2 (ref. 260) have been developed and have achieved enhanced photocatalytic hydrogen evolution properties. Because sulphide semiconductors consist of d and sp orbitals, where the S 3p orbital is more negative than the O 2p orbitals, their CB potential is sufficient to reduce H2O to H2.29 For example, Jia et al.301 reported that TiO2−xSx nanotube-films coupled with ZnS–In2S3–Ag2S solid solution showed improved visible light absorption up to 800 nm; the sulfur doping substitutes O in the TiO2 lattice, which creates a mid-band because of the S 3p orbital. This leads to a decrease in the band gap energy and shifting of the flat band potential to a higher level; this results in improved electron transfer in the system and, therefore, improved photocatalytic activity.213 CdS-Mn/MoS2/CdTe/TiO2 quaternary photocatalyst showed an evolution of 10.165 mL cm−2 h−1. The higher photocatalytic activity is due to synergetic effects of the CdS–Mn, MoS2, CdTe and TiO2 nanophase structures.261
5.5 Phosphate-TiO2 composites
Based on a series of previous reports on phosphate groups,262,263 Luan et al. functionally modified polyhydric phosphates on the surface of nanocrystalline α-Fe2O3 through a wet-chemical process and then combined it with TiO2. The phosphate groups acted as “electronic bridges” between TiO2 and α-Fe2O3 which were found to be a medium to facilitate charge transportation, as shown in Fig. 34.264 They demonstrated that the lifetimes and separation of excitons in the TiO2–Fe2O3 nanocomposite were greatly enhanced, and hydrogen evolution doubled upon the introduction of phosphate bridges.265
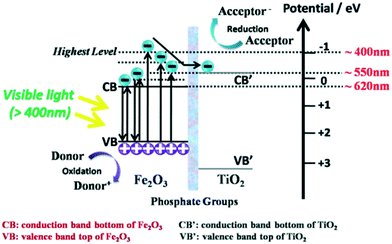 |
| Fig. 34 Charge carrier transfer and separation in phosphate-bridged TiO2–Fe2O3 composite. Reprinted with permission from ref. 264. Copyright 2015 RSC. | |
Similarly, Xie et al. reported that a phosphate-bridged TiO2–BiVO4 nanocomposite showed enhanced photocatalytic activities for water oxidation and H2 evolution which were found to be about three times higher than those of the un-bridged TiO2–BiVO4.266 Table S6 in the ESI† shows a list of TiO2-based composites for H2 generation.
6. Co-catalyst/plasmonic metal supports
Metals such as Rh, Pt, Pd, Ag, Cu and Au have been extensively utilized as supporting carrier materials for TiO2 towards photocatalytic water splitting. Kudo et al. reported that if the necessary active sites are not present on the surface for redox reactions, the probability of electron–hole recombination is strong.267–271 Therefore, the introduction of a co-catalyst largely creates and/or facilitates active sites on the TiO2. As a result, due to the CB level of TiO2 and the work functions of these metals, back reactions are largely suppressed and hydrogen generation occurs efficiently, as shown in Fig. 35.223
 |
| Fig. 35 Mechanism of photosensitization of TiO2 with Au nanoparticles. Reprinted with permission from ref. 223. Copyright 2013 RSC. | |
6.1 Pt-Supported TiO2
Honda et al. demonstrated in 1983 that Pt can serve as an active site for hydrogen production on Pt–TiO2.272 In these systems, the accumulation of electrons in the metal particles shifts the Fermi level closer to the CB level of TiO2, resulting in higher negative energy levels. It was found that smaller noble metal particles display more negative shifts in the Fermi level. In general, noble metals possess lower Fermi levels compared to titania; therefore, electron transport is greatly facilitated from titania to the noble metal. This process subsequently adsorbs protons on the surface and reduces them to hydrogen, as depicted in Fig. 36.225,273 Because the work function of Pt is highest, the electron trapping ability of Pt is the strongest. Zielińska-Jurek et al. reported the order of Pt, Au and Ag-supported photocatalytic activity; it was found to be Pt > Au > Ag.274 Pt also possesses the lowest activation energy for proton reduction; hence, it has been used as an excellent co-catalyst for a range of photocatalysts. The electron structure of Pt-modified TiO2 surfaces was mapped66,275 and the dynamics of charge transfer from Pt to TiO2 were also studied using UPS measurements.276 Hainer et al. investigated the effects of sacrificial agents on true water splitting; they reported higher H2 production in the presence of sacrificial agents such as methanol and formic acid. Additionally, they claimed that earth-abundant Cu shows competitive catalytic activity with noble metal ions, such as Pt, Pd and Au.277 Pd and WO3 supported on bare TiO2 nanotubes showed a maximum quantum efficiency of 7.7% using an aqueous solution of methanol (50 vol%).278 Pt and CoOx dual cocatalyst-coated TiO2 showed hydrogen generation of 275.9 μmol h−1 g−1 compared to 56.5 μmol h−1 g−1 for TiO2; this was attributed to precise charge carrier separation using Pt for electron separation and CoOx for hole separation.279
 |
| Fig. 36 Transmission electron microscopic images of (a and b) Pt–TiO2, (c and d) Au–TiO2 and (e and f) Au/Pt–TiO2. Reprinted with permission from ref. 232. Copyright 2016 Nature. | |
It was reported that Pt loading can enhance the separation of photoexcited charge carriers through the formation of Schottky energy barriers.280 Serrano et al. experimented with various amounts of metallic Pt deposition using different impregnation methods and found that the material prepared by the “citrate method”, based on reduction and encapsulation with sodium citrate, provided very good dispersion of Pt onto the TiO2. The Pt nanoparticles were deposited and reduced preferentially within the pores of m-TiO2, leading to stronger interactions and better dispersion than the other two explored dispersion routes (wet impregnation and photodeposition). Although it involved a lower concentration of Pt (0.78%) than the other two dispersion routes (2%), the citrate method produced close to twice the amount of hydrogen. Furthermore, the production capacity was also found to be higher when the amount of Pt loading was increased, with the optimal concentration being determined as 2% (w/w). When platinum was deposited either by incipient-wet impregnation or photodeposition, the delay to reach the stationary regime was longer compared to when the photocatalyst was prepared by the citrate method.281
Wei et al. reported that Pt-modified TiO2 microspheres synthesized by an impregnation–reduction process showed remarkable photocatalytic activity by loading an optimal amount (1.2 wt%) of Pt; they exhibited about 125 times higher H2 production than unmodified TiO2 microspheres.282 Fang et al. reported that Pt rapidly traps the photoexcited electrons and reduces H+ to generate H2, whereas the holes can be scavenged by electron donors, namely sacrificial agents; this results in effective inhibition of recombination of charge carriers and increased photocatalytic hydrogen production. In addition, it was found that the charge recombination was suppressed depending upon on the concentration of Pt onto the TiO2.283 However, higher metal dispersion led to screening effects, which significantly decreased the photocatalytic activity due to the limited access of TiO2 to light. Nguyen et al. reported that TiO2 nanotubes with a mesh of TiO2 nanofibrils along with Pt showed enhanced hydrogen evolution compared to the conventional Pt decoration technique. This is essentially because the Pt nanoparticles were able to efficiently trap the conduction band electrons and mediate their transfer to the liquid phase.284 Conversely, Pt modification on rutile TiO2 showed lower performance in acidic and basic conditions due to photo-corrosion effects.285 Wu et al. formulated a Pt/N-TiO2 system and observed twice the amount of hydrogen production compared to Pd/N-TiO2,286 mainly due to rapid electron transfer from Pt to TiO2.
6.2 Au/Ag-Supported TiO2
It has been demonstrated that the electrons transferred from the CB of TiO2 can locate themselves on Au NPs; this creates a Schottky barrier, which significantly decreases the charge recombination and leads to stabilization of the charge carriers.287,288 A variety of Au/TiO2 systems289 have shown enhanced photocatalytic effects.241–290 For example, Au/TiO2 showed enhanced H2 generation of 32.4 μmol h−1 g−1 under visible light irradiation and displayed improved plasmonic effects. The impinging photons on gold nanoparticles produce a surface plasmon resonance (SPR) effect that largely augments the production of e−/h+ pairs by enhancing291 the visible light absorption and effectively transfers them to the surface of photocatalyst for efficient photocatalytic reactions.292 Tanaka et al.302 compared various metal loadings (Pt, Ag or Cu) onto Au/TiO2 and pristine TiO2 photocatalysts. An optimal concentration of 1 wt% Au loading displayed improved H2 generation due to the unique metal/oxide heterojunctions and dispersion of the gold particles.243 A hierarchical porous Au-decorated-N-doped TiO2 photocatalyst showed visible light activity, which was attributed to the synergetic effects of N doping and mesoporosity for increased specific surface area and effective charge transfer, resulting in improved H2 evolution.293 TiO2 photocatalysts with high Au loading and large Au nanoparticle sizes (≈50 nm) produced 5.3 mmol g−1 cat h−1 of H2 from the gas phase reaction of water and methanol.294
Similarly, Ag nanoparticles295 also exhibit surface plasmon resonance effects. Yang et al. deposited Ag NPs onto a TiO2 film, as shown in Fig. 37; they observed that the Ag nanoparticles exhibited strong SPR effects, leading to stronger visible light absorption, which facilitated the communal oscillation of conduction/surface electrons and exhibited enhanced H2 production of 7.2 μmol·cm−2. This was ascribed to the synergistic effects between surface plasmon resonance and charge transfer in the Ag–TiO2 system.296
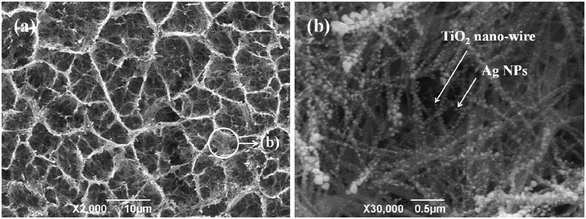 |
| Fig. 37 SEM images of a Ag/TiO2 nanowire film. (a) Ag/TiO2 nanowire film and (b) the enlarged image of (a). Reprinted with permission from ref. 245. Copyright 2014 Trans Tech. | |
Wu et al. deposited Ag NPs on TiO2 nanotube arrays (TiO2 NTs), as shown in Fig. 38; they achieved enhanced surface plasmon resonance effects and restrained recombination in the TiO2 photocatalyst.297 Mixed metal loading or loading of alloys has also been performed, but by very few groups.298,299 For example, loading of Au–Pt nanoalloy onto TiO2 was reported by Montes-Navajas et al.; they observed that the system was more proficient in enhancing the activity of TiO2 than loading of the individual metals onto the TiO2.300 The observed efficiency was attributed to the added effects of the bimetals, such as more active centers and better electron separation and transfer. Table S7† shows a list of noble metal-loaded TiO2 photocatalysts.
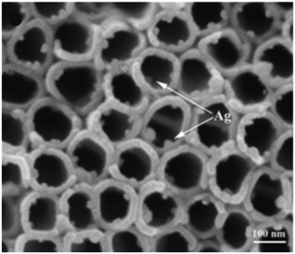 |
| Fig. 38 Ag–TiO2 nanotubes. Reprinted with permission from ref. 246. Copyright 2013 springer. | |
7. Conclusions and future perspectives
TiO2 is being widely explored for its functional properties; this research is still in progress in various fields. Especially in the field of energy, TiO2 has been largely studied for applications in photocatalysis, solar cells, super capacitors, batteries, etc. In this direction, TiO2 is a truly versatile material for potential application in the field of photocatalysis, where it has been demonstrated for use in photocatalytic degradation of pollutants, hydrogen production, fuel conversion, etc. However, the properties of TiO2 for individual applications remain to be improved to obtain enhanced efficiency. Because energy is a crucial requirement for current and future generations, photocatalytic hydrogen production has received significant attention in the field of energy research. Accordingly, TiO2 has been found to be a potential material for photocatalytic hydrogen production applications. However, limitations such as (i) wide band gap energy, (ii) inadequate charge separation efficiency and (iii) limited charge transfer properties have largely hindered the real-time application of TiO2. In this context, the physical and chemical properties of TiO2 have been modified, which essentially simulates the energy structures in the material to improve its properties. Accordingly, this report reviewed physically and chemically modified TiO2 through processes such as defect creation, doping, compositing and metal loading onto TiO2 materials. These modifications essentially reveal that TiO2 should possess the following properties to achieve enhanced photocatalytic hydrogen production: (i) increased crystalline nature, (ii) defect structures within the threshold limit, (iii) more negative CB potential, (iv) a support for hole transportation, (v) narrow band gap energy to absorb visible light or equipping with plasmonic materials, and (vi) a dispersed CB for effective diffusion of electrons to the surface. Further, it is also revealed that these modifications in TiO2 substantially alter its energy structures and equip it with features and functions that increase its suitability for photocatalytic hydrogen production applications.
