Humic acid removal by gas–liquid interface discharge plasma: performance, mechanism and comparison to ozonation†
Received
31st July 2018
, Accepted 15th November 2018
First published on 16th November 2018
Abstract
The removal of natural organic matter (NOM) is a major objective for drinking water treatment. In this study, a novel advanced oxidation process (AOP) based on plasma in gas–liquid interface discharge was investigated for removing humic acid (HA), and ozonation treatment effect was evaluated as a comparison. Several indexes including UV254, dissolved organic carbon (DOC), specific UV absorbance (SUVA), and excitation-emission matrix (EEM) fluorescence spectra were employed to characterize HA transformation. The results showed that typical species including ozone, hydrogen peroxide and HO˙ were produced in the plasma process, which made better removal efficiency than ozonation. Especially, UV254 was removed effectively by 96.5% and 90.3% within 15 min by plasma and ozonation, while DOC was 49.2% and 41.1%, respectively. HO˙ radicals were majorly responsible for the degradation of HA in the plasma process, which were mainly generated via reaction of in situ produced ozone and H2O2 during the discharge process. UV/vis absorbance and EEM results further showed that HA with the large molecular weight fraction was decomposed to small molecular weight fraction. This study indicates that plasma technology has a significant application prospect as a replacement for ozone in drinking water industry.
Water impact
Natural organic matter (NOM) removal is a major objective for drinking water treatment. An advanced oxidation process (AOP) based on plasma in gas–liquid interface discharge was evaluated for humic acid removal. Much better performance was obtained compared to ozonation. The OH˙ radicals generated by reaction of in situ produced ozone and H2O2 during discharge process were mainly responsible for the removal.
|
1. Introduction
The removal of natural organic matter (NOM) is a major objective for drinking water treatment. NOM usually contributes to undesirable color, taste and odors in water sources, acts as a carrier for metals and various harmful organic chemicals, and even causes bacterial re-growth in drinking water distribution system.1,2 In addition, NOM in the source water can decrease the efficiency of disinfectants and lead to the formation of toxic disinfection by-products (DBPs).3 Besides THMs and HAAs, more than 600 DBPs have been identified in drinking water to date.4–6 Moreover, NOM has been attributed to the fouling of microfiltration, ultrafiltration, and nanofiltration (NF) membranes.7,8 Humic acids (HA) are the major fractions of NOM in water and therefore the removal of HA has been extensively investigated.9,10
Coagulation and activated carbon adsorption are commonly adopted for the removal of HA in drinking water treatment;11 however, it is frequently found to be limited with low molecular weight fractions. Many of the studies have been related to ozonation or advanced oxidation processes (AOPs) because of their high oxidation potentials. Ozone (O3) can react fast with organic pollutants containing amino groups, activated aromatic systems, double bonds, or reduced sulphur moieties.12 Comparably, the AOPs are attractive because of the production of hydroxyl radicals (HO˙). Different AOP technologies have been studied in water treatment, e.g., UV/peroxide, ozone/peroxide, UV/ozone, photocatalysis, etc.13–15
Electrical discharge plasma, an emerging AOP, has gained increasing interest in water treatment because of its high removal efficiency. It leads to various physical and chemical effects, such as primary formation of oxidizing species: radicals (hydroxyl radical, oxygen radical and hydrogen radical) and molecules (H2O2, O3, etc.), shockwave, UV and electrohydraulic cavitation.16 This makes it effective even for some typical contaminants that cannot to be easily destroyed by conventional AOPs. Additionally, electrical discharge plasma has many advantages such as high degradation efficiency, no chemical addition and no secondary pollution.17 Compared with some other technologies,18 it might be a relatively efficient and safe option for the removal of micro pollutants from drinking water. For example, some risks would be brought by residual Al3+ during coagulation;19 large amount of disinfection by-products would be generated using ozone or other oxidation treatments.20 Some studies have recently addressed plasma applications in drinking water treatment for the removal of micro pollutants.21–23 However, for natural water, the presence of NOM not only affects the removal effect of micro-pollutants, but also has the potential impact of producing byproducts of disinfection. Therefore, it is essential to understand the characteristic transformation of HA during the oxidation process. Wang and Panorel et al. have evaluated the HA removal potential by plasma.24,25 However, there is still a lack of understanding on the oxidation mechanism of plasma. It is known that ozone could be produced by pulsed discharge and plays a major role in the removal of contaminants. In order to further understand the mechanism of plasma, a comparable study between ozonation and the plasma process is necessary.
