Photoinactivation of uncultured, indigenous enterococci†
Received
20th September 2018
, Accepted 15th November 2018
First published on 16th November 2018
Abstract
Enterococci are used to monitor recreational water quality worldwide, so understanding their fate and transport in the environment is essential to the protection of human health. As such, researchers have documented enterococci inactivation under various exposure conditions and in diverse water matrices. However, the majority of studies have been performed using lab-cultured bacteria, which are distinct from indigenous, uncultured bacteria found in the environment. Here we investigate the photoinactivation of indigenous, uncultured enterococci from a range of sources, including wastewater treatment plants (WWTPs), marine beaches, urban streams, and a wastewater-influenced pond. We concentrated indigenous enterococci from their sources using filtration and centrifugation, placed them in a clear buffer solution, and then exposed them to simulated sunlight to measure their photoinactivation rates. First order decay rate constants (k) of indigenous, uncultured enterococci spanned an order of magnitude, from 0.3 to 2.3 m2 kJUVB−1. k values of indigenous enterococci from WWTPs tended to be larger than those from surface waters. The k value of lab-cultured Enterococcus faecalis was larger than those of indigenous, uncultured enterococci from most sources. Negative associations between the fraction of pigmented enterococci and sunlight susceptibility were observed. This work suggests that caution should be taken when extending results on bacterial photoinactivation obtained using lab-cultured bacteria to environmental bacteria, and that enterococci pigmentation may be a useful metric for estimating photoinactivation rate constants.
Environmental significance
Enterococci are known to decay under sunlight exposure through a process called photoinactivation. However, it remains a challenge to predict their photoinactivation decay rates in natural water systems, which limits the development of accurate and dependable water quality models. In this study we document photoinactivation rates of indigenous populations of enterococci from diverse natural and wastewater sources. We find that photoinactivation differs among indigenous enterococci from diverse sources and that the pigmentation of enterococci communities is associated with photoinactivation rate constants.
|
1. Introduction
Enterococci are used to monitor recreational water quality, and their presence in recreational waters is associated with gastrointestinal illnesses of swimmers and surfers.1–5 In the United States, microbial pollution at recreational beaches is estimated to cause 90 million illnesses each year, resulting in an economic burden of over $2 billion.6 Microbial water quality in the natural environment varies significantly on both spatial and temporal scales.7–10 Modeling efforts that consider enterococci fate and transport aim to understand and predict enterococci concentration variability in natural systems.11–13
Sunlight is one of the most important factors affecting pathogen and pathogen indicator concentrations in surface waters.14–19 Previous work to understand the rates and mechanisms of bacterial pathogen and indicator photoinactivation has relied mostly on lab-cultured bacteria, including reference strains purchased from culture collections and strains isolated from the environment.20–27 Fewer studies have used indigenous or wastewater bacterial pathogens or indicators to study photoinactivation rates in mesocosms or in situ in surface waters.15,16,28–30 Lab-cultured, environmental strains have been observed to decay differently under sunlight exposure than lab-cultured, reference strains.21,27,31,32 For example, Nguyen et al. observed that a lab-cultured, environmental isolate of enterococci from wastewater photoinactivated significantly more slowly than the lab-cultured, reference strain Enterococcus faecalis.31 These differences may be due to phenotypic or genomic differences between strains including their degree of pigmentation.32,33
Indigenous bacteria in environmental reservoirs differ from lab-cultured bacteria in several ways. First, the lab-culturing procedure alters bacterial physiology by exposing indigenous bacteria to nutrient-rich growth conditions. This change in physiology likely has an impact on the measured photoinactivation rates,34 possibly by altering intracellular concentrations of photosensitizers.21 Second, bacteria in lab cultures are in active growth states and are therefore phenotypically different from bacteria in environmental waters, which are more likely to be expressing non-growing phenotypes due to nutrient limitations. Bacteria in non-growing states have been shown to be more resistant to a range of environmental stresses.35,36 Third, bacteria in lab cultures have likely not been previously exposed to the range of stresses present in environmental waters. Sublethal exposure to stresses can increase resistance to those stresses.37 Despite these biases that may be introduced during lab-culturing, few studies have investigated photoinactivation of indigenous enterococci in the laboratory without first lab-culturing isolates,31,38–42 and in almost all cases, indigenous enterococci in those studies were sourced from a single wastewater or fecal source.31,38,39,42 Field studies of indigenous enterococci photoinactivation almost exclusively examine photoinactivation of wastewater enterococci.15,28,30
The goal of this research is to investigate the photoinactivation kinetics of indigenous, uncultured enterococci from a broad range of aqueous environments including WWTPs and surface waters. Enterococci were concentrated from waters using filtration and centrifugation; they were not sub-cultured or exposed to exogenous nutrient sources, in order to maintain their environmental physiological profile as much as possible. We then measured enterococci photoinactivation in a lab-controlled system. Finally, as enterococci pigmentation has been previously associated with decreased photoinactivation rate constants,31,32 we measured the fraction of pigmented enterococci in each sample and investigated the impact of pigmentation on photoinactivation.
2. Methods
2.1 Sample collection
Samples were collected from WWTPs and surface waters between September 2017 and February 2018 (Fig. 1 and Table S1†). All surface water samples were collected within one hour of sunrise. Two liter samples of primary treated effluent were collected at WWTPs, and 20–40 L samples were collected from surface waters. Surface waters included three marine beaches, three urban streams, and one wastewater-influenced pond with a large population of ducks, and are subsequently referred to as beach, stream, and pond samples, respectively. Locations were chosen based on the expected high concentrations of enterococci.18,43,44 All samples were collected in autoclaved plastic containers and placed on blue ice for transport to the laboratory. Upon arrival at the laboratory, approximately 500 mL of each sample was used to measure enterococci concentrations in the raw water using EPA Method 1600 (ref. 45) and other physicochemical parameters (see the ESI†). The remaining sample volumes were then processed to concentrate bacteria using tangential filtration and centrifugation.