Future developments in TiO2 will involve customized designs to achieve higher quantum yields and enhanced solar-to-hydrogen conversion efficiencies. Especially, materials such as black-TiO2 are being intrinsically modified to absorb visible light. Similarly, TiO2 can also be intrinsically modified, such as red-TiO2, blue-TiO2 and yellow-TiO2, to specifically absorb certain visible wavelengths; these species have also demonstrated good photocatalytic activities. Therefore, these substantial developments and techniques for the customization of TiO2 show promise for their real-time application towards solar light-driven photocatalytic hydrogen production for sustainable energy and a clean environment, which will be important energy requirements in the near future.
Conflicts of interest
There are no conflicts to declare.
Acknowledgements
The authors gratefully acknowledge the financial support from Jain University and DST Nanomission (SR/NM/NS-20/2014).
References
- T. Kawai and T. Sakata, Conversion of carbohydrate into hydrogen fuel by a photocatalytic process, Nature, 1980, 286, 474–476 CrossRef CAS.
- H. P. Maruska and A. K. Ghosh, Photocatalytic decomposition of water at semiconductor electrodes, Sol. Energy, 1978, 20, 443–458 CrossRef CAS.
- A. Kudo, K. Ueda, H. Kato and I. Mikami, Photocatalytic O2 evolution under visible light irradiation on BiVO4 in aqueous AgNO3 solution, Catal. Lett., 1998, 53, 229–230 CrossRef CAS.
- H. Yoneyama, Electrochemical aspects of light-induced heterogeneous reactions on semiconductors, Crit. Rev. Solid State Mater. Sci., 1993, 18, 69–111 CrossRef CAS.
- K. Domen, J. N. Kondo, M. Hara and T. Takata, Photo- and Mechano-Catalytic Overall Water Splitting Reactions to Form Hydrogen and Oxygen on Heterogeneous Catalysts, Bull. Chem. Soc. Jpn., 2000, 73, 1307–1331 CrossRef CAS.
-
M. Graetzel, Energy Resources through Photochemistry and Catalysis, Academic Press, 1983 Search PubMed.
-
N. Serpone and E. PelizzettiPhotocatalysis, Wiley, New York, 1989 Search PubMed.
- A. Kudo, Hyomen, 1998, 36, 625 CAS.
- R. Shwetharani and R. Geetha Balakrishna, Comparative study of homogeneous and heterogeneous photo-oxidative treatment on bacterial cell via multianalytical techniques, J. Photochem. Photobiol., A, 2014, 295, 11–16 CrossRef CAS.
- H. Arakawa and K. Sayama, Solar hydrogen production. Significant effect of Na2CO3 addition on water splitting using simple oxide semiconductor photocatalysts, Catal. Surv. Jpn., 2000, 4, 75–80 CrossRef CAS.
- K. Domen, M. Hara, J. N. Kondo, T. Takata, A. Kudo, H. Kobayashi and Y. Inoue, New aspects of heterogeneous photocatalysts for water decomposition, Korean J. Chem. Eng., 2001, 18, 862–866 CrossRef CAS.
- Z. Zou and H. Arakawa, Direct water splitting into H2 and O2 under visible light irradiation with a new series of mixed oxide semiconductor photocatalysts, J. Photochem. Photobiol., A, 2003, 158, 145–162 CrossRef CAS.
- K. Maeda and K. Domen, New Non-Oxide Photocatalysts Designed for Overall Water Splitting under Visible Light, J. Phys. Chem. C, 2007, 111, 7851–7861 CrossRef CAS.
- T. Hisatomi, J. Kubota and K. Domen, Recent advances in semiconductors for photocatalytic and photoelectrochemical water splitting, Chem. Soc. Rev., 2014, 43, 7520–7535 RSC.
- L. P. D'Souza, R. Shwetharani, V. Amoli, C. A. N. Fernando, A. K. Sinha and R. G. Balakrishna, Photoexcitation of neodymium doped TiO2 for improved performance in dye-sensitized solar cells, Mater. Des., 2016, 104, 346–354 CrossRef.
- G. A. Somorjai, H. Frei and J. Y. Park, Advancing the frontiers in nanocatalysis, biointerfaces, and renewable energy conversion by innovations of surface techniques, J. Am. Chem. Soc., 2009, 131, 16589–16605 CrossRef CAS PubMed.
- S. Y. Moon, E. H. Gwag and J. Y. Park, Hydrogen Generation on Metal/Mesoporous Oxides: The Effects of Hierarchical Structure, Doping, and Co-catalysts, Energy Technol., 2018, 6, 459–469 CrossRef CAS.
- K. Maeda and K. Domen, Solid Solution of GaN and ZnO as a Stable Photocatalyst for Overall Water Splitting under Visible Light, Chem. Mater., 2010, 22, 612–623 CrossRef CAS.
- Y. Ma, X. Wang, Y. Jia, X. Chen, H. Han and C. Li, Titanium dioxide-based nanomaterials for photocatalytic fuel generations, Chem. Rev., 2014, 114, 9987–10043 CrossRef CAS PubMed.
- L. L. Jensen, J. T. Muckerman and M. D. Newton, First-Principles Studies of the Structural and Electronic Properties of the (Ga1-xZnx)(N1-xOx) Solid Solution Photocatalyst, J. Phys. Chem. C, 2008, 112, 3439–3446 CrossRef CAS.
- A. Kudo and Y. Miseki, Heterogeneous photocatalyst materials for water splitting, Chem. Soc. Rev., 2009, 38, 253–278 RSC.
- S. Ekambaram, Photoproduction of clean H2 or O2 from water using oxide semiconductors in presence of sacrificial reagent, J. Alloys Compd., 2008, 448, 238–245 CrossRef CAS.
- M. Zalas and M. Laniecki, Photocatalytic hydrogen generation over lanthanides-doped titania, Sol. Energy Mater. Sol. Cells, 2005, 89, 287–296 CrossRef CAS.
- F. E. Osterloh, Inorganic Materials as Catalysts for Photochemical Splitting of Water, Chem. Mater., 2008, 20, 35–54 CrossRef CAS.
- L. P. D'Souza, S. Shree and G. R. Balakrishna, Bifunctional Titania Float for Metal Ion Reduction and Organics Degradation, via Sunlight, Ind. Eng. Chem. Res., 2013, 52, 16162–16168 CrossRef.
- K. U. Minchitha and R. Geetha Balakrishna, Structural modification and property tailoring in titania for high efficiency in sunlight, Mater. Chem. Phys., 2012, 136, 720–728 CrossRef CAS.
- R. Shwetharani and R. G. Balakrishna, Efficient algal lipid extraction via photocatalysis and its conversion to biofuel, Appl. Energy, 2016, 168, 364–374 CrossRef CAS.
- R. Shwetharani and R. G. Balakrishna, Photo-active float for field water disinfection, Photochem. Photobiol. Sci., 2016, 15, 447–455 RSC.
- A. Kudo and Y. Miseki, Heterogeneous photocatalyst materials for water splitting, Chem. Soc. Rev., 2009, 38, 253–278 RSC.
- E. Moretti, E. Rodríguez-Aguado, A. I. Molina, E. Rodríguez-Castellón, A. Talon and L. Storaro, Sustainable photo-assisted CO oxidation in H2-rich stream by simulated solar light response of Au nanoparticles supported on TiO2, Catal. Today, 2017, 304, 135–142 CrossRef.
- Y.-P. Yuan, L.-W. Ruan, J. Barber, S. C. Joachim Loo and C. Xue, Hetero-nanostructured suspended photocatalysts for solar-to-fuel conversion, Energy Environ. Sci., 2014, 7, 3934–3951 RSC.
- T. Luttrell, S. Halpegamage, J. Tao, A. Kramer, E. Sutter and M. Batzill, Why is anatase a better photocatalyst than rutile?--Model studies on epitaxial TiO2 films, Sci. Rep., 2014, 4, 4043 CrossRef PubMed.
- A. Li, Z. Wang, H. Yin, S. Wang, P. Yan, B. Huang, X. Wang, R. Li, X. Zong, H. Han and C. Li, Understanding the anatase–rutile phase junction in charge separation and transfer in a TiO2electrode for photoelectrochemical water splitting, Chem. Sci., 2016, 7, 6076–6082 RSC.
- E. Pulido Melián, O. González Díaz, A. Ortega Méndez, C. R. López, M. Nereida Suárez, J. M. Doña Rodríguez, J. A. Navío, D. Fernández Hevia and J. Pérez Peña, Efficient and affordable hydrogen production by water photo-splitting using TiO2-based photocatalysts, Int. J. Hydrogen Energy, 2013, 38, 2144–2155 CrossRef.
- J. Hu, Y. Cao, K. Wang and D. Jia, Green solid-state synthesis and photocatalytic hydrogen production activity of anatase TiO2 nanoplates with super heat-stability, RSC Adv., 2017, 7, 11827–11833 RSC.
- H. Razavi-Khosroshahi, K. Edalati, M. Hirayama, H. Emami, M. Arita, M. Yamauchi, H. Hagiwara, S. Ida, T. Ishihara, E. Akiba, Z. Horita and M. Fuji, Visible-Light-Driven Photocatalytic Hydrogen Generation on Nanosized TiO2-II Stabilized by High-Pressure Torsion, ACS Catal., 2016, 6, 5103–5107 CrossRef CAS.
- G. Cheng, Y. Wei, J. Xiong, Y. Gan, J. Zhu and F. Xu, Same titanium glycolate precursor but different products: successful synthesis of twinned anatase TiO2 nanocrystals with excellent solar photocatalytic hydrogen evolution capability, Inorg. Chem. Front., 2017, 4, 1319–1329 RSC.
- Y. X. Chen, V. Gombac, T. Montini, A. Lavacchi, J. Filippi, H. A. Miller, P. Fornasiero and F. Vizza, An increase in hydrogen production from light and ethanol using a dual scale porosity photocatalyst, Green Chem., 2018, 20, 2299–2307 RSC.
- H.-y. Lin and C.-y. Shih, Efficient One-Pot Microwave-Assisted Hydrothermal Synthesis of Nitrogen-Doped TiO2 for Hydrogen Production by Photocatalytic Water Splitting, Catal. Surv. Asia, 2012, 16, 231–239 CrossRef CAS.
- L. Pan, J. Zhang, X. Jia, Y.-H. Ma, X. Zhang, L. Wang and J.-J. Zou, Highly efficient Z-scheme WO3–x quantum dots/TiO2 for photocatalytic hydrogen generation, Chin. J. Catal., 2017, 38, 253–259 CrossRef CAS.
- K. Maeda, Z-Scheme Water Splitting Using Two Different Semiconductor Photocatalysts, ACS Catal., 2013, 3, 1486–1503 CrossRef CAS.
- F. Xu, W. Xiao, B. Cheng and J. Yu, Direct Z-scheme anatase/rutile bi-phase nanocomposite TiO2 nanofiber photocatalyst with enhanced photocatalytic H2-production activity, Int. J. Hydrogen Energy, 2014, 39, 15394–15402 CrossRef CAS.
- K. Maeda, N. Murakami and T. Ohno, Dependence of Activity of Rutile Titanium(IV) Oxide Powder for Photocatalytic Overall Water Splitting on Structural Properties, J. Phys. Chem. C, 2014, 118, 9093–9100 CrossRef CAS.
- J. Wang, P. Zhang, X. Li, J. Zhu and H. Li, Synchronical pollutant degradation and H2 production on a Ti3+−doped TiO2 visible photocatalyst with dominant (001) facets, Appl. Catal., A, 2013, 134–135, 198–204 CrossRef CAS.
- K. M. Parida, S. Pany and B. Naik, Green synthesis of fibrous hierarchical meso-macroporous N doped TiO2 nanophotocatalyst with enhanced photocatalytic H2 production, Int. J. Hydrogen Energy, 2013, 38, 3545–3553 CrossRef CAS.
- P. S. Niphadkar, S. K. Chitale, S. K. Sonar, S. S. Deshpande, P. N. Joshi and S. V. Awate, Synthesis, characterization and photocatalytic behavior of TiO2–SiO2 mesoporous composites in hydrogen generation from water splitting, J. Mater. Sci., 2014, 49, 6383–6391 CrossRef CAS.
- T. Wang, X. Yan, S. Zhao, B. Lin, C. Xue, G. Yang, S. Ding, B. Yang, C. Ma, G. Yang and G. Yang, A facile one-step synthesis of three-dimensionally ordered macroporous N-doped TiO2 with ethanediamine as the nitrogen source, J. Mater. Chem. A, 2014, 2, 15611–15619 RSC.