The objectives of this study were to assess the HA removal characteristics by gas–liquid discharge plasma and investigate the basic mechanism of the plasma treatment. As comparison, ozonation treatment effect was also evaluated. In this study, a pulsed discharge reactor in water droplet spray was applied. The main active components produced by pulsed discharge in both oxygen atmosphere and argon atmosphere were analyzed, then UV254 and DOC removal efficiency by plasma and O3 treatment were compared.
2. Materials and methods
2.1. Chemicals
The commercial HA (technical grade), t-butanol (99.5%) and the catalase for quenching H2O2 were purchased from Wako Pure Chemical Industries Ltd. (Japan). Analytical grade sodium hydroxide (NaOH) (8 mol L−1), hydrochloric acid (HCl) (1 mol L−1), KH2PO4 (99.5%) and Na2HPO4 (99%) were also purchased from Wako Pure Chemical Industries Ltd. (Japan).
2.2. Synthetic water preparation
HA stock solution was first prepared by mixing 0.50 g of HA in 500 mL of pure water over a period of at least 3 h of continuous stirring, during which pH was adjusted at 9.5–10.0 by using 8 M NaOH. After this, the pH of HA stock solution was adjusted to 7.0 with 1 M HCl. The solution was first filtered through 0.45 μm glass fiber membrane filters to remove undissolved HA and stored in the dark at 4 °C. The synthetic waters were prepared with about 3.0 mg L−1 DOC (spiking desired HA stock solutions), which is the usual level reflecting some typical surface source waters. To maintain the pH with no significant variations during the reaction experiments, the synthetic waters were buffered using phosphate solutions (0.03 mM KH2PO4 and 0.105 mM Na2HPO4, pH = 7.3–7.5).
2.3. Experimental procedure
2.3.1. Plasma treatment.
The schematic diagram of the plasma experimental apparatus is shown in Fig. 1(a). The experimental apparatus consists of a pulsed power generator, reactor chamber, water tank, magnetic pump, circulating pipe, oxygen gas bomb, argon gas bomb, and gas mass flow controller (MFC). The reactor chamber has a similar configuration to the one developed by Minamitani et al.,26 and consists of wire-to-cylinder coaxial electrode (inner diameter of 35 mm and 300 mm length), and a spray shower that is set at the top of the reactor chamber. The wire electrode is formed of titanium wire (diameter 0.5 mm) for applying pulse voltages, and the cylindrical electrode is a stainless steel one to be grounded. The water tank (3 L) is provided with a pump for circulation of treated water, which is also made of stainless steel. Oxygen gas (99.9% pure) or argon gas (99.999% pure) can be switched or mixed as carrier gases in the reactor chamber.
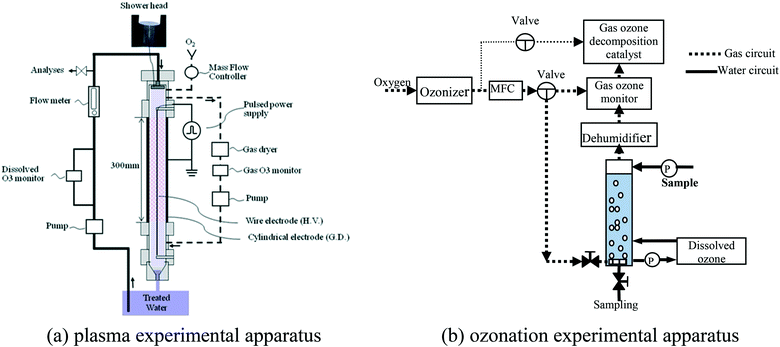 |
| Fig. 1 Schematic diagram of the plasma experimental apparatus and ozonation experimental apparatus. | |
Positive pulsed high voltage was applied to the wire electrode to generate pulsed discharge between the electrodes. The power supply (MPC3010S-50SP, Suematsu Electronics) used a magnetic pulse compressor (MPC) to generate short pulsed voltage with a width of about 100 ns. The waveforms of the pulse voltage and discharging current were measured by a high voltage probe (P6015, Tektronix) and a current monitor (6959, Pearson), respectively, and monitored with an oscilloscope (DPO5104, Tektronix).