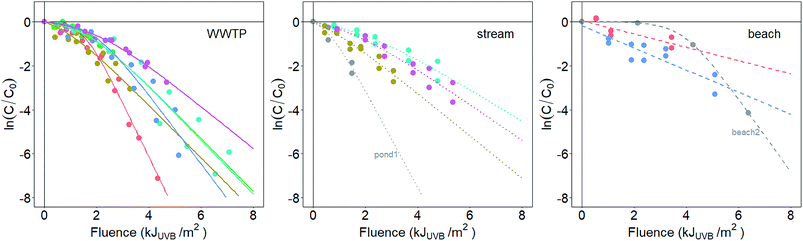 |
| Fig. 1 Photoinactivation of enterococci from WWTP (left), stream (center, including pond1 in gray), and beach (right) sources. Data in gray (samples beach2 and pond1) are excluded from averaged photoinactivation rate constants as described in the main text. ln(C/C0) = natural log-transformed relative concentration of enterococci, measured as CFU mL−1 on mEI agar. Fluence was calculated in the UVB wavelengths. Different colors correspond to photoinactivation data from experiments that used indigenous, uncultured bacteria from different sources. | |
2.2 Wastewater and surface water bacteria concentration
Bacteria were concentrated from wastewater and surface water using a combination of filtration and centrifugation. These methods were chosen because they are relatively simple and do not require exposing the bacteria to additional nutrient sources that may change the physiological profile of bacteria in the samples. As the separation methods rely on size and density, solids of similar size and density were co-concentrated with the enterococci.
Surface water samples were first concentrated using a tangential filtration membrane system (OMEGA suspended screen cassette; 30 kDa pore size; Pall Corporation, Port Washington, NY) until approximately 5 L of retentate remained. The wastewater was not concentrated first with tangential filtration due to its relatively high bacterial concentrations. Bacteria in wastewater samples and the surface water retentate were then concentrated using three rounds of centrifugation (10 minutes at 9600 × g (retaining the pellet), 5 minutes at 500 × g (retaining the supernatant), 10 minutes at 9600 × g (retaining the pellet)). The final pellet was resuspended in phosphate buffered saline (PBS, pH 6.77; Fisher Scientific, Fair Lawn, NJ) for a final volume of 10–30 mL for each sample. Concentrated bacteria were stored in the dark at 4 °C until photoinactivation experiments were performed (within 48 h of initial water sample collection) while we determined the enterococci concentration in the concentrate (which indicated the volume needed to seed the experimental reactors, as described below). See the ESI† for further details.
2.3 Photoinactivation experiments
Concentrated bacteria were diluted into PBS to a final volume of 50 mL in three sterile 100 mL beakers wrapped in black electrical tape. The volume of concentrated bacteria added to the PBS varied among experiments in order to achieve initial enterococci concentrations in the reactors that ranged between 100 and 105 CFU mL−1. Two beakers were designated experimental reactors (i.e. biological duplicates) and were exposed to full spectrum simulated sunlight in an Atlas Suntest XLS+ solar simulator (Chicago, IL) with a 1.1 kW xenon arc lamp set to an intensity of 250 W m−2 and equipped with a glass filter to remove wavelengths below 290 nm (see Fig. S1† for the spectrum which approximates the spectrum of late morning sun during the summer in Palo Alto, CA, USA). The third reactor (dark control) was covered with foil to prevent exposure to simulated sunlight and placed next to the experimental reactors in the solar simulator. The solar simulator was equipped with a recirculating water bath to maintain the reactors at 15 °C. Photoinactivation experiments were run for 40–160 minutes, depending on the expected inactivation kinetics. A time course of samples was taken from both experimental and control reactors for enterococci quantification following EPA Method 1600 (ref. 45) (see the ESI†). The number of samples collected and their volumes varied between experiments and depended on the starting enterococci concentration; our goal was to not remove more than 10% of the volume of the reactors, yet still be able to measure enterococci. 3–7 samples were taken from each experimental reactor, and 3 samples were taken from each control reactor.
2.4 Enterococci pigmentation tests
The fraction of enterococci in the raw water samples that were pigmented was quantified using a previously described method,46 with slight variation. Between 1 and 3 mEI plates that were used to measure enterococci concentrations in the raw water (prior to concentration) were chosen for each water sample, sealed with parafilm, and stored at 4 °C until pigmentation analysis (within 6 months). Individual enterococci colonies from stored mEI agar plates were sub-cultured in tryptic soy broth (TSB; Sigma-Aldrich, St Louis, MO) and then streaked onto tryptic soy agar (TSA; BD Difco, Sparks, MD). Each colony was identified as pigmented or nonpigmented by picking a small amount of biomass from the streak on a sterile cotton swab and visually examining the swab for pigmentation (see the ESI†). The total number of isolates examined for each water sample ranged from 20 to 89 (Table 1).
Table 1 Results of photoinactivation modeling and pigmentation tests. Values in parentheses represent standard error
Sample name |
k (m2 kJUVB−1) |
S (kJUVB m−2) |
Total isolates tested |
Isolates pigmented |
Fraction pigmented |
Note that light scattering may influence k and S values for this result and was not corrected.
|
WWTP1 |
2.5 (0.2) |
1.5 (0.1) |
20 |
1 |
5.0% (4.9%) |
WWTP2 |
1.2 (0.3) |
0.8 (0.4) |
27 |
0 |
0.0% (0.0%) |
WWTP3 |
1.2 (0.2) |
1.6 (0.1) |
43 |
5 |
11.6% (4.9%) |
WWTP4 |
1.2 (0.2) |
1.6 (0.5) |
47 |
6 |
12.8% (4.9%) |
WWTP5 |
1.6 (0.2) |
1.9 (0.3) |
66 |
11 |
16.7% (4.6%) |
WWTP6 |
1.0 (0.08) |
2.0 (0.1) |
28 |
2 |
7.1% (4.9%) |
pond1 |
2.2 (0.7) |
0.6 (0.3) |
21 |
4 |
19.0% (8.6%) |
beach1 |
0.3 (0.07) |
— |
23 |
8 |
34.8% (9.9%) |
beach2a |
1.7 (0.007) |
3.9 (0.008) |
67 |
21 |
31.3% (5.7%) |
beach3 |
0.5 (0.07) |
— |
37 |
13 |
35.1% (7.8%) |
stream1 |
1.0 (0.1) |
0.6 (0.3) |
88 |
13 |
14.8% (3.8%) |
stream2 |
0.7 (0.1) |
1.8 (0.5) |
89 |
37 |
41.6% (5.2%) |
stream3 |
0.8 (0.1) |
1.4 (0.4) |
49 |
4 |
8.2% (3.9%) |
2.5 Photoinactivation of lab-cultured Enterococcus faecalis
Enterococcus faecalis (ATCC 19433) batch cultures were grown in Brain Heart Infusion broth (Fluka Analytical, Steinheim, Germany) at 37 °C for ∼20 hours. 1 mL of the batch culture was washed by centrifuging at 9600 × g for 10 minutes and resuspending the pellet in 1 mL PBS. The bacteria were washed in PBS two more times, centrifuging for 5 minutes between each wash, and then diluted into 50 mL PBS for photoinactivation experiments as described above.