- C. Han, Y. Wang, Y. Lei, B. Wang, N. Wu, Q. Shi and Q. Li, In situ synthesis of graphitic-C3N4 nanosheet hybridized N-doped TiO2 nanofibers for efficient photocatalytic H2 production and degradation, Nano Res., 2015, 8, 1199–1209 CrossRef CAS.
- T. Sreethawong, S. Ngamsinlapasathian and S. Yoshikawa, Influences of urea–glycerol mixtures as mixed mesopore-controlling agents on tailoring physicochemical properties and photocatalytic H2 production activity of sol–gel-derived mesoporous-assembled TiO2 nanocrystals, Mater. Res. Bull., 2013, 48, 30–36 CrossRef CAS.
- Z. Zhang, F. Zuo and P. Feng, Hard template synthesis of crystalline mesoporous anatase TiO2 for photocatalytic hydrogen evolution, J. Mater. Chem., 2010, 20, 2206–2212 RSC.
- F. Teng, M. Chen, N. Li, X. Hua, K. Wang and T. Xu, Effect of TiO2 Surface Structure on the Hydrogen Production Activity of the Pt@CuO/TiO2 Photocatalysts for Water Splitting, ChemCatChem, 2014, 6, 842–847 CrossRef CAS.
- S. Zhu, F. Yao, C. Yin, Y. Li, W. Peng, J. Ma and D. Zhang, Fe2O3/TiO2 photocatalyst of hierarchical structure for H2 production from water under visible light irradiation, Microporous Mesoporous Mater., 2014, 190, 10–16 CrossRef CAS.
- L. Ding, H. Zhou, S. Lou, J. Ding, D. Zhang, H. Zhu and T. Fan, Butterfly wing architecture assisted CdS/Au/TiO2 Z-scheme type photocatalytic water splitting, Int. J. Hydrogen Energy, 2013, 38, 8244–8253 CrossRef CAS.
- K. Kiatkittipong, A. Iwase, J. Scott and R. Amal, Photocatalysis of heat treated sodium- and hydrogen-titanate nanoribbons for water splitting, H2/O2 generation and oxalic acid oxidation, Chem. Eng. Sci., 2013, 93, 341–349 CrossRef CAS.
- S.-y. Guo, S. Han, B. Chi, J. Pu and J. Li, Synthesis of shape-controlled mesoporous titanium phosphate nanocrystals: The hexagonal titanium phosphate with enhanced hydrogen generation from water splitting, Int. J. Hydrogen Energy, 2014, 39, 2446–2453 CrossRef CAS.
- Z. Haider and Y. S. Kang, Facile Preparation of Hierarchical TiO2 Nano Structures: Growth Mechanism and Enhanced Photocatalytic H2 Production from Water Splitting Using Methanol as a Sacrificial Reagent, ACS Appl. Mater. Interfaces, 2014, 6, 10342–10352 CrossRef CAS PubMed.
- R. Ghosh Chaudhuri and S. Paria, Core/Shell Nanoparticles: Classes, Properties, Synthesis Mechanisms, Characterization, and Applications, Chem. Rev., 2012, 112, 2373–2433 CrossRef CAS PubMed.
- M. B. Gawande, A. Goswami, T. Asefa, H. Guo, A. V. Biradar, D.-L. Peng, R. Zboril and R. S. Varma, Core-shell nanoparticles: synthesis and applications in catalysis and electrocatalysis, Chem. Soc. Rev., 2015, 44, 7540–7590 RSC.
- Y. Im, S. Kang, K. M. Kim, T. Ju, G. B. Han, N.-K. Park, T. J. Lee and M. Kang, Dynamic Hydrogen Production from Methanol/Water Photo-Splitting Using Core@Shell-Structured CuS@TiO2Catalyst Wrapped by High Concentrated TiO2Particles, Int. J. Photoenergy, 2013, 2013, 1–10 CrossRef.
- J. Fang, L. Xu, Z. Zhang, Y. Yuan, S. Cao, Z. Wang, L. Yin, Y. Liao and C. Xue, Au@TiO2–CdS Ternary Nanostructures for Efficient Visible-Light-Driven Hydrogen Generation, ACS Appl. Mater. Interfaces, 2013, 5, 8088–8092 CrossRef CAS PubMed.
- J. E. Carrera-Crespo, G. Ramos-Sánchez, V. De la Luz, F. González, E. Barrera and I. González, Photoelectrochemical hydrogen generation on TiO2 nanotube arrays sensitized with CdS@Sb2S3 core shell particles, Int. J. Hydrogen Energy, 2017, 42, 30249–30256 CrossRef CAS.
- C. Yang, Z. Wang, T. Lin, H. Yin, X. Lü, D. Wan, T. Xu, C. Zheng, J. Lin, F. Huang, X. Xie and M. Jiang, Core-Shell Nanostructured “Black” Rutile Titania as Excellent Catalyst for Hydrogen Production Enhanced by Sulfur Doping, J. Am. Chem. Soc., 2013, 135, 17831–17838 CrossRef CAS PubMed.
- A. Madhumitha, V. Preethi and S. Kanmani, Photocatalytic hydrogen production using TiO2 coated iron-oxide core shell particles, Int. J. Hydrogen Energy, 2018, 43, 3946–3956 CrossRef CAS.
- X. An, D. Cheng, L. Dai, B. Wang, H. J. Ocampo, J. Nasrallah, X. Jia, J. Zou, Y. Long and Y. Ni, Synthesis of nano-fibrillated cellulose/magnetite/titanium dioxide (NFC@Fe3O4@TNP) nanocomposites and their application in the photocatalytic hydrogen generation, Appl. Catal., A, 2017, 206, 53–64 CrossRef CAS.
- G. Liu, J. Pan, L. Yin, J. T. S. Irvine, F. Li, J. Tan, P. Wormald and H.-M. Cheng, Heteroatom-Modulated Switching of Photocatalytic Hydrogen and Oxygen Evolution Preferences of Anatase TiO2 Microspheres, Adv. Funct. Mater., 2012, 22, 3233–3238 CrossRef CAS.
- U. Diebold, The Surface Science of Titanium Dioxide, Surf. Sci. Rep., 2003, 48, 53–229 CrossRef CAS.
- C. D. V. Emanuele Finazzi and P. Gianfranco, Boron-Doped Anatase TiO2: Pure and Hybrid DFT Calculations, J. Phys. Chem. C, 2009, 113, 220–228 CrossRef.
- N. Feng, A. Zheng, Q. Wang, P. Ren, X. Gao, S.-B. Liu, Z. Shen, T. Chen and F. Deng, Boron Environments in B-Doped and (B, N)-Codoped TiO2 Photocatalysts: A Combined Solid-State NMR and Theoretical Calculation Study, J. Phys. Chem. C, 2011, 115, 2709–2719 CrossRef CAS.
- H. S. Kim, D. Kim, B. S. Kwak, G. B. Han, M.-H. Um and M. Kang, Synthesis of magnetically separable core@shell structured NiFe2O4@TiO2 nanomaterial
and its use for photocatalytic hydrogen production by methanol/water splitting, Chem. Eng. J., 2014, 243, 272–279 CrossRef CAS.
- C.-C. Lin, T.-Y. Wei, K.-T. Lee and S.-Y. Lu, Titania and Pt/titania aerogels as superior mesoporous structures for photocatalytic water splitting, J. Mater. Chem., 2011, 21, 12668–12674 RSC.
- S. K. Parayil, R. J. Psota and R. T. Koodali, Modulating the textural properties and photocatalytic hydrogen production activity of TiO2 by high temperature supercritical drying, Int. J. Hydrogen Energy, 2013, 38, 10215–10225 CrossRef CAS.
- J. Huang, Y. Jiang, G. Li, C. Xue and W. Guo, Hetero-structural NiTiO3/TiO2 nanotubes for efficient photocatalytic hydrogen generation, Renewable Energy, 2017, 111, 410–415 CrossRef CAS.
- S. Swetha, M. K. Singh, K. U. Minchitha and R. G. Balakrishna, Elucidation of cell killing mechanism by comparative analysis of photoreactions on different types of bacteria, Photochem. Photobiol., 2012, 88, 414–422 CrossRef CAS PubMed.
- H. X. Zhu, P. X. Zhou, X. Li and J. M. Liu, Electronic structures and optical properties of rutile TiO2 with different point defects from DFT + U calculations, Phys. Lett. A, 2014, 378, 2719–2724 CrossRef CAS.
- S. Swetha, S. M. Santhosh and R. Geetha Balakrishna, Enhanced bactericidal activity of modified titania in sunlight against Pseudomonas aeruginosa, a water-borne pathogen, Photochem. Photobiol., 2010, 86, 1127–1134 CrossRef CAS PubMed.
- L. P. D'Souza, S. Muralikrishna, H. R. Chandan, T. Ramakrishnappa and R. G. Balakrishna, Neodymium doped titania as photoanode and graphene oxide–CuS composite as counter electrode material in quantum dot solar cell, J. Mater. Res., 2015, 30, 3241–3251 CrossRef.
- B. Roose, S. Pathak and U. Steiner, Doping of TiO2 for sensitized solar cells, Chem. Soc. Rev., 2015, 44, 8326–8349 RSC.
- M. S. Jyothi, P. D'Souza Laveena, R. Shwetharani and G. R. Balakrishna, Novel hydrothermal method for effective doping of N and F into nano Titania for both, energy and environmental applications, Mater. Res. Bull., 2016, 74, 478–484 CrossRef CAS.
- S. Swetha and R. G. Balakrishna, Preparation and Characterization of High Activity Zirconium-Doped Anatase Titania for Solar Photocatalytic Degradation of Ethidium Bromide, Chin. J. Catal., 2011, 32, 789–794 CrossRef CAS.
- S. Santhosh, S. Swetha and G. Balakrishna, Structure and photocatalytic activity of Ti1−X MX O2 (M = Zr, Co and Mo) synthesized by pulverized solid state technique, Open Chem., 2010, 8, 453–460 CAS.
- W. Y. Teoh, J. A. Scott and R. Amal, Progress in Heterogeneous Photocatalysis: From Classical Radical Chemistry to Engineering Nanomaterials and Solar Reactors, J. Phys. Chem. Lett., 2012, 3, 629–639 CrossRef CAS PubMed.
- R. Shwetharani, M. S. Jyothi, P. D. Laveena and R. Geetha Balakrishna, Photoactive titania float for disinfection of water; evaluation of cell damage by bioanalytical techniques, Photochem. Photobiol., 2014, 90, 1099–1107 CAS.
-
W. Shockley, Electrons and Holes in Semiconductors, 1950 Search PubMed.
- A. Ibhadon and P. Fitzpatrick, Heterogeneous Photocatalysis: Recent Advances and Applications, Catalysts, 2013, 3, 189–218 CrossRef CAS.
- A. L. Linsebigler, G. Lu and J. T. Yates, Photocatalysis on TiO2 Surfaces: Principles, Mechanisms, and Selected Results, Chem. Rev., 1995, 95, 735–758 CrossRef CAS.
- R. F. Howe and M. Gratzel, EPR study of hydrated anatase under UV irradiation, J. Phys. Chem., 1987, 91, 3906–3909 CrossRef CAS.
- G. Rothenberger, J. Moser, M. Graetzel, N. Serpone and D. K. Sharma, Charge Carrier Trapping and Recombination Dynamics in Small Semiconductor Particles, J. Am. Chem. Soc., 1985, 107, 8054–8059 CrossRef CAS.
- C.-H. Liao, C.-W. Huang and J. C. S. Wu, Hydrogen Production from Semiconductor-based Photocatalysis via Water Splitting, Catalysts, 2012, 2, 490–516 CrossRef CAS.
- Z. Zou, J. Ye, K. Sayama and H. Arakawa, Direct splitting of water under visible light irradiation with an oxide semiconductor photocatalyst, Nature, 2001, 414, 625–627 CrossRef CAS PubMed.
- W. Choi, A. Termin and M. R. Hoffmann, The Role of Metal Ion Dopants in Quantum-Sized TiO2: Correlation between Photoreactivity and Charge Carrier Recombination Dynamics, J. Phys. Chem., 1994, 98, 13669–13679 CrossRef.
- X. Chen, L. Liu, P. Y. Yu and S. S. Mao, Increasing solar absorption for photocatalysis with black hydrogenated titanium dioxide nanocrystals, Science, 2011, 331, 746–750 CrossRef CAS PubMed.
- Y.-D. Zhang, X.-M. Huang, Y.-Y. Yang, N. Zhou, Y.-H. Luo, D.-M. Li and Q.-B. Meng, How to improve the performance of dye-sensitized solar modules by “back leads”, Sol. Energy Mater. Sol. Cells, 2012, 102, 109–113 CrossRef CAS.
- W. Zhou, Y.-h. Zheng and G.-h. Wu, Novel luminescent RE/TiO2 (RE = Eu, Gd) catalysts prepared by in-situation sol–gel approach construction of multi-functional precursors and their photo or photocatalytic oxidation properties, Appl. Surf. Sci., 2006, 253, 1387–1392 CrossRef CAS.
- Z. Zongyan and L. Qingju, Effects of lanthanide doping on electronic structures and optical properties of anatase TiO 2 from density functional theory calculations, J. Phys. D: Appl. Phys., 2008, 41, 085417 CrossRef.
- Z. Tong, T. Peng, W. Sun, W. Liu, S. Guo and X.-Z. Zhao, Introducing an Intermediate Band into Dye-Sensitized Solar Cells by W6+ Doping into TiO2 Nanocrystalline Photoanodes, J. Phys. Chem. C, 2014, 118, 16892–16895 CrossRef CAS.
- J. Zhang, Z. Zhao, X. Wang, T. Yu, J. Guan, Z. Yu, Z. Li and Z. Zou, Increasing the Oxygen Vacancy Density on the TiO2 Surface by La-Doping for Dye-Sensitized Solar Cells, J. Phys. Chem. C, 2010, 114, 18396–18400 CrossRef CAS.
- A. T. Kuvarega and B. B. Mamba, TiO2-based Photocatalysis: Toward Visible Light-Responsive Photocatalysts Through Doping and Fabrication of Carbon-based Nanocomposites, Crit. Rev. Solid State Mater. Sci., 2016, 1–52 Search PubMed.
- C. M. Malengreaux, S. L. Pirard, G. Léonard, J. G. Mahy, M. Herlitschke, B. Klobes, R. Hermann, B. Heinrichs and J. R. Bartlett, Study of the photocatalytic activity of Fe3+, Cr3+, La3+ and Eu3+ single-doped and co-doped TiO2 catalysts produced by aqueous sol-gel processing, J. Alloys Compd., 2017, 691, 726–738 CrossRef CAS.
- S. Peng, Y. Huang and Y. Li, Rare earth doped TiO2-CdS and TiO2-CdS composites with improvement of photocatalytic hydrogen evolution under visible light irradiation, Mater. Sci. Semicond. Process., 2013, 16, 62–69 CrossRef CAS.
- C. H. Ravikumar, M. Sakar, A. Mahto, R. T. Nanjundaiah, R. Thippeswamy, S. R. Teixeira and R. G. Balakrishna, Observation of oxo-bridged yttrium in TiO 2 nanostructures and their enhanced photocatalytic hydrogen generation under UV/Visible light irradiations, Mater. Res. Bull., 2018, 104, 212–219 CrossRef CAS.
- R. Shwetharani, M. Sakar, H. R. Chandan and R. Geetha Balakrishna, Observation of simultaneous photocatalytic degradation and hydrogen evolution on the lanthanum modified TiO 2 nanostructures, Mater. Lett., 2018, 218, 262–265 CrossRef CAS.
- N. Tai Ly, T. Hoa Dao, L. H. Hoang To, D. Lam Vu and V. Hong Le, Hydrogen generation by photoelectrochemical effect of the Cu-doped TiO2 photoanode, Adv. Nat. Sci.: Nanosci. Nanotechnol., 2014, 5, 035009 Search PubMed.
- M.-Y. Xie, K.-Y. Su, X.-Y. Peng, R.-J. Wu, M. Chavali and W.-C. Chang, Hydrogen production by photocatalytic water-splitting on Pt-doped TiO2–ZnO under visible light, J. Taiwan Inst. Chem. Eng., 2017, 70, 161–167 CrossRef CAS.
- K. Villa, A. Black, X. Domènech and J. Peral, Nitrogen doped TiO2 for hydrogen production under visible light irradiation, Sol. Energy, 2012, 86, 558–566 CrossRef CAS.
- R. Dholam, N. Patel, M. Adami and A. Miotello, Hydrogen production by
photocatalytic water-splitting using Cr- or Fe-doped TiO2 composite thin films photocatalyst, Int. J. Hydrogen Energy, 2009, 34, 5337–5346 CrossRef CAS.
- G. Fu, P. Zhou, M. Zhao, W. Zhu, S. Yan, T. Yu and Z. Zou, Carbon coating stabilized Ti3+−doped TiO2 for photocatalytic hydrogen generation under visible light irradiation, Dalton Trans., 2015, 44, 12812–12817 RSC.
- F. Wang, J. H. Ho, Y. Jiang and R. Amal, Tuning Phase Composition of TiO2 by Sn4+ Doping for Efficient Photocatalytic Hydrogen Generation, ACS Appl. Mater. Interfaces, 2015, 7, 23941–23948 CrossRef CAS PubMed.
- N. A. Race, W. Zhang, M. E. Screen, B. A. Barden and W. R. McNamara, Iron polypyridyl catalysts assembled on metal oxide semiconductors for photocatalytic hydrogen generation, Chem. Commun., 2018, 54, 3290–3293 RSC.
- X. Fan, J. Fan, X. Hu, E. Liu, L. Kang, C. Tang, Y. Ma, H. Wu and Y. Li, Preparation and characterization of Ag deposited and Fe doped TiO2 nanotube arrays for photocatalytic hydrogen production by water splitting, Ceram. Int., 2014, 40, 15907–15917 CrossRef CAS.
- L. Zhang, W. Wang, S. Zeng, Y. Su and H. Hao, Enhanced H2 evolution from photocatalytic cellulose conversion based on graphitic carbon layers on TiO2/NiOx, Green Chem., 2018, 20, 3008–3013 RSC.
- T. Sun, J. Fan, E. Liu, L. Liu, Y. Wang, H. Dai, Y. Yang, W. Hou, X. Hu and Z. Jiang, Fe and Ni co-doped TiO2 nanoparticles prepared by alcohol-thermal method: Application in hydrogen evolution by water splitting under visible light irradiation, Powder Technol., 2012, 228, 210–218 CrossRef CAS.
- T.-R. Kuo, H.-J. Liao, Y.-T. Chen, C.-Y. Wei, C.-C. Chang, Y.-C. Chen, Y.-H. Chang, J.-C. Lin, Y.-C. Lee, C.-Y. Wen, S.-S. Li, K.-H. Lin and D.-Y. Wang, Extended visible to near-infrared harvesting of earth-abundant FeS2–TiO2 heterostructures for highly active photocatalytic hydrogen evolution, Green Chem., 2018, 20, 1640–1647 RSC.
- N. Lakshmana Reddy, K. K. Cheralathan, V. Durga Kumari, B. Neppolian and S. Muthukonda Venkatakrishnan, Photocatalytic Reforming of Biomass Derived Crude Glycerol in Water: A Sustainable Approach for Improved Hydrogen Generation Using Ni(OH)2 Decorated TiO2 Nanotubes under Solar Light Irradiation, ACS Sustainable Chem. Eng., 2018, 6, 3754–3764 CrossRef CAS.
- M. T. Uddin, Y. Nicolas, C. Olivier, W. Jaegermann, N. Rockstroh, H. Junge and T. Toupance, Band alignment investigations of heterostructure NiO/TiO2 nanomaterials used as efficient heterojunction earth-abundant metal oxide photocatalysts for hydrogen production, Phys. Chem. Chem. Phys., 2017, 19, 19279–19288 RSC.
- B. Allured, DelaCruz, Steven Darling, Timothy Huda, Muhammad, N. Subramanian, Vaidyanathan. Enhancing the visible light absorbance of Bi2Ti2O7 through Fe-substitution and its effects on photocatalytic hydrogen evolution, Appl. Catal., A, 2014, 144, 261–268 CrossRef CAS.
- T. Kako, Z. Zou and J. Ye, Photocatalytic oxidation of 2-propanol in the gas phase over cesium bismuth niobates under visible light irradiation, Res. Chem. Intermed., 2005, 31, 359–364 CrossRef CAS.
- L. L. Garza-Tovar, L. M. Torres-Martínez, D. Bernal Rodríguez, R. Gómez and G. del Angel, Photocatalytic degradation of methylene blue on Bi2MNbO7 (M=Al, Fe, In, Sm) sol–gel catalysts, J. Mol. Catal. A: Chem., 2006, 247, 283–290 CrossRef CAS.
- S. Murugesan, M. N. Huda, Y. Yan, M. M. Al-Jassim and V. R. Subramanian, Band-Engineered Bismuth Titanate Pyrochlores for Visible Light Photocatalysis, J. Phys. Chem. C, 2010, 114, 10598–10605 CrossRef CAS.
- M. N. Huda, A. Walsh, Y. Yan, S.-H. Wei and M. M. Al-Jassim, Electronic, structural, and magnetic effects of 3d transition metals in hematite, J. Appl. Phys., 2010, 107, 123712 CrossRef.
- C. Li, Z. Jiang and Z. Yao, Fabrication and characterization of multi-metal co-doped titania films for a water-splitting reaction, Dalton Trans., 2010, 39, 10692–10696 RSC.
- J. Huang, G. Li, Z. Zhou, Y. Jiang, Q. Hu, C. Xue and W. Guo, Efficient photocatalytic hydrogen production over Rh and Nb codoped TiO2 nanorods, Chem. Eng. J., 2018, 337, 282–289 CrossRef CAS.
- S. Chu, A. E. Becerikli, B. Kortewille, F. E. Oropeza and J. Strunk, Tin-grafted TiO2 with enhanced activity for photocatalytic hydrogen generation from aqueous methanol solutions, Int. J. Hydrogen Energy, 2014, 39, 18784–18792 CrossRef CAS.
-
M. Matsuoka and M. Anpo, Local Structures, Excited States, and Photocatalytic Reactivities of “Single-Site” Ti-Oxide Photocatalysts Constructed Within Zeolites or Mesoporous Materials. In Environmentally Benign Photocatalysts: Applications of Titanium Oxide-based Materials, ed. M. Anpo and P. V. Kamat, Springer New York, New York, NY, 2010, pp. 217–232 Search PubMed.
- Q. Zhou, L. Li, Y. Xin, D. Liu and X. Zhang, Three-dimensionally ordered macroporous Sn4+−doped TiO2 with anatase–rutile mixed phase via Pt loading by photoreduction method: enhanced photodegradation and hydrogen production performance, New J. Chem., 2018, 42, 15190–15199 RSC.
- L. Hou, Z. Guan, M. Zhang, C. He, Q. Li and J. Yang, Adjusting the ratio of bulk single-electron-trapped oxygen vacancies/surface oxygen vacancies in TiO2 for efficient photocatalytic hydrogen evolution, Catal. Sci. Technol., 2018, 8, 2809–2817 RSC.
- L.-B. Xiong, J.-L. Li, B. Yang and Y. Yu, Ti3+in the Surface of Titanium Dioxide: Generation, Properties and Photocatalytic Application, J. Nanomater., 2012, 2012, 1–13 CrossRef.
- F. N. Sayed, O. D. Jayakumar, R. Sasikala, R. M. Kadam, S. R. Bharadwaj, L. Kienle, U. Schürmann, S. Kaps, R. Adelung, J. P. Mittal and A. K. Tyagi, Photochemical Hydrogen Generation Using Nitrogen-Doped TiO2–Pd Nanoparticles: Facile Synthesis and Effect of Ti3+ Incorporation, J. Phys. Chem. C, 2012, 116, 12462–12467 CrossRef CAS.
- F. N. Sayed, R. Sasikala, O. D. Jayakumar, R. Rao, C. A. Betty, A. Chokkalingam, R. M. Kadam, Jagannath, S. R. Bharadwaj, A. Vinu and A. K. Tyagi, Photocatalytic hydrogen generation from water using a hybrid of graphene nanoplatelets and self doped TiO2-Pd, RSC Adv., 2014, 4, 13469–13476 RSC.
- I. Justicia, P. Ordejón, G. Canto, J. L. Mozos, J. Fraxedas, G. A. Battiston, R. Gerbasi and A. Figueras, Designed Self-Doped Titanium Oxide Thin Films for Efficient Visible-Light Photocatalysis, Adv. Mater., 2002, 14, 1399–1402 CrossRef CAS.
- N. L. De Silva, A. C. A. Jayasundera, A. Folger, O. Kasian, S. Zhang, C.-F. Yan, C. Scheu and J. Bandara, Superior solar-to-hydrogen energy conversion efficiency by visible light-driven hydrogen production via highly reduced Ti2+/Ti3+ states in a blue titanium dioxide photocatalyst, Catal. Sci. Technol., 2018, 8, 4657–4664 RSC.