2.6 Data analysis
Upon visual examination of concentration time series (C) from the various experiments, we observed that some inactivation curves had an apparent shoulder while others did not. Therefore, data were fit using a least-squares method to log-linear (eqn (1)) and shoulder log-linear (eqn (2)) models:47 |  | (1) |
| 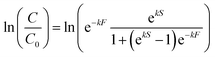 | (2) |
where ln(C/C0) is the ln-transformed relative concentration, C0 is the concentration measured at F = 0, k is the photoinactivation rate constant with units m2 kJUVB−1, F is fluence in kJUVB m−2 in the UVB range (280–320 nm), and S is the shoulder constant with units of kJUVB m−2 and was constrained to values >0 during the curve fitting. Fluence was corrected for light screening using the absorbance of reactor solutions in each experiment (see the ESI &Fig. S2†). Data points from biological replicates were combined prior to fitting eqn (1) and (2). The fit of the two model types, as measured by the residual standard error (RSE), was compared, and the model with the lowest RSE was chosen as the final model. F90% (UVB fluence required to achieve 90% inactivation) was calculated by solving eqn (1) or (2) for F using the fitted k and S parameters (if applicable) and setting (C/C0) = 0.1 using Microsoft Excel Solver. k values from either eqn (1) or (2) for bacteria sourced from WWTP, beach, or stream were averaged, respectively, to generate kWWTP, kbeach, and kstream. Pair-wise comparisons of k averaged for each sample-type were performed using a z-test at a confidence level of 95%.48 Photoinactivation rate constants with respect to fluence in the UVA & UVB range (280–400 nm) are also provided in the ESI.†
The fraction of pigmented isolates was calculated as the number of pigmented isolates divided by the total number of isolates for each sample or source type, and the standard error was calculated as
| 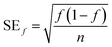 | (3) |
where
f is the fraction of pigmented isolates and
n is the total number of isolates. Pearson correlations between the fraction of pigmented isolates and
k or
F90% were calculated.
To compare the photoinactivation rate constants in this study to those in previous studies, we systematically reviewed the literature to identify studies that measured sunlight inactivation of enterococci, as well as studies that measured enterococci pigmentation and sunlight inactivation. Details of the systematic reviews are provided in the ESI.† We performed unit conversions and accounted for light screening where necessary to conform rate constants to the same units used in this study. One of the identified studies also includes a model using biological weighting functions to predict photoinactivation rates of wastewater-sourced enterococci;24 we therefore also used this model to compare to the wastewater photoinactivation rate constants observed in this study. All details for unit conversions, light screening calculations, and applying the biological weighting function model are described in the ESI.†
All data analyses described herein were performed in Microsoft Excel and R v3.4.0.
3. Results
At the time of sampling, water sources had diverse temperatures (range = 8.4 to 26.4 °C), salinities (0.1 to 32.7 ppt), dissolved oxygen concentrations (0.30 to 12.08 mg L−1), and turbidities (1.37 to 232.5 NTU). Enterococci concentrations in WWTP and surface water samples ranged from 2 × 103 to 3 × 104 CFU mL−1 (WWTP) and 0.5 to 8.8 CFU mL−1 (surface water), respectively (Table S2†). The fraction of pigmented enterococci isolates ranged from 0 to 42% across the samples (Table 1). The absorbance spectra of the environmental waters are provided in Fig. S3.†
Enterococci inactivation in the sunlit (Fig. 1) reactors were modeled using log-linear or shoulder log-linear equations. All inactivation was modeled with respect to fluence in the UVB range, taking into account light attenuation due to absorbance in the water column (described further in the ESI†). This was necessary as the absorbance of the solutions placed in the reactors varied among experiments (Fig. S4†). The method for calculating fluence requires an assumption that light attenuation due to absorbance is more important than that due to scattering. This assumption was valid for all samples except beach2. Sample beach2 likely violated this assumption due to the particularly low absorbance of the reactor solution for this sample (Fig. S4†).
Decay rate constants for dark controls (kd, Fig. S5†) for all but one experiment were not statistically different from zero (p > 0.05), suggesting that inactivation observed in sunlit reactors was due to sunlight exposure. Statistically significant decay occurred in the dark control reactor containing enterococci from a stream (stream3), but the effect size was small (kd ± SE = 0.04 ± 0.003 m2 kJUVB−1, p = 0.04, compared to k ± SE = 0.8 ± 0.1 m2 kJUVB−1 in the sunlit reactor), so no correction for dark decay was made.
Inactivation curves of uncultivated, indigenous enterococci from eleven of the thirteen waters were best fit using the shoulder log-linear model. The shoulder lengths (S) from these models ranged from 0.6 to 3.9 kJUVB m−2. The remaining two waters (beach1 and beach3) were best fit using the log-linear model. k values from the inactivation curves, considering k from both log-linear and shoulder log linear models together, varied over an order of magnitude, from 0.3 to 2.3 m2 kJUVB−1 (Table 1).
The inactivation of a laboratory-cultured, reference strain of E. faecalis was best fit with a shoulder log-linear model with a k value of 3.1 m2 kJUVB−1 (SE = 0.6), significantly larger than the k values measured for the indigenous, uncultured bacteria (p < 0.05, Z test) except for those from WWTP1 (k ± SE = 2.5 ± 0.2 m2 kJUVB−1) and pond1 (k ± SE = 2.2 ± 0.7 m2 kJUVB−1).