- X. Kong, Y. Xu, Z. Cui, Z. Li, Y. Liang, Z. Gao, S. Zhu and X. Yang, Defect enhances photocatalytic activity of ultrathin TiO 2 (B) nanosheets for hydrogen production by plasma engraving method, Appl. Catal., A, 2018, 230, 11–17 CrossRef CAS.
- Y. Jiang, H. Ning, C. Tian, B. Jiang, Q. Li, H. Yan, X. Zhang, J. Wang, L. Jing and H. Fu, Single-crystal TiO 2 nanorods assembly for efficient and stable cocatalyst-free photocatalytic hydrogen evolution, Appl. Catal., A, 2018, 229, 1–7 CrossRef CAS.
- G. Wang, H. Wang, Y. Ling, Y. Tang, X. Yang, R. C. Fitzmorris, C. Wang, J. Z. Zhang and Y. Li, Hydrogen-treated TiO2 nanowire arrays for photoelectrochemical water splitting, Nano Lett., 2011, 11, 3026–3033 CrossRef CAS PubMed.
- X. Jiang, Y. Zhang, J. Jiang, Y. Rong, Y. Wang, Y. Wu and C. Pan, Characterization of Oxygen Vacancy Associates within Hydrogenated TiO2: A Positron Annihilation Study, J. Phys. Chem. C, 2012, 116, 22619–22624 CrossRef CAS.
- Z. Zheng, B. Huang, J. Lu, Z. Wang, X. Qin, X. Zhang, Y. Dai and M.-H. Whangbo, Hydrogenated titania: synergy of surface modification and morphology improvement for enhanced photocatalytic activity, Chem. Commun., 2012, 48, 5733–5735 RSC.
- W. Wei, N. Yaru, L. Chunhua and X. Zhongzi, Hydrogenation of TiO2 nanosheets with exposed {001} facets for enhanced photocatalytc activity, RSC Adv., 2012, 2, 8286–8288 RSC.
- J. Lu, Y. Dai, H. Jin and B. Huang, Effective increasing of optical absorption and energy conversion efficiency of anatase TiO2 nanocrystals by hydrogenation, Phys. Chem. Chem. Phys., 2011, 13, 18063–18068 RSC.
- Z. Wang, C. Yang, T. Lin, H. Yin, P. Chen, D. Wan, F. Xu, F. Huang, J. Lin, X. Xie and M. Jiang, H-Doped Black Titania with Very High Solar Absorption and Excellent Photocatalysis Enhanced by Localized Surface Plasmon Resonance, Adv. Funct. Mater., 2013, 23, 5444–5450 CrossRef CAS.
- O. Elbanna, M. Fujitsuka, S. Kim and T. Majima, Charge Carrier Dynamics in TiO2 Mesocrystals with Oxygen Vacancies for Photocatalytic Hydrogen Generation under Solar Light Irradiation, J. Phys. Chem. C, 2018, 122, 15163–15170 CrossRef CAS.
- U. Pal, S. Ghosh and D. Chatterjee, Effect of sacrificial electron donors on hydrogen generation over visible light–irradiated nonmetal-doped TiO2 photocatalysts, Transition Met. Chem., 2012, 37, 93–96 CrossRef CAS.
- J. Zhang, W. Dang, Z. Ao, S. K. Cushing and N. Wu, Band gap narrowing in nitrogen-doped La2Ti2O7 predicted by density-functional theory calculations, Phys. Chem. Chem. Phys., 2015, 17, 8994–9000 RSC.
- G. Mountjoy, D. M. Pickup, G. W. Wallidge, R. Anderson, J. M. Cole, R. J. Newport and M. E. Smith, XANES Study of Ti Coordination in Heat-Treated (TiO2)x(SiO2)1-x Xerogels, Chem. Mater., 1999, 11, 1253–1258 CrossRef CAS.
- C. Wang, Q. Hu, J. Huang, L. Wu, Z. Deng, Z. Liu, Y. Liu and Y. Cao, Efficient hydrogen production by photocatalytic water splitting using N-doped TiO2 film, Appl. Surf. Sci., 2013, 283, 188–192 CrossRef CAS.
- J. Tang, A. J. Cowan, J. R. Durrant and D. R. Klug, Mechanism of O2 Production from Water Splitting: Nature of Charge Carriers in Nitrogen Doped Nanocrystalline TiO2 Films and Factors Limiting O2 Production, J. Phys. Chem. C, 2011, 115, 3143–3150 CrossRef CAS.
- H. Lu, J. Zhao, L. Li, L. Gong, J. Zheng, L. Zhang, Z. Wang, J. Zhang and Z. Zhu, Selective oxidation of sacrificial ethanol over TiO2-based photocatalysts during water splitting, Energy Environ. Sci., 2011, 4, 3384–3388 RSC.
- P. Zheng, H. Wu, J. Guo, J. Dong, S. Jia and Z. Zhu, P–N co-doping induced structural recovery of TiO2 for overall water splitting under visible light irradiation, J. Alloys Compd., 2014, 615, 79–83 CrossRef CAS.
- X. Sun, H. Liu, J. Dong, J. Wei and Y. Zhang, Preparation and Characterization of Ce/N-Codoped TiO2 Particles for Production of H2
by Photocatalytic Splitting Water Under Visible Light, Catal. Lett., 2010, 135, 219–225 CrossRef CAS.
- R. Asahi, T. Morikawa, T. Ohwaki, K. Aoki and Y. Taga, Visible-light photocatalysis in nitrogen-doped titanium oxides, Science, 2001, 293, 269–271 CrossRef CAS PubMed.
- Q. Xiang, J. Yu and M. Jaroniec, Nitrogen and sulfur co-doped TiO2nanosheets with exposed {001} facets: synthesis, characterization and visible-light photocatalytic activity, Phys. Chem. Chem. Phys., 2011, 13, 4853–4861 RSC.
- J. A. Rengifo-Herrera, E. Mielczarski, J. Mielczarski, N. C. Castillo, J. Kiwi and C. Pulgarin, Escherichia coli inactivation by N, S co-doped commercial TiO2 powders under UV and visible light, Appl. Catal., A, 2008, 84, 448–456 CrossRef CAS.
- K. Nishijima, T. Kamai, N. Murakami, T. Tsubota and T. Ohno, Photocatalytic Hydrogen or Oxygen Evolution from Water over S- or N-Doped TiO2under Visible Light, Int. J. Photoenergy, 2008, 2008, 1–7 CrossRef.
- K. Nishijima, T. Kamai, N. Murakami, T. Tsubota and T. Ohno, Photocatalytic Hydrogen or Oxygen Evolution from Water over S- or N-Doped TiO2 under Visible Light, Int. J. Photoenergy, 2008, 2008, 7 CrossRef.
- H. Bai, K. S. Y. Kwan, Z. Liu, X. Song, S. S. Lee and D. D. Sun, Facile synthesis of hierarchically meso/nanoporous s- and c-codoped TiO2 and its high photocatalytic efficiency in H2 generation, Appl. Catal., A, 2013, 129, 294–300 CrossRef CAS.
- W. Hao and P. L. James, Effects of dopant states on photoactivity in carbon-doped TiO 2, J. Phys.: Condens. Matter, 2005, 17, L209 CrossRef.
- S. U. Khan, M. Al-Shahry and W. B. Ingler Jr., Efficient photochemical water splitting by a chemically modified n-TiO2, Science, 2002, 297, 2243–2245 CrossRef CAS PubMed.
- Y. Choi, T. Umebayashi and M. Yoshikawa, Fabrication and characterization of C-doped anatase TiO2 photocatalysts, J. Mater. Sci., 2004, 39, 1837–1839 CrossRef CAS.
- O. Diwald, T. L. Thompson, T. Zubkov, S. D. Walck and J. T. Yates, Photochemical Activity of Nitrogen-Doped Rutile TiO2(110) in Visible Light, J. Phys. Chem. B, 2004, 108, 6004–6008 CrossRef CAS.
- T. Tachikawa, S. Tojo, K. Kawai, M. Endo, M. Fujitsuka, T. Ohno, K. Nishijima, Z. Miyamoto and T. Majima, Photocatalytic Oxidation Reactivity of Holes in the Sulfur- and Carbon-Doped TiO2 Powders Studied by Time-Resolved Diffuse Reflectance Spectroscopy, J. Phys. Chem. B, 2004, 108, 19299–19306 CrossRef CAS.
- Z. J. Daimei Chen, G. Jiaqing, W. Qun and Y. Dong, Carbon and Nitrogen Co-doped TiO2 with Enhanced Visible-Light Photocatalytic Activity, Ind. Eng. Chem. Res., 2007, 46, 2741–2746 CrossRef.
- S.-H. Liu and H.-R. Syu, High visible-light photocatalytic hydrogen evolution of C,N-codoped mesoporous TiO2 nanoparticles prepared via an ionic-liquid-template approach, Int. J. Hydrogen Energy, 2013, 38, 13856–13865 CrossRef CAS.
- F. Z. Jia, Z. P. Yao and Z. H. Jiang, Carbon Coated N-Doping of TiO2 Nanotube Films with Enhanced Visible-Light Photocatalytic Activity, Adv. Mater. Res., 2012, 512-515, 1564–1567 CAS.
- M. Khannam, S. Sharma, S. Dolui and S. K. Dolui, A graphene oxide incorporated TiO2 photoanode for high efficiency quasi solid state dye sensitized solar cells based on a poly-vinyl alcohol gel electrolyte, RSC Adv., 2016, 6, 55406–55414 RSC.
- N. Zhang, Y. Zhang and Y. J. Xu, Recent progress on graphene-based photocatalysts: current status and future perspectives, Nanoscale, 2012, 4, 5792–5813 RSC.
- B. F. Machado and P. Serp, Graphene-based materials for catalysis, Catal. Sci. Technol., 2012, 2, 54–75 RSC.
- Y. Liu, X. Dong and P. Chen, Biological and chemical sensors based on graphene materials, Chem. Soc. Rev., 2012, 41, 2283–2307 RSC.
- Q. Xiang, J. Yu and M. Jaroniec, Graphene-based semiconductor photocatalysts, Chem. Soc. Rev., 2012, 41, 782–796 RSC.
- P. V. Kamat, Graphene-Based Nanoassemblies for Energy Conversion, J. Phys. Chem. Lett., 2011, 2, 242–251 CrossRef CAS.
- M. Pumera, Graphene-based nanomaterials for energy storage, Energy Environ. Sci., 2011, 4, 668–674 RSC.
- F. Pei, Y. Liu, S. Xu, J. Lü, C. Wang and S. Cao, Nanocomposite of graphene oxide with nitrogen-doped TiO2 exhibiting enhanced photocatalytic efficiency for hydrogen evolution, Int. J. Hydrogen Energy, 2013, 38, 2670–2677 CrossRef CAS.
- Z. Mou, Y. Wu, J. Sun, P. Yang, Y. Du and C. Lu, TiO2 Nanoparticles-Functionalized N-Doped Graphene with Superior Interfacial Contact and Enhanced Charge Separation for Photocatalytic Hydrogen Generation, ACS Appl. Mater. Interfaces, 2014, 6, 13798–13806 CrossRef CAS PubMed.
- H. Y. Hafeez, S. K. Lakhera, S. Bellamkonda, G. R. Rao, M. V. Shankar, D. W. Bahnemann and B. Neppolian, Construction of ternary hybrid layered reduced graphene oxide supported g-C3N4-TiO2 nanocomposite and its photocatalytic hydrogen production activity, Int. J. Hydrogen Energy, 2018, 43, 3892–3904 CrossRef CAS.
- X. Wei, J. Cao and F. Fang, A novel multifunctional Ag and Sr2+ co-doped TiO2@rGO ternary nanocomposite with enhanced p-nitrophenol degradation, and bactericidal and hydrogen evolution activity, RSC Adv., 2018, 8, 31822–31829 RSC.
- X. B. Li, Q. Liu, X. Y. Jiang and J. Huang, Enhanced Photocatalytic Activity of Ga-N Co-doped Anatase TiO2 for Water Decomposition to Hydrogen, Int. J. Electrochem. Sci., 2012, 7, 11519–11527 CAS.
- Y. Gai, J. Li, S. S. Li, J. B. Xia and S. H. Wei, Design of narrow-gap TiO2: a passivated codoping approach for enhanced photoelectrochemical activity, Phys. Rev. Lett., 2009, 102, 036402 CrossRef PubMed.
- T. Umebayashi, T. Yamaki, H. Itoh and K. Asai, Analysis of electronic structures of 3d transition metal-doped TiO2 based on band calculations, J. Phys. Chem. Solids, 2002, 63, 1909–1920 CrossRef CAS.
- P. Romero-Gomez, A. Borras, A. Barranco, J. P. Espinos and A. R. Gonzalez-Elipe, Enhanced Photoactivity in Bilayer Films with Buried Rutile–Anatase Heterojunctions, ChemPhysChem, 2011, 12, 191–196 CrossRef CAS PubMed.
- W. Zhu, X. Qiu, V. Iancu, X. Q. Chen, H. Pan, W. Wang, N. M. Dimitrijevic, T. Rajh, H. M. Meyer, , 3rd, M. P. Paranthaman, G. M. Stocks, H. H. Weitering, B. Gu, G. Eres and Z. Zhang, Band gap narrowing of titanium oxide semiconductors by noncompensated anion-cation codoping for enhanced visible-light photoactivity, Phys. Rev. Lett., 2009, 103, 226401 CrossRef PubMed.
- Y. Sakatani, J. Nunoshige, H. Ando, K. Okusako, H. Koike, T. Takata, J. N. Kondo, M. Hara and K. Domen, Photocatalytic Decomposition of Acetaldehyde under Visible Light Irradiation over La3+ and N Co-doped TiO2, Chem. Lett., 2003, 32, 1156–1157 CrossRef CAS.
- K. Nagaveni, M. S. Hegde, N. Ravishankar, G. N. Subbanna and G. Madras, Synthesis and structure of nanocrystalline TiO2 with lower band gap showing high photocatalytic activity, Langmuir, 2004, 20, 2900–2907 CrossRef CAS PubMed.
- M. Sathish, B. Viswanathan, R. P. Viswanath and C. S. Gopinath, Synthesis, Characterization, Electronic Structure, and Photocatalytic Activity of Nitrogen-Doped TiO2 Nanocatalyst, Chem. Mater., 2005, 17, 6349–6353 CrossRef CAS.
- X. Chen and C. Burda, Photoelectron Spectroscopic Investigation of Nitrogen-Doped Titania Nanoparticles, J. Phys. Chem. B, 2004, 108, 15446–15449 CrossRef CAS.
- M. M. Joshi, N. K. Labhsetwar, P. A. Mangrulkar, S. N. Tijare, S. P. Kamble and S. S. Rayalu, Visible light induced photoreduction of methyl orange by N-doped mesoporous titania, Appl. Catal., A, 2009, 357, 26–33 CrossRef CAS.
- J. L. Gole, J. D. Stout, C. Burda, Y. Lou and X. Chen, Highly Efficient Formation of Visible Light Tunable TiO2-xNx Photocatalysts and Their Transformation at the Nanoscale, J. Phys. Chem. B, 2004, 108, 1230–1240 CrossRef CAS.
- M. Seyler, K. Stoewe and W. F. Maier, New hydrogen-producing photocatalysts—A combinatorial search, Appl. Catal., B, 2007, 76, 146–157 CrossRef CAS.
- G. Impellizzeri, V. Scuderi, L. Romano, P. M. Sberna, E. Arcadipane, R. Sanz, M. Scuderi, G. Nicotra, M. Bayle, R. Carles, F. Simone and V. Privitera, Fe ion-implanted TiO2 thin film for efficient visible-light photocatalysis, J. Appl. Phys., 2014, 116, 173507 CrossRef.
- H. B. Jalali, L. Trabzon and H. Kizil, Characterization and Comparison of Visible Light Active Fe-Doped and N-doped TiO2 Films Prepared by Sol-Gel Process, Aust. J. Basic Appl. Sci., 2014, 8(23), 259–263 CAS.
- J. Yu, Q. Xiang and M. Zhou, Preparation, characterization and visible-light-driven photocatalytic activity of Fe-doped titania nanorods and first-principles study for electronic structures, Appl. Catal., A, 2009, 90, 595–602 CrossRef CAS.
- R. Sasikala, A. R. Shirole, V. Sudarsan, Jagannath, C. Sudakar, R. Naik, R. Rao and S. R. Bharadwaj, Enhanced photocatalytic activity of indium and nitrogen co-doped TiO2–Pd nanocomposites for hydrogen generation, Appl. Catal., A, 2010, 377, 47–54 CrossRef CAS.
- F. Meng, J. Li, Z. Hong, M. Zhi, A. Sakla, C. Xiang and N. Wu, Photocatalytic generation of hydrogen with visible-light nitrogen-doped lanthanum titanium oxides, Catal. Today, 2013, 199, 48–52 CrossRef CAS.
- X. Zhang, Y. Chen, Y. Xiao, W. Zhou, G. Tian and H. Fu, Enhanced charge transfer and separation of hierarchical hydrogenated TiO2 nanothorns/carbon nanofibers composites decorated by NiS quantum dots for remarkable photocatalytic H2 production activity, Nanoscale, 2018, 10, 4041–4050 RSC.
- S. K. Parayil, H. S. Kibombo and R. T. Koodali, Naphthalene derivatized TiO2–carbon hybrid materials for efficient photocatalytic splitting of water, Catal. Today, 2013, 199, 8–14 CrossRef CAS.
- S. K. Parayil, H. S. Kibombo, C.-M. Wu, R. Peng, J. Baltrusaitis and R. T. Koodali, Enhanced photocatalytic water splitting activity of carbon-modified TiO2 composite materials synthesized by a green synthetic approach, Int. J. Hydrogen Energy, 2012, 37, 8257–8267 CrossRef CAS.
- K.-J. Chao, W.-Y. Cheng, T.-H. Yu and S.-Y. Lu, Large enhancements in hydrogen production of TiO2 through a simple carbon decoration, Carbon, 2013, 62, 69–75 CrossRef CAS.
- Y. Zang, L. Li, Y. Xu, Y. Zuo and G. Li, Hybridization of brookite TiO2 with g-C3N4: a visible-light-driven photocatalyst for As3+ oxidation, MO degradation and water splitting for hydrogen evolution, J. Mater. Chem. A, 2014, 2, 15774–15780 RSC.
- Z. Yu, J. Meng, Y. Li and Y. Li, Efficient photocatalytic hydrogen production from water over a CuO and carbon fiber comodified TiO2 nanocomposite photocatalyst, Int. J. Hydrogen Energy, 2013, 38, 16649–16655 CrossRef CAS.
- Q. Wang, G. Yun, Y. Bai, N. An, Y. Chen, R. Wang, Z. Lei and W. Shangguan, CuS, NiS as co-catalyst for enhanced photocatalytic hydrogen evolution over TiO2, Int. J. Hydrogen Energy, 2014, 39, 13421–13428 CrossRef CAS.
- X.-J. Lv, S.-X. Zhou, C. Zhang, H.-X. Chang, Y. Chen and W.-F. Fu, Synergetic effect of Cu and graphene as cocatalyst on TiO2 for enhanced photocatalytic hydrogen evolution from solar water splitting, J. Mater. Chem., 2012, 22, 18542–18549 RSC.
- G. Nagaraju, K. Manjunath, S. Sarkar, E. Gunter, S. R. Teixeira and J. Dupont, TiO2–RGO hybrid nanomaterials for enhanced water splitting reaction, Int. J. Hydrogen Energy, 2015, 40, 12209–12216 CrossRef CAS.
- P. Cheng, Z. Yang, H. Wang, W. Cheng, M. Chen, W. Shangguan and G. Ding, TiO2–graphene nanocomposites for photocatalytic hydrogen production from splitting water, Int. J. Hydrogen Energy, 2012, 37, 2224–2230 CrossRef CAS.
- Y. Yang, E. Liu, H. Dai, L. Kang, H. Wu, J. Fan, X. Hu and H. Liu, Photocatalytic activity of Ag–TiO2-graphene ternary nanocomposites and application in hydrogen evolution by water splitting, Int. J. Hydrogen Energy, 2014, 39, 7664–7671 CrossRef CAS.
- M. Lindén, J. Blanchard, S. Schacht, S. A. Schunk and F. Schüth, Phase Behavior and Wall Formation in Zr(SO4)2/CTABr and TiOSO4/CTABr Mesophases, Chem. Mater., 1999, 11, 3002–3008 CrossRef.
- Y. Yao, G. Li, S. Ciston, R. M. Lueptow and K. A. Gray, Photoreactive TiO2/carbon nanotube composites: synthesis and reactivity, Environ. Sci. Technol., 2008, 42, 4952–4957 CrossRef CAS PubMed.
- D. Ke, P. Tianyou, K. Dingning and W. Bingqing, Photocatalytic hydrogen generation using a nanocomposite of multi-walled carbon nanotubes and TiO 2 nanoparticles under visible light irradiation, Nanotechnology, 2009, 20, 125603 CrossRef PubMed.
- C. Zhao, H. Luo, F. Chen, P. Zhang, L. Yi and K. You, A novel composite of TiO2 nanotubes with remarkably high efficiency for hydrogen production in solar-driven water splitting, Energy Environ. Sci., 2014, 7, 1700–1707 RSC.
- A. Berko, I. Ulrych and K. C. Prince, Encapsulation of Rh Nanoparticles Supported on TiO2(110)-(1 × 1) Surface: XPS and STM Studies, J. Phys. Chem. B, 1998, 102, 3379–3386 CrossRef CAS.
- Y.-C. Hsu, H.-C. Lin, C.-W. Lue, Y.-T. Liao and C.-M. Yang, A novel synthesis of carbon-coated anatase nanocrystals showing high adsorption capacity and photocatalytic activity, Appl. Catal., A, 2009, 89, 309–314 CrossRef CAS.
- N. Li, Y. Ma, B. Wang, Y. Huang, Y. Wu, X. Yang and Y. Chen, Synthesis of semiconducting SWNTs by arc discharge and their enhancement of water splitting performance with TiO2 photocatalyst, Carbon, 2011, 49, 5132–5141 CrossRef CAS.
- H. Yu, J. Yu, S. Liu and S. Mann, Template-free Hydrothermal Synthesis of CuO/Cu2O Composite Hollow Microspheres, Chem. Mater., 2007, 19, 4327–4334 CrossRef CAS.
- B. Li, Y. Hao, B. Zhang, X. Shao and L. Hu, A multifunctional noble-metal-free catalyst of CuO/TiO2 hybrid nanofibers, Appl. Catal., A, 2017, 531, 1–12 CrossRef CAS.
- J. Nie, A. O. T. Patrocinio, S. Hamid, F. Sieland, J. Sann, S. Xia, D. W. Bahnemann and J. Schneider, New insights into the plasmonic enhancement for photocatalytic H2 production by Cu–TiO2 upon visible light illumination, Phys. Chem. Chem. Phys., 2018, 20, 5264–5273 RSC.
- G. D. Moon, J. B. Joo, I. Lee and Y. Yin, Decoration of size-tunable CuO nanodots on TiO2 nanocrystals for noble metal-free photocatalytic H2 production, Nanoscale, 2014, 6, 12002–12008 RSC.
- I. Mondal and U. Pal, Synthesis of MOF templated Cu/CuO@TiO2 nanocomposites for synergistic hydrogen production, Phys. Chem. Chem. Phys., 2016, 18, 4780–4788 RSC.
- S. S. Lee, H. Bai, Z. Liu and D. D. Sun, Novel-structured electrospun
TiO2/CuO composite nanofibers for high efficient photocatalytic cogeneration of clean water and energy from dye wastewater, Water Res., 2013, 47, 4059–4073 CrossRef CAS PubMed.
- Z. Jin, X. Zhang, Y. Li, S. Li and G. Lu, 5.1% Apparent quantum efficiency for stable hydrogen generation over eosin-sensitized CuO/TiO2 photocatalyst under visible light irradiation, Catal. Commun., 2007, 8, 1267–1273 CrossRef CAS.
- S. Xu, A. J. Du, J. Liu, J. Ng and D. D. Sun, Highly efficient CuO incorporated TiO2 nanotube photocatalyst for hydrogen production from water, Int. J. Hydrogen Energy, 2011, 36, 6560–6568 CrossRef CAS.
- L. Yu, S. Yuan, L. Shi, Y. Zhao and J. Fang, Synthesis of Cu2+ doped mesoporous titania and investigation of its photocatalytic ability under visible light, Microporous Mesoporous Mater., 2010, 134, 108–114 CrossRef CAS.
- S. Zhang, H. Wang, M. Yeung, Y. Fang, H. Yu and F. Peng, Cu(OH)2-modified TiO2 nanotube arrays for efficient photocatalytic hydrogen production, Int. J. Hydrogen Energy, 2013, 38, 7241–7245 CrossRef CAS.
- C. Wang, Q. Hu, J. Huang, C. Zhu, Z. Deng, H. Shi, L. Wu, Z. Liu and Y. Cao, Enhanced hydrogen production by water splitting using Cu-doped TiO2 film with preferred (001) orientation, Appl. Surf. Sci., 2014, 292, 161–164 CrossRef CAS.