We tested the hypotheses that photoinactivation varied (1) among enterococci from diverse source types and (2) as a function of pigmentation. We excluded results from beach2 due to the relative importance of light scattering that yielded potentially inaccurate estimates of UVB fluence (see the ESI†). We excluded pond1 from our testing of the first hypothesis because n = 1 for this source type. Average kWWTP, kbeach, and kstream were statistically different from each other (Z test, p < 0.05, Fig. 3) with kWWTP > kstream > kbeach. There was also an association between the fraction of pigmented enterococci in the raw water and sample type (Chi-square test, p < 0.05, excluding pond sample type because npond = 1). To test hypothesis (2), we evaluated the correlation between pigmentation fraction and k or F90%. Including data from pond1, there was a significant, positive correlation between the fraction of pigmented enterococci in the water at the time it was collected and F90% for the indigenous, uncultured enterococci (Pearson correlation, R = 0.61, p < 0.05, n = 12) and a negative correlation between the fraction of pigmented enterococci and k (R = 0.51, p < 0.1, n = 12, curve fit: k = −2.5 × f + 1.6) (Fig. 2). Overall, 35%, 24%, and 11% of enterococci isolates were pigmented from beach, stream, and WWTP sources (Fig. 3).
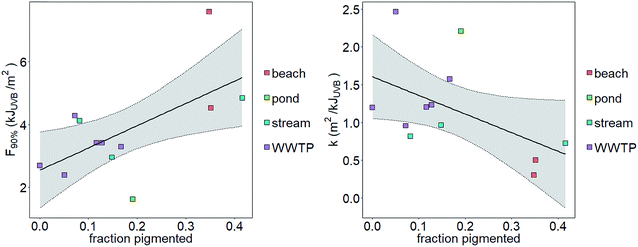 |
| Fig. 2 Correlation between the fraction of pigmented enterococci isolates and F90% (left) or k (right). Shaded region bounded by dotted lines is 95% confidence interval around a linear model fit. | |
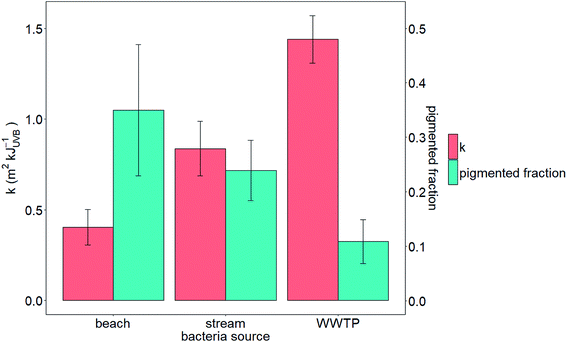 |
| Fig. 3 Average photoinactivation rate constants (k) of enterococci sourced from wastewater (n = 6), urban streams (n = 3), and beach water (n = 2), and fraction of pigmented enterococci isolated from wastewater (n = 231), urban streams (n = 226), and beach water (n = 60). Error bars represent the 95% confidence interval. | |
We also performed all data analyses described above after calculating rate constants that accounted for light screening of both UVA and UVB wavelengths (280–400 nm; see Table S3† for rate constants). Inclusion of the UVA wavelengths in the light screening calculation did not significantly alter any of the relationships presented above. As UVB photons are the most energetic UV photons incident on the Earth's surface, they are expected to exert the most influence on photoinactivation of bacteria when inactivation is dominated by endogenous processes. As such, we feel justified to choose the energy in the UVB as the basis for our rate constant calculations.31,42,49 Therefore, additional discussion of results will be centered on inactivation after adjusting for screening of UVB.
As experiments were carried out in the laboratory by adding indigenous bacteria to buffer (PBS), we did not explore associations between photoinactivation rates and raw water properties like temperature and salinity.
4. Discussion
4.1 Indigenous, uncultured enterococci inactivate at different rates than laboratory-cultured E. faecalis
In our evaluation of indigenous, uncultured enterococci photoinactivation from thirteen different sources, indigenous uncultured enterococci from eleven of the sources had smaller k values than a common lab-cultured reference strain E. faecalis. Thus, caution should be taken in applying photoinactivation rate constants obtained for a single lab-culture strain to indigenous, environmental enterococci.
One procedural difference in the experiments that used indigenous, environmental enterococci and lab-cultured enterococci is that the indigenous uncultured enterococci were stored prior to commencement of the photoinactivation experiments up to 48 hours while the lab-cultured E. faecalis were used immediately after overnight culturing. While we did not specifically test the effect of storage on photoinactivation of the decay of E. faecalis, previous work has shown that the growth rate and growth phase of E. faecalis minimally affect its photoinactivation kinetics under full spectrum simulated sunlight.50
Previous studies have calculated photoinactivation rate constants of lab-cultured E. faecalis that range between 0.4 and 2.0 m2 kJUVB−1,23,24,31,42 which are smaller than the value presented herein (k = 3.1 m2 kJUVB−1) but are also larger than photoinactivation rate constants from the majority of indigenous uncultured enterococci presented herein.
Waterborne indigenous enterococci likely represent a mixture of various Enterococcus species with potentially different decay characteristics. There are more than 30 species of Enterococcus.51 To our knowledge, the photoinactivation of each of these species has not been documented with the exception of lab-cultured E. casseliflavus (k = 0.2–0.6 m2 kJUVB−1) which is a pigmented species.31,32 The photoinactivation rate constants of the indigenous, uncultured enterococci photoinactivation rate constants in our study are similar to those reported previously for lab-cultured E. casseliflavus. This led us to suspect that pigmentation may be an important factor controlling photoinactivation kinetics of the uncultured indigenous enterococci.