- S. Xu, J. Ng, X. Zhang, H. Bai and D. D. Sun, Fabrication and comparison of highly efficient Cu incorporated TiO2 photocatalyst for hydrogen generation from water, Int. J. Hydrogen Energy, 2010, 35, 5254–5261 CrossRef CAS.
- H. Chen, Z.-G. Gu, S. Mirza, S.-H. Zhang and J. Zhang, Hollow Cu–TiO2/C nanospheres derived from a Ti precursor encapsulated MOF coating for efficient photocatalytic hydrogen evolution, J. Mater. Chem. A, 2018, 6, 7175–7181 RSC.
- R. Schaub, P. Thostrup, N. Lopez, E. Laegsgaard, I. Stensgaard, J. K. Norskov and F. Besenbacher, Oxygen vacancies as active sites for water dissociation on rutile TiO(2)(110), Phys. Rev. Lett., 2001, 87, 266104 CrossRef CAS PubMed.
- M. Chandra, K. Bhunia and D. Pradhan, Controlled Synthesis of CuS/TiO2 Heterostructured Nanocomposites for Enhanced Photocatalytic Hydrogen Generation through Water Splitting, Inorg. Chem., 2018, 57, 4524–4533 CrossRef CAS PubMed.
- V. Polliotto, S. Livraghi, A. Krukowska, M. V. Dozzi, A. Zaleska-Medynska, E. Selli and E. Giamello, Copper-Modified TiO2 and ZrTiO4: Cu Oxidation State Evolution during Photocatalytic Hydrogen Production, ACS Appl. Mater. Interfaces, 2018, 10, 27745–27756 CrossRef CAS PubMed.
- C. Castañeda, F. Tzompantzi, A. Rodríguez-Rodríguez, M. Sánchez-Dominguez and R. Gómez, Improved photocatalytic hydrogen production from methanol/water solution using CuO supported on fluorinated TiO2, J. Chem. Technol. Biotechnol., 2018, 93, 1113–1120 CrossRef.
- S. Taylor, M. Mehta, D. Barbash and A. Samokhvalov, One-pot photoassisted synthesis, in situ photocatalytic testing for hydrogen generation and the mechanism of binary nitrogen and copper promoted titanium dioxide, Photochem. Photobiol. Sci., 2017, 16, 916–924 RSC.
- J. Yu and J. Ran, Facile preparation and enhanced photocatalytic H2-production activity of Cu(OH)2 cluster modified TiO2, Energy Environ. Sci., 2011, 4, 1364–1371 RSC.
- S. A. Rawool, M. R. Pai, A. M. Banerjee, R. D. Bapat, C. Nayak and A. K. Tripathi, Lab scale optimization of various factors for photocatalytic hydrogen generation over low cost Cu 0.02 Ti 0.98 O 2-δ photocatalyst under UV/Visible irradiation and sunlight, Int. J. Hydrogen Energy, 2018, 43, 1271–1284 CrossRef CAS.
- L. Xiong, F. Yang, L. Yan, N. Yan, X. Yang, M. Qiu and Y. Yu, Bifunctional photocatalysis of TiO2/Cu2O composite under visible light: Ti3+ in organic pollutant degradation and water splitting, J. Phys. Chem. Solids, 2011, 72, 1104–1109 CrossRef CAS.
- A. J. Simamora, T. L. Hsiung, F. C. Chang, T. C. Yang, C. Y. Liao and H. P. Wang, Photocatalytic splitting of seawater and degradation of methylene blue on CuO/nano TiO2, Int. J. Hydrogen Energy, 2012, 37, 13855–13858 CrossRef CAS.
- S. Guo, S. Han, H. Mao, S. Dong, C. Wu, L. Jia, B. Chi, J. Pu and J. Li, Structurally controlled ZnO/TiO2 heterostructures as efficient photocatalysts for hydrogen generation from water without noble metals: The role of microporous amorphous/crystalline composite structure, J. Power Sources, 2014, 245, 979–985 CrossRef CAS.
- A. Pérez-Larios, R. Lopez, A. Hernández-Gordillo, F. Tzompantzi, R. Gómez and L. M. Torres-Guerra, Improved hydrogen production from water splitting using TiO2–ZnO mixed oxides photocatalysts, Fuel, 2012, 100, 139–143 CrossRef.
- N. A. M. Barakat, A. Taha, M. Motlak, M. M. Nassar, M. S. Mahmoud, S. S. Al-Deyab, M. El-Newehy and H. Y. Kim, ZnO&Fe2O3-incoportaed TiO2 nanofibers as super effective photocatalyst for water splitting under visible light radiation, Appl. Catal., A, 2014, 481, 19–26 CrossRef CAS.
- R. Wang, H. Tan, Z. Zhao, G. Zhang, L. Song, W. Dong and Z. Sun, Stable ZnO@TiO2 core/shell nanorod arrays with exposed high energy facets for self-cleaning coatings with anti-reflective properties, J. Mater. Chem. A, 2014, 2, 7313–7318 RSC.
- Y. Ling, G. Wang, D. A. Wheeler, J. Z. Zhang and Y. Li, Sn-doped hematite nanostructures for photoelectrochemical water splitting, Nano Lett., 2011, 11, 2119–2125 CrossRef CAS PubMed.
- J. Brillet, M. Grätzel and K. Sivula, Decoupling Feature Size and Functionality in Solution-Processed, Porous Hematite Electrodes for Solar Water Splitting, Nano Lett., 2010, 10, 4155–4160 CrossRef CAS PubMed.
- I. Cesar, K. Sivula, A. Kay, R. Zboril and M. Grätzel, Influence of Feature Size, Film Thickness, and Silicon Doping on the Performance of Nanostructured Hematite Photoanodes for Solar Water Splitting, J. Phys. Chem. C, 2009, 113, 772–782 CrossRef CAS.
- X. Qian, X. Zhang, Y. Bai, T. Li, X. Tang, E. Wang and S. Dong, Photoelectrochemical Characteristics Of α-Fe2O3 Nanocrystalline Semiconductor Thin Film, J. Nanopart. Res., 2000, 2, 191–198 CrossRef CAS.
- M. P. Dare-Edwards, J. B. Goodenough, A. Hamnett and P. R. Trevellick, Electrochemistry and photoelectrochemistry of iron(III) oxide, J. Chem. Soc., Faraday Trans. 1, 1983, 79, 2027–2041 RSC.
- K. Sivula, R. Zboril, F. Le Formal, R. Robert, A. Weidenkaff, J. Tucek, J. Frydrych and M. Grätzel, Photoelectrochemical Water Splitting with Mesoporous Hematite Prepared by a Solution-Based Colloidal Approach, J. Am. Chem. Soc., 2010, 132, 7436–7444 CrossRef CAS PubMed.
- K. Maeda, T. Takata, M. Hara, N. Saito, Y. Inoue, H. Kobayashi and K. Domen, GaN:ZnO Solid Solution as a Photocatalyst for Visible-Light-Driven Overall Water Splitting, J. Am. Chem. Soc., 2005, 127, 8286–8287 CrossRef CAS PubMed.
- A. M. Hussein and R. V. Shende, Enhanced hydrogen generation using ZrO2-modified coupled ZnO/TiO2 nanocomposites in the absence of noble metal co-catalyst, Int. J. Hydrogen Energy, 2014, 39, 5557–5568 CrossRef CAS.
- H. Navío, G. Colón, S. G. Botta and M. I. Litter, Preparation and Physicochemical Properties of ZrO2 and Fe/ZrO2 Prepared by a Sol−Gel Technique, Langmuir, 2001, 17, 202–210 CrossRef.
- S. C. Surakerk Onsuratooma and S. Thammanoon, Hydrogen production from water splitting under UV lightirradiation over Ag-loaded mesoporous-assembled TiO2-ZrO2 mixed oxide nanocrystal photocatalysts, Int. J. Hydrogen Energy, 2011, 36, 5246–5261 CrossRef.
- R. Shwetharani, C. A. N. Fernando and G. R. Balakrishna, Excellent hydrogen evolution by a multi approach via structure–property tailoring of titania, RSC Adv., 2015, 5, 39122–39130 RSC.
- N. Vinothkumar and M. De, Hydrogen production from water–methanol solution over visible light active indium–titanium oxide photocatalysts modified with copper oxide, Int. J. Hydrogen Energy, 2014, 39, 11494–11500 CrossRef CAS.
- Y. Yu, L. Zhang, J. Wang, Z. Yang, M. Long, N. Hu and Y. Zhang, Preparation of hollow porous Cu2O microspheres and photocatalytic activity under visible light irradiation, Nanoscale Res. Lett., 2012, 7, 347 CrossRef PubMed.
- W. Zhao, Y. Wang, Y. Yang, J. Tang and Y. Yang, Carbon spheres supported visible-light-driven CuO-BiVO4 heterojunction: Preparation, characterization, and photocatalytic properties, Appl. Catal., A, 2012, 115-116, 90–99 CrossRef CAS.
- L. Huang, F. Peng, H. Wang, H. Yu and Z. Li, Preparation and characterization of Cu2O/TiO2 nano–nano heterostructure photocatalysts, Catal. Commun., 2009, 10, 1839–1843 CrossRef CAS.
- S. Qin, F. Xin, Y. Liu, X. Yin and W. Ma, Photocatalytic reduction of CO2 in methanol to methyl formate over CuO–TiO2 composite catalysts, J. Colloid Interface Sci., 2011, 356, 257–261 CrossRef CAS PubMed.
- Y. Jiang, X. Jing, K. Zhu, Z. Peng, J. Zhang, Y. Liu, W. Zhang, L. Ni and Z. Liu, Ta3N5 nanoparticles/TiO2 hollow sphere (0D/3D) heterojunction: facile synthesis and enhanced photocatalytic activities of levofloxacin degradation and H2 evolution, Dalton Trans., 2018, 47(37), 13113–13125 RSC.
- M. Stodolny and M. Laniecki, Synthesis and characterization of mesoporous Ta2O5–TiO2 photocatalysts for water splitting, Catal. Today, 2009, 142, 314–319 CrossRef CAS.
- C.-M. Wu, R. Peng, N. M. Dimitrijevic, T. Rajh and R. T. Koodali, Preparation of TiO2–SiO2 aperiodic mesoporous materials with controllable formation of tetrahedrally coordinated Ti4+ ions and their performance for photocatalytic hydrogen production, Int. J. Hydrogen Energy, 2014, 39, 127–136 CrossRef CAS.
- N. Rungjaroentawon, S. Onsuratoom and S. Chavadej, Hydrogen production from water splitting under visible light irradiation using sensitized mesoporous-assembled TiO2–SiO2 mixed oxide photocatalysts, Int. J. Hydrogen Energy, 2012, 37, 11061–11071 CrossRef CAS.
- S. Hu, F. Li, Z. Fan and J. Gui, Improved photocatalytic hydrogen production property over Ni/NiO/N–TiO2−x heterojunction nanocomposite prepared by NH3 plasma treatment, J. Power Sources, 2014, 250, 30–39 CrossRef CAS.
- D. Xu, Y. Hai, X. Zhang, S. Zhang and R. He, Bi 2 O 3 cocatalyst improving photocatalytic hydrogen evolution performance of TiO 2, Appl. Surf. Sci., 2017, 400, 530–536 CrossRef CAS.
- J. Yan, Q. Liu, L. Guan, F. Liang and H. Gu, Photocatalytic hydrogen generation of Pt-Sr(Zr1 − x Y x )O3 − δ -TiO2 heterojunction under the irradiation of simulated sunlight, Front. Chem. China, 2009, 4, 121–126 CrossRef.
- Y. Li, Z. Yu, J. Meng and Y. Li, Enhancing the activity of a SiC–TiO2 composite catalyst for photo-stimulated catalytic water splitting, Int. J. Hydrogen Energy, 2013, 38, 3898–3904 CrossRef CAS.
- X. Fan, B. Lin, H. Liu, L. He, Y. Chen and B. Gao, Remarkable promotion of photocatalytic hydrogen evolution from water on TiO2-pillared titanoniobate, Int. J. Hydrogen Energy, 2013, 38, 832–839 CrossRef CAS.
- Q. Wang, N. An, Y. Bai, H. Hang, J. Li, X. Lu, Y. Liu, F. Wang, Z. Li and Z. Lei, High photocatalytic hydrogen production from methanol aqueous solution using the photocatalysts CuS/TiO2, Int. J. Hydrogen Energy, 2013, 38, 10739–10745 CrossRef CAS.
- J. S. Jang, W. Li, S. H. Oh and J. S. Lee, Fabrication of CdS/TiO2 nano-bulk composite photocatalysts for hydrogen production from aqueous H2S solution under visible light, Chem. Phys. Lett., 2006, 425, 278–282 CrossRef CAS.
- H. Feng, S. Zhang, X. Zhang, B. Liu and N. Tang, Highly efficient visible-light-induced photoactivity of the CdS–Mn/MoS2/CdTe/TiO2 quaternary photocatalyst for label-free immunoassay of tris-(2,3-dibromopropyl) isocyanurate and enhanced solar hydrogen generation, Anal. Methods, 2018, 10, 3462–3469 RSC.
- L. Jing, J. Zhou, J. R. Durrant, J. Tang, D. Liu and H. Fu, Dynamics of photogenerated charges in the phosphate modified TiO2 and the enhanced activity for photoelectrochemical water splitting, Energy Environ. Sci., 2012, 5, 6552–6558 RSC.
- W. Sun, Q. Meng, L. Jing, D. Liu and Y. Cao, Facile Synthesis of Surface-Modified Nanosized α-Fe2O3 as Efficient Visible Photocatalysts and Mechanism Insight, J. Phys. Chem. C, 2013, 117, 1358–1365 CrossRef CAS.
- P. Luan, M. Xie, X. Fu, Y. Qu, X. Sun and L. Jing, Improved photoactivity of TiO2–Fe2O3 nanocomposites for visible-light water splitting after phosphate bridging and its mechanism, Phys. Chem. Chem. Phys., 2015, 17, 5043–5050 RSC.
- P. Luana, M. Xie, X. Fua, Y. Qu, X. Sun and L. Jing, Improved photoactivities of TiO2/Fe2O3 nanocomposites for visible-light water splitting after phosphate bridging and mechanism, Phys. Chem. Chem. Phys., 2015, 17, 5043–5050 RSC.
- M. Xie, Y. Feng, X. Fu, P. Luan and L. Jing, Phosphate-bridged TiO2–BiVO4 nanocomposites with exceptional visible activities for photocatalytic water splitting, J. Alloys Compd., 2015, 631, 120–124 CrossRef CAS.
- H. Kato and A. Kudo, Visible-Light-Response and Photocatalytic Activities of TiO2 and SrTiO3 Photocatalysts Codoped with Antimony and Chromium, J. Phys. Chem. B, 2002, 106, 5029–5034 CrossRef CAS.
- Y. Miseki, H. Kato and A. Kudo, Water splitting into H2 and O2 over niobate and titanate photocatalysts with (111) plane-type layered perovskite structure, Energy Environ. Sci., 2009, 2, 306–314 RSC.
- A. Kudo, Photocatalyst Materials for Water Splitting, Catal. Surv. Asia, 2003, 7, 31–38 CrossRef CAS.
- Y. Zhong, K. Ueno, Y. Mori, T. Oshikiri and H. Misawa, Cocatalyst Effects on Hydrogen Evolution in a Plasmon-Induced Water-Splitting System, J. Phys. Chem. C, 2015, 119, 8889–8897 CrossRef CAS.
- J. Yang, D. Wang, H. Han and C. Li, Roles of Cocatalysts in Photocatalysis and Photoelectrocatalysis, Acc. Chem. Res., 2013, 46, 1900–1909 CrossRef CAS PubMed.
- A. Fujishima and K. Honda, Electrochemical Photolysis of Water at a Semiconductor Electrode, Nature, 1972, 238, 37–38 CrossRef CAS PubMed.
- S. Shuang, R. Lv, Z. Xie and Z. Zhang, Surface Plasmon Enhanced Photocatalysis of Au/Pt-decorated TiO2 Nanopillar Arrays, Sci. Rep., 2016, 6, 26670 CrossRef CAS PubMed.
- A. Zielińska-Jurek, Progress, Challenge, and Perspective of Bimetallic TiO2-Based Photocatalysts, J. Nanomater., 2014, 2014, 1–17 CrossRef.
- Z. Chang and G. Thornton, Effect of Pd on the interaction of formic acid with TiO2(110), Surf. Sci. Rep., 2000, 459, 303–309 CrossRef CAS.
- K. D. Schierbaum, S. Fischer, M. C. Torquemada, J. L. de Segovia, E. Roman and J. A. Martin-Gago, The interaction of Pt with TIO2(110) surfaces: a comparative XPS, UPS, ISS, and ESD study, Surf. Sci. Rep., 1996, 345, 261–273 CrossRef CAS.
- A. S. Hainer, J. S. Hodgins, V. Sandre, M. Vallieres, A. E. Lanterna and J. C. Scaiano, Photocatalytic Hydrogen Generation Using Metal-Decorated TiO2: Sacrificial Donors vs True Water Splitting, ACS Energy Lett., 2018, 3, 542–545 CrossRef CAS.
- S. Y. Toledo Camacho, A. Rey, M. D. Hernández-Alonso, J. Llorca, F. Medina and S. Contreras, Pd/TiO 2 -WO 3 photocatalysts for hydrogen generation from water-methanol mixtures, Appl. Surf. Sci., 2018, 455, 570–580 CrossRef CAS.
- J. Zhang, Z. Yu, Z. Gao, H. Ge, S. Zhao, C. Chen, S. Chen, X. Tong, M. Wang, Z. Zheng and Y. Qin, Porous TiO2 Nanotubes with Spatially Separated Platinum and CoOx Cocatalysts Produced by Atomic Layer Deposition for Photocatalytic Hydrogen Production, Angew. Chem., Int. Ed., 2017, 56, 816–820 CrossRef CAS PubMed.
- D. P. Serrano, G. Calleja, P. Pizarro and P. Gálvez, Enhanced photocatalytic hydrogen production by improving the Pt dispersion over mesostructured TiO2, Int. J. Hydrogen Energy, 2014, 39, 4812–4819 CrossRef CAS.
- Z. Li, B. Tian, W. Zhen, Y. Wu and G. Lu, Inhibition of hydrogen and oxygen recombination using oxygen transfer reagent hemin chloride in Pt/TiO2 dispersion for photocatalytic hydrogen generation, Appl. Catal., A, 2017, 203, 408–415 CrossRef CAS.
- P. Wei, J. Liu and Z. Li, Effect of Pt loading and calcination temperature on the photocatalytic hydrogen production activity of TiO2 microspheres, Ceram. Int., 2013, 39, 5387–5391 CrossRef CAS.
- J. Fang, F. Shi, J. Bu, J. Ding, S. Xu, J. Bao, Y. Ma, Z. Jiang, W. Zhang, C. Gao and W. Huang, One-Step Synthesis of Bifunctional TiO2 Catalysts and Their Photocatalytic Activity, J. Phys. Chem. C, 2010, 114, 7940–7948 CrossRef CAS.
- N. T. Nguyen, J. Yoo, M. Altomare and P. Schmuki, “Suspended” Pt nanoparticles over TiO2 nanotubes for enhanced photocatalytic H2 evolution, Chem. Commun., 2014, 50, 9653–9656 RSC.
- K. Maeda, Photocatalytic properties of rutile TiO2 powder for overall water splitting, Catal. Sci. Technol., 2014, 4, 1949–1953 RSC.
- R.-J. Wu, Y.-C. Hsieh, H.-C. Hung, C. Ie and M. Chavali, Visible Light Photocatalytic Activity of Pt/N-TiO2 towards Enhanced H2 Production from Water Splitting, J. Chin. Chem. Soc., 2014, 61, 495–500 CrossRef CAS.
- M. Buaki-Sogo, M. Serra, A. Primo, M. Alvaro and H. Garcia, Alginate as Template in the Preparation of Active Titania Photocatalysts, ChemCatChem, 2013, 5, 513–518 CrossRef CAS.
- A. May-Masnou, L. Soler, M. Torras, P. Salles, J. Llorca and A. Roig, Fast and Simple Microwave Synthesis of TiO2/Au Nanoparticles for Gas-Phase Photocatalytic Hydrogen Generation, Front. Chem., 2018, 6, 110 CrossRef PubMed.
- S. K. Khore, S. R. Kadam, S. D. Naik, B. B. Kale and R. S. Sonawane, Solar light active plasmonic Au@TiO2 nanocomposite with superior photocatalytic performance for H2 production and pollutant degradation, New J. Chem., 2018, 42, 10958–10968 RSC.
- S. Oros-Ruiz, R. Zanella, R. Lopez, A. Hernandez-Gordillo and R. Gomez, Photocatalytic hydrogen production by water/methanol decomposition using Au/TiO2 prepared by deposition-precipitation with urea, J. Hazard. Mater., 2013, 263(Pt 1), 2–10 CrossRef CAS PubMed.
- B. Liu, Y. Jiang, Y. Wang, S. Shang, Y. Ni, N. Zhang, M. Cao and C. Hu, Influence of dimensionality and crystallization on visible-light hydrogen production of Au@TiO2 core–shell photocatalysts based on localized surface plasmon resonance, Catal. Sci. Technol., 2018, 8, 1094–1103 RSC.
- S. S. Rayalu, D. Jose, M. V. Joshi, P. A. Mangrulkar, K. Shrestha and K. Klabunde, Photocatalytic water splitting on Au/TiO2 nanocomposites synthesized through various routes: Enhancement in photocatalytic activity due to SPR effect, Appl. Catal., A, 2013, 142–143, 684–693 CrossRef CAS.
- B. Naik, S. M. Kim, C. H. Jung, S. Y. Moon, S. H. Kim and J. Y. Park, Enhanced H2Generation of Au-Loaded, Nitrogen-Doped TiO2Hierarchical Nanostructures under Visible Light, Adv. Mater. Interfaces, 2014, 1, 1300018 CrossRef.
- A. May-Masnou, L. Soler, M. Torras, P. Salles, J. Llorca and A. Roig, Fast and Simple Microwave Synthesis of TiO2/Au Nanoparticles for Gas-Phase Photocatalytic Hydrogen Generation, Front. Chem., 2018, 6, 110 CrossRef PubMed.
- X. Wei, J. Li, Z. Liu, X. Yang, S. Naraginti, X. Xu and X. Wang, Visible light photocatalytic mineralization of 17α-ethinyl estradiol (EE2) and hydrogen evolution over silver and strontium modified TiO2 nanoparticles: mechanisms and phytotoxicity assessment, RSC Adv., 2018, 8, 4329–4339 RSC.
- Y. Hu, C. Pan, C. X. Gao, J. Fan and E. Z. Liu, Photocatalytic Water Splitting over Ag/TiO2 Nano-Wire Films, Appl. Mech. Mater., 2014, 665, 288–291 CAS.
- F. Wu, X. Hu, J. Fan, E. Liu, T. Sun, L. Kang, W. Hou, C. Zhu and H. Liu, Photocatalytic Activity of Ag/TiO2 Nanotube Arrays Enhanced by Surface Plasmon Resonance and Application in Hydrogen Evolution by Water Splitting, Plasmonics, 2013, 8, 501–508 CrossRef CAS.
- H. Soleimanzadeh, A. Niaei, D. Salari, S. M. Mousavi and A. Tarjamannejad, Performance Study f V2O5/TiO2 Mixed Metal Oxide Nanocatalysts in Selective Catalytic Reduction of Nox Prepared by Co-Precipitation Method, Procedia Mater. Sci., 2015, 11, 655–660 CrossRef CAS.
- P. N. Kapoor, A. K. Bhagi, R. S. Mulukutla and K. Klabunde, Mixed Metal Oxide Nanoparticles, Dekker Encycl. Nanosci. Nanotechnol., 2004, 2007–2015 Search PubMed.
- P. Montes-Navajas, M. Serra and H. Garcia, Influence of the irradiation wavelength on the photocatalytic activity of Au-Pt nanoalloys supported on TiO2 for hydrogen generation from water, Catal. Sci. Technol., 2013, 3, 2252–2258 RSC.
- F. Jia, Z. Yao and Z. Jiang, Solvothermal synthesis ZnS–In2S3–Ag2S solid solution coupled with TiO2−xSx nanotubes film for photocatalytic hydrogen production, Int. J. Hydrogen Energy, 2012, 37(4), 3048–3055 CrossRef CAS.
- A. Tanaka, S. Sakaguchi, K. Hashimoto and H. Kominami, Preparation of Au/TiO2 with Metal Cocatalysts Exhibiting Strong Surface Plasmon Resonance Effective for Photoinduced Hydrogen Formation under Irradiation of Visible Light, ACS Catal., 2013, 3, 79–85 CrossRef CAS.
Footnote |
† Electronic supplementary information (ESI) available. See DOI: 10.1039/c8cy01395k |
|
This journal is © The Royal Society of Chemistry 2019 |
Click here to see how this site uses Cookies. View our privacy policy here.