The analysis presented herein assumes that correcting for light screening in the UVB portion of the spectrum adequately controls for differences between the experimental solutions, so that k values can be compared among the various experiments. Fig. S4† clearly shows that absorbance varies among the solutions, likely due to the co-concentration of colored material along with the indigenous bacteria. Inherent in this assumption is that exogenous indirect photoinactivation, or inactivation caused by reactive species generated through the interactions of photons and colored molecules or particles in the reactors, plays an insignificant role compared to endogenous photoinactivation. This assumption is probably valid given that evidence suggests that it is unusual for exogenous indirect photoinactivation to play an important role in the photoinactivation of enterococci.23 However, some work using treated wastewater effluent as a matrix has highlighted that the exogenous, indirect mechanism of photoinactivation can sometimes be important for enterococci.31 Interestingly, the pond1 sample was obtained from a pond filled with treated wastewater, and its k value was among the highest reported even though the community was highly pigmented, which may suggest that exogenous indirect mechanisms were at work and enhancing photoinactivation during the experiments.
4.2 The fraction of pigmented enterococci affects photoinactivation
Previous studies suggest that enterococcal pigments can influence photoinactivation.31,32,46,52,53 We observed an association between pigmentation and the environment from which the enterococci were isolated (WWTPs, streams and beaches). This could be due to differences in enterococci speciation or previous sunlight exposures between environments, as pigmentation is associated with sunlight exposure and certain enterococci species are more likely to exhibit pigmentation than others.32,54 Furthermore, we observed a negative association between pigmentation fraction and k providing support for a causal relationship between the two. The association suggested a linear relationship between k and f.
We investigated whether the linear relationship between k and f derived through or laboratory studies could predict k from other studies where both k and f were measured concurrently. There is only one study in the literature that reports both k and f for indigenous enterococci at a field site.16,32 Boehm et al. determined in situ enterococcal photoinactivation at a beach in southern California using a mechanistic model16 and found k = 0.8 m2 kJUVB−1 (see the ESI†). In another study at the same location and time, Maraccini et al.32 report that f varies as a function of the time of day with average f = 0.22. Assuming k = −2.5 × f + 1.6, k is predicted to be 1.1 m2 kJUVB−1, similar to the k previously reported for this beach using in situ measurements and empirical modeling.16 Further work to optimize enterococci decay modeling based on easily measurable community characteristics, such as pigmentation, is warranted.
A few previous studies have evaluated photoinactivation of indigenous, uncultured enterococci from wastewater and reported k as ranging from 0.08 to 0.6 m2 kJUVB−1.24,31 These k values are smaller than those calculated for indigenous wastewater enterococci published herein (1–2.5 m2 kJUVB−1). We applied a previously developed model using biological weighting functions to determine photoinactivation rates of wastewater-source enterococci; these models predict kWWTP = 0.09–0.1 m2 kJUVB−1,24 smaller than the k values we report. We hypothesize that differences in the fraction of pigmented enterococci in the wastewater-sourced enterococcal communities in our study and those published previously may explain some of the differences in reported and modeled decay rate constants.
4.3 A shoulder log-linear model was needed to describe inactivation of most indigenous enterococci
The simplest and most common method used for modeling the decay rates of pathogen indicators like enterococci is a log-linear model. However, studies observe deviations from log-linear kinetics.23,26,55 The shoulder log-linear model includes a shouldering behavior that is attributed to some protective component in the bacteria or water matrix that must be destroyed before inactivation is observed.47,56 For eleven of the thirteen indigenous enterococci communities evaluated, a shoulder log-linear model provided the best fit for the photoinactivation data, suggesting that such a model may be needed for modeling enterococci decay in sunlit waters. Shoulder log-linear models have been used in previous work on the decay of indicator bacteria and bacterial pathogens.21,27,57 Incorporating shoulder log-linear kinetics into predictive or mechanistic water quality models presents a challenge because it implies that bacterial decay is dependent on previous exposure duration, and often this is not a reasonable parameter to estimate since contamination sources are usually unknown.58 However, in specific instances where there are known sources of pollution, using a shoulder log-linear model may be feasible.
4.4 Practical implications
The use of water quality models to aid in pollution source identification or health risk assessment is becoming increasingly common.13,59,60 Such models require knowledge on bacterial inactivation and common practice is to use lab-cultured strains to parameterize this process. The variability of enterococci photoinactivation rates observed in this study and the influence of pigmentation point toward the importance of physiological characteristics and community heterogeneity on inactivation in environmental systems. Furthermore, the results call into question the appropriateness of using laboratory-cultured bacteria for estimating inactivation rates of indigenous, uncultured enterococci. A recent study on viral disinfection identified variability between environmental and laboratory strains as a key factor in determining a virus's susceptibility to inactivation.61
While homogeneous laboratory cultures are ideal for improving experimental reproducibility and evaluating specific decay processes, we recommend that future work should attempt to better address the differences between bacterial communities generated in laboratory culture versus those occurring in environmental reservoirs. Additionally, our work demonstrates that easy-to-measure characteristics like pigmentation significantly influence enterococci decay and that a shoulder log-linear model provided the best fit in most cases. These parameters should be included in future water quality modeling efforts in order to improve prediction of microbial pollutant decay.
5. Conclusions
• Photoinactivation rate constants of uncultured enterococci from wastewater and surface waters are different from those of lab-cultured E. faecalis.
• Enterococcal pigmentation is associated with decreasing photoinactivation rate constants.
• Photoinactivation of indigenous uncultured enterococci often displays a shouldering behavior before decay.
Conflicts of interest
There are no conflicts to declare.
Acknowledgements
This work was funded by National Science Foundation (NSF) grant CBET-1334359, and JSM was supported by a NSF Graduate Research Fellowship (DGE-114747). We would like to thank the staff at the wastewater treatment plants for their assistance with sample collection; Bryan Olney for contributing to preliminary experiments and method development; Andrew Gutierrez, Katy Graham, Hilary Starks, and Ben Kranner for their assistance with surface water sample collection; and Alex Szczuka for performing NPOC measurements.
References
- V. J. Cabelli, A. P. Dufour, L. J. McCabe and M. A. Levin, Swimming-Associated Gastroenteritis and Water Quality, Am. J. Epidemiol., 1982, 115(4), 606–616 CrossRef CAS.
- B. F. Arnold, K. C. Schiff, A. Ercumen, J. Benjamin-Chung, J. A. Steele, J. F. Griffith, S. J. Steinberg, P. Smith, C. D. McGee and R. Wilson,
et al., Acute Illness among Surfers after Exposure to Seawater in Dry-and Wet-Weather Conditions, Am. J. Epidemiol., 2017, 186(7), 866–875 CrossRef PubMed.
- A. Pruss, Review of Epidemiological Studies on Health Effects from Exposure to Recreational Water, Int. J. Epidemiol., 1998, 27(1), 1–9 CrossRef CAS PubMed.
-
V. J. Cabelli, Health Effects Criteria for Marine Recreational Waters, U.S. Environmental Protection Agency, Research Triangle Park, 1983 Search PubMed.
- T. J. Wade, N. Pai, J. N. S. Eisenberg and J. M. Colford, Do U.S. Environmental Protection Agency Water Quality Guidelines for Recreational Waters Prevent Gastrointestinal Illness? A Systematic Review and Meta-Analysis, Environ. Health Perspect., 2003, 111(8), 1102–1109 CrossRef PubMed.
- S. DeFlorio-Barker, C. Wing, R. M. Jones and S. Dorevitch, Estimate of Incidence and Cost of Recreational Waterborne Illness on United States Surface Waters, Environ. Health: Global Access Sci. Source, 2018, 17(1), 1–10 CrossRef.
- C. Brendel and M. Soupir, Relating Watershed Characteristics to Elevated Stream Escherichia Coli Levels in Agriculturally Dominated Landscapes: An Iowa Case Study, Water, 2017, 9(3), 154 CrossRef.
- D. Aranda, J. V. Lopez, H. M. Solo-Gabriele and J. M. Fleisher, Using Probabilities of Enterococci Exceedance and Logistic Regression to Evaluate Long Term Weekly Beach Monitoring Data, J. Water Health, 2016, 14(1), 81–89 CrossRef PubMed.
- K. D. Goodwin, A. Schriewer, A. Jirik, K. Curtis and A. Crumpacker, Consideration of Natural Sources in a Bacteria TMDL - Lines of Evidence, Including Beach Microbial Source Tracking, Environ. Sci. Technol., 2017, 51(14), 7775–7784 CrossRef CAS PubMed.
- A. B. Boehm, N. J. Ashbolt, J. M. Colford, L. E. Dunbar, L. E. Fleming, M. A. Gold, J. A. Hansel, P. R. Hunter, A. M. Ichida and C. D. McGee,
et al., A Sea Change Ahead for Recreational Water Quality Criteria, J. Water Health, 2009, 7(1), 9–20 CrossRef PubMed.
- K. H. Cho, S. M. Cha, J. H. Kang, S. W. Lee, Y. Park, J. W. Kim and J. H. Kim, Meteorological Effects on the Levels of Fecal Indicator Bacteria in an Urban Stream: A Modeling Approach, Water Res., 2010, 44(7), 2189–2202 CrossRef CAS PubMed.
- L. Liu, M. S. Phanikumar, S. L. Molloy, R. L. Whitman, D. A. Shively, M. B. Nevers, D. J. Schwab and J. B. Rose, Modeling the Transport and Inactivation of E. coli and Enterococci in the near-Shore Region of Lake Michigan, Environ. Sci. Technol., 2006, 40(16), 5022–5028 CrossRef CAS PubMed.
- D. Hou, S. J. M. Rabinovici and A. B. Boehm, Enterococci Predictions from Partial Least Squares Regression Models in Conjunction with a Single-Sample Standard Improve the Efficacy of Beach Management Advisories, Environ. Sci. Technol., 2006, 40(6), 1737–1743 CrossRef CAS PubMed.
- E. J. Viau, K. D. Goodwin, K. M. Yamahara, B. A. Layton, L. M. Sassoubre, S. L. Burns, H. I. Tong, S. H. C. Wong, Y. Lu and A. B. Boehm, Bacterial Pathogens in Hawaiian Coastal Streams-Associations with Fecal Indicators, Land Cover, and Water Quality, Water Res., 2011, 45(11), 3279–3290 CrossRef CAS PubMed.
- R. J. Davies-Colley, A. M. Donnison, D. J. Speed, C. M. Ross and J. W. Nagels, Inactivation of Faecal Indicator Micro-Organisms in Waste Stabilisation Ponds: Interactions of Environmental Factors with Sunlight, Water Res., 1999, 33(5), 1220–1230 CrossRef CAS.
- A. B. Boehm, K. M. Yamahara, D. C. Love, B. M. Peterson, K. Mcneill and K. L. Nelson, Covariation and Photoinactivation of Traditional and Novel Indicator Organisms and Human Viruses at a Sewage-Impacted Marine Beach, Environ. Sci. Technol., 2009, 43(21), 8046–8052 CrossRef CAS.
- S. R. Corsi, M. a. Borchardt, R. B. Carvin, T. R. Burch, S. K. Spencer, M. a. Lutz, C. M. McDermott, K. M. Busse, G. T. Kleinheinz and X. Feng,
et al., Human and Bovine Viruses and Bacteria at Three Great Lakes Beaches: Environmental Variable Associations and Health Risk, Environ. Sci. Technol., 2015, 50(2), 987–995 CrossRef PubMed.
- T. L. Russell, L. M. Sassoubre, D. Wang and A. B. Boehm, A Coupled Modeling and Molecular Biology Approach to Microbial Source Tracking at Cowell Beach, Santa Cruz, CA, United States, Environ. Sci. Technol., 2013, 47(18), 10231–10239 CAS.
- A. B. Boehm, K. E. Graham and W. Jennings, Can We Swim yet? Systematic Review, Meta-Analysis, and Risk Assessment of Aging Sewage in Surface Waters, Environ. Sci. Technol., 2018, 52(17), 9634–9645 CrossRef CAS.
- M. Berney, H.-U. Weilenmann, A. Simonetti and T. Egli, Efficacy of Solar Disinfection of Escherichia coli, Shigella flexneri, Salmonella typhimurium and Vibrio cholerae, J. Appl. Microbiol., 2006, 101(4), 828–836 CrossRef CAS PubMed.
- A. B. Boehm, C. Soetjipto and D. Wang, Solar Inactivation of Four Salmonella Serovars in Fresh and Marine Waters, J. Water Health, 2012, 10(4), 504–510 CrossRef.
- L. M. Sassoubre, K. L. Nelson and A. B. Boehm, Mechanisms for Photoinactivation of Enterococcus faecalis in Seawater, Appl. Environ. Microbiol., 2012, 78(21), 7776–7785 CrossRef CAS PubMed.
- P. A. Maraccini, J. Wenk and A. B. Boehm, Photoinactivation of Eight Health-Relevant Bacterial Species: Determining the Importance of the Exogenous Indirect Mechanism, Environ. Sci. Technol., 2016, 50(10), 5050–5059 CrossRef CAS PubMed.
- A. I. Silverman and K. L. Nelson, Modeling the Endogenous Sunlight Inactivation Rates of Laboratory Strain and Wastewater E. coli and Enterococci Using Biological Weighting Functions, Environ. Sci. Technol., 2016, 50(22), 12292–12301 CrossRef CAS PubMed.
- M. Boyle, C. Sichel, P. Fernández-Ibáñez, G. B. Arias-Quiroz, M. Iriarte-Puña, A. Mercado, E. Ubomba-Jaswa and K. G. McGuigan, Bactericidal Effect of Solar Water Disinfection under Real Sunlight Conditions, Appl. Environ. Microbiol., 2008, 74(10), 2997–3001 CrossRef CAS PubMed.
- J. S. McClary, L. M. Sassoubre and A. B. Boehm,
Staphylococcus aureus Strain Newman Photoinactivation and Cellular Response to Sunlight Exposure, Appl. Environ. Microbiol., 2017, 83(17), 1–14 CrossRef PubMed.
- N. Al-Jassim, D. Mantilla-Calderon, T. Wang and P.-Y. Hong, Inactivation and Gene Expression of a Virulent Wastewater Escherichia coli Strain and the Nonvirulent Commensal Escherichia coli DSM1103 Strain Upon Solar Irradiation, Environ. Sci. Technol., 2017, 51(7), 3649–3659 CrossRef CAS.
- L. W. Sinton, C. H. Hall, P. A. Lynch and R. J. Davies-Colley, Sunlight Inactivation of Fecal Indicator Bacteria and Bacteriophages from Waste Stabilization Pond Effluent in Fresh and Saline Waters, Appl. Environ. Microbiol., 2002, 68(3), 1122–1131 CrossRef CAS.
- R. L. Whitman, M. B. Nevers, G. C. Korinek and M. N. Byappanahalli, Solar and Temporal Effects on Escherichia Coli Concentration at a Lake Michigan Swimming Beach, Appl. Environ. Microbiol., 2004, 70(7), 4276–4285 CrossRef CAS PubMed.
- R. S. Fujioka, H. H. Hashimoto, E. B. Siwak and R. H. F. Young, Effect of Sunlight on Survival of Indicator Bacteria in Seawater, Appl. Environ. Microbiol., 1981, 41(3), 690–696 CAS.
- M. T. Nguyen, J. T. Jasper, A. B. Boehm and K. L. Nelson, Sunlight Inactivation of Fecal Indicator Bacteria in Open-Water Unit Process Treatment Wetlands: Modeling Endogenous and Exogenous Inactivation Rates, Water Res., 2015, 83, 282–292 CrossRef CAS.
- P. A. Maraccini, D. M. Ferguson and A. B. Boehm, Diurnal Variation in Enterococcus Species Composition in Polluted Ocean Water and a Potential Role for the Enterococcal Carotenoid in Protection against Photoinactivation, Appl. Environ. Microbiol., 2012, 78(2), 305–310 CrossRef CAS PubMed.
- T. Hagi, M. Kobayashi and M. Nomura, Aerobic Condition Increases Carotenoid Production Associated with Oxidative Stress Tolerance in Enterococcus gilvus, FEMS Microbiol. Lett., 2014, 350(2), 223–230 CrossRef CAS PubMed.
- T. P. Curtis, D. D. Mara and S. A. Silva, Influence of PH, Oxygen, and Humic Substances on Ability of Sunlight to Damage Fecal Coliforms in Waste Stabilization Pond Water, Appl. Environ. Microbiol., 1992, 58(4), 1335–1343 CAS.
-
K. I. Metselaar, T. Abee, M. H. Zwietering and M. W. D. Besten, Modeling and Validation of the Ecological Behavior of Wild-Type Listeria monocytogenes and Stress-Resistant Variants, Appl. Environ. Microbiol., 2016, 82(17), 5389–5401 Search PubMed.
-
A. M. Sousa, I. Machado and M. O. Pereira, Phenotypic Switching: An Opportunity to Bacteria Thrive, Sci. against Microb. Pathog. Commun. Curr. Res. Technol. Adv., 2011, pp. 252–262 Search PubMed.
- S. Probst-Rud, K. McNeill and M. Ackermann, Thiouridine Residues in TRNAs Are Responsible for a Synergistic Effect of UVA and UVB Light in Photoinactivation of Escherichia coli, Environ. Microbiol., 2016, 19(2), 434–442 CrossRef PubMed.
- M. B. Fisher, M. Iriarte and K. L. Nelson, Solar Water Disinfection (SODIS) of Escherichia coli, Enterococcus Spp., and MS2 Coliphage: Effects of Additives and Alternative Container Materials, Water Res., 2012, 46(6), 1745–1754 CrossRef CAS PubMed.
- K. I. Brown and A. B. Boehm, Comparative Decay of Catellicoccus marimmalium and Enterococci in Beach Sand and Seawater, Water Res., 2015, 83, 377–384 CrossRef CAS PubMed.
- L. W. Sinton, R. J. Davies-Colley and R. G. Bell, Inactivation of Enterococci and Fecal Coliforms from Sewage and Meatworks Effluents in Seawater Chambers, Appl. Environ. Microbiol., 1994, 60(6), 2040–2048 CAS.
- K. L. Anderson, J. E. Whitlock, J. Valerie and V. J. Harwood, Persistence and Differential Survival of Fecal Indicator Bacteria in Subtropical Waters and Sediments, Appl. Environ. Microbiol., 2005, 71(6), 3041–3048 CrossRef CAS PubMed.
- P. A. Maraccini, M. C. M. Mattioli, L. M. Sassoubre, Y. Cao, J. F. Griffith, J. S. Ervin, L. C. Van De Werfhorst and A. B. Boehm, Solar Inactivation of Enterococci and Escherichia coli in Natural Waters: Effect of Water Absorbance and Depth, Environ. Sci. Technol., 2016, 50(10), 5068–5076 CrossRef CAS PubMed.
-
S. Wuertz, D. Wang, K. Zamani and F. Bombardelli, An Analysis of Water Circulation in Pillar Point Harbor, Half Moon Bay, California, Based on the Dye Distribution Study of September 27, 2008, 2011 Search PubMed.
- L. M. Sassoubre, S. P. Walters, T. L. Russell and A. B. Boehm, Sources and Fate of Salmonella and Fecal Indicator Bacteria in an Urban Creek, J. Environ. Monit., 2011, 13(8), 2206–2212 RSC.
-
United States Environmental Protection Agency, Method 1600: Enterococci in Water by Membrane Filtration Using Membrane-Enterococcus Indoxyl-β-D-Glucoside Agar (MEI), 2002 Search PubMed.
- R. R. Facklam and M. D. Collins, Identification of Enterococcus Species Isolated from Human Infections by a Conventional Test Scheme, J. Clin. Microbiol., 1989, 27(4), 731–734 CAS.
- A. H. Geeraerd, V. P. Valdramidis and J. F. Van Impe, GInaFiT, a Freeware Tool to Assess Non-Log-Linear Microbial Survivor Curves, Int. J. Food Microbiol., 2005, 102(1), 95–105 CrossRef CAS.
- L. E. Brooks and K. G. Field, Bayesian Meta-Analysis to Synthesize Decay Rate Constant Estimates for Common Fecal Indicator Bacteria, Water Res., 2016, 104, 262–271 CrossRef CAS.
- K. L. Nelson, A. B. Boehm, R. J. Davies-Colley, M. C. Dodd, T. Kohn, K. G. Linden, Y. Liu, P. A. Maraccini, K. McNeill and W. A. Mitch,
et al., Sunlight-Mediated Inactivation of Health-Relevant Microorganisms in Water: A Review of Mechanisms and Modeling Approaches, Environ. Sci.: Processes Impacts, 2018, 20(8), 1089–1122 RSC.
- P. A. Maraccini, D. Wang, J. S. McClary and A. B. Boehm, Growth-Dependent Photoinactivation Kinetics of Enterococcus faecalis, J. Appl. Microbiol., 2015, 118(5), 1226–1237 CrossRef CAS.
- W. Köhler, The Present State of Species within the Genera Streptococcus and Enterococcus, Int. J. Med. Microbiol., 2007, 297(3), 133–150 CrossRef PubMed.
- D. M. Ferguson, D. F. Moore, M. A. Getrich and M. H. Zhowandai, Enumeration and Speciation of Enterococci Found in Marine and Intertidal Sediments and Coastal Water in Southern California, J. Appl. Microbiol., 2005, 99(3), 598–608 CrossRef CAS PubMed.
- F. D. Halstead, J. E. Thwaite, R. Burt, T. R. Laws, M. Raguse, R. Moeller, M. A. Webber and B. A. Oppenheim, The Antibacterial Activity of Blue Light against Nosocomial Wound Pathogens Growing Planktonically and as Mature Biofilms, Appl. Environ. Microbiol., 2016, 82, 4006–4016 CrossRef CAS PubMed.
- J. O. Mundt, Occurrence of Enterococci on Plants in a Wild Environment, Appl. Microbiol., 1963, 11(2), 141–144 CAS.
- A. F. Brouwer, M. C. Eisenberg, J. V. Remais, P. A. Collender, R. Meza and J. N. S. Eisenberg, Modeling Biphasic Environmental Decay of Pathogens and Implications for Risk Analysis, Environ. Sci. Technol., 2016, 3 Search PubMed.
-
D. A. A. Mossel, J. E. L. Corry, C. B. Struijk and R. M. Baird, Essentials of Microbiology of Foods, John Wiley & Sons, 1995 Search PubMed.
- L. W. Sinton, R. K. Finlay and P. A. Lynch, Sunlight Inactivation of Fecal Bacteriophages and Bacteria in Sewage- Polluted Seawater, Appl. Environ. Microbiol., 1999, 65(8), 3605–3613 CAS.
-
M. B. Nevers and A. B. Boehm, Modeling Fate and Transport of Fecal Bacteria in Surface Water, in Fecal Bacteria, ed. M. J. Sadowsky and R. L. Whitman, ASM Press, 2010, pp. 165–188 Search PubMed.
- A. B. Boehm, N. S. Ismail, L. M. Sassoubre and E. A. Andruszkiewicz, Oceans in Peril: Grand Challenges in Applied Water Quality Research for the 21st Century, Environ. Eng. Sci., 2015, 34(1) DOI:10.1089/ees.2015.0252.
- A. Safaie, A. Wendzel, Z. Ge, M. B. Nevers, R. L. Whitman, S. R. Corsi and M. S. Phanikumar, Comparative Evaluation of Statistical and Mechanistic Models of Escherichia coli at Beaches in Southern Lake Michigan, Environ. Sci. Technol., 2016 DOI:50(5), 2442-2449.
- S. Meister, M. E. Verbyla, M. Klinger and T. Kohn, Variability in Disinfection Resistance between Currently Circulating Enterovirus B Serotypes and Strains, Environ. Sci. Technol., 2018, 52(6), 3696–3705 CrossRef CAS.
Footnotes |
† Electronic supplementary information (ESI) available: Methods for calculation of light scattering and depth-averaged light intensity; explanation for exclusion of sample pond1 from pigmentation analyses; and additional results including complete physicochemical and microbial measurements of samples, irradiation spectra, absorbance spectra of reactor solutions, dark control data, and absorbance spectra of raw water samples. See DOI: 10.1039/c8em00443a |
‡ Present address: School of Freshwater Sciences, University of Wisconsin-Milwaukee, Milwaukee, WI, USA. |
|
This journal is © The Royal Society of Chemistry 2019 |
Click here to see how this site uses Cookies. View our privacy policy here.