In the discharge plasma treatment, the discharge conditions were set as follows: pulse discharge energy was 12.0 mJ per pulse; repetition frequency of the applied voltage was 500 pps; and carrier gas flow rate of oxygen or argon was set at 0.2 L min−1 at standard conditions controlled by a MFC (SEC-400, STEC). At this discharge condition, a gas ozone concentration of about 20 g Nm−3 was produced while using oxygen carrier gas, which was used as the applied condition for ozonation treatment comparison. While conducting the plasma experiment, 2.8 L of the synthetic water was sprayed into the reactor chamber with a PTFE magnetic pump (IFP-611, AS ONE) at a circulation flow rate of 1.8 L min−1, and a dissolved ozone monitor was placed along with the pump. Samples were taken at given time intervals and the residual ozone was liberated by at least 0.5 min air purging. The gas in the reactor was circulated with a PTFE bellows pump (BA-106F, Iwaki) at a flow rate of 0.5 L min−1, and a gaseous ozone monitor was placed in the flow path.
In addition, the plasma characteristics and the treatment efficiency after reducing the gaseous ozone concentration were investigated in this study. This was achieved by introducing an ozone decomposing catalyst (SCA-131, Sakai Chemical Industry) in the gas circulation path (Fig. S1†), in which the gaseous ozone concentration could be reduced to the desired level by adjusting the gas circulation flow rate.
2.3.2. Ozonation treatment.
The schematic diagram of the ozonation experimental apparatus is shown in Fig. 1(b). Ozone was generated from oxygen using an ozone generator (OP-75C, Mitsubishi Electric Corporation, Japan). The ozonation reactor (inner diameter 50 mm, height 1520 mm) was made of transparent polyvinyl chloride (PVC) material. Before entering into the air, the used ozone from the reactor (off gas) was decomposed by an ozone decomposition catalyst. The gaseous ozone and dissolved ozone concentration could be detected on line by the ozone monitor (Ebara Jitsugyo, Japan).
For ozonation experiments, the reactor operated in semi-batch mode with a continuous inflow of an ozone mixture. Before the start of the experiment, 2.8 L of the synthetic water was transferred into the reactor via a peristaltic pump. To compare with the plasma treatment, the ozone flow rate was set to 0.2 L min−1 with the help of a mass flow controller, and the inlet gaseous ozone concentration was set to 20 g Nm−3. Prior to each run, the produced inlet gaseous ozone was stabilized for 5–10 min and then its concentration was measured. Afterwards, ozone was introduced to the treated water via a porous glass diffusion tube, and then the off gas was measured for the remaining period. At regular time intervals, samples were taken for different analyses.
2.4. Analytical methods
The dissolved organic carbon (DOC) of the solution was determined on a TOC analyzer (Shimadzu, TOC-V CSH, Japan) by the 680 °C combustion catalytic oxidation method.
The UV/vis absorbance of the solutions in the range λ = 200 nm to 700 nm was measured in a UV/vis spectrophotometer (UV-2550, Shimadzu, Japan), and the UV absorbance at 254 nm (UV254) was also recorded. EEM fluorescence spectra were measured on a fluorescence spectrophotometer (FP-6300, JASCO, Japan). EEM spectra were recorded in the excitation wavelength range of λex = 240–700 nm with a step width of 5 nm and in the emission wavelength range of λem = 250–700 nm with a step width of 5 nm.
The pH and conductivity during the plasma treatment process were measured using LAQUA twin compact pH meter (HORIBA B-711, Japan) and conductivity meter (HORIBA B-771, Japan), respectively.
Gaseous ozone concentrations were determined by online measurements with a Model EG-600 and EG-620 UV ozone monitor (Ebara Jitsugyo, Japan) in ozonation and plasma treatment system, respectively. Dissolved ozone concentrations were determined by online measurements with a Model EL-600 and EL-620 ozone dissolved monitor (Ebara Jitsugyo, Japan) in ozonation and plasma system, respectively, which were calibrated by subtracting the UV absorbance at 254 nm produced by the corresponding HA concentration during treatment. The concentrations of hydrogen peroxide produced during the discharge process were measured using a hydrogen peroxide meter (SUPER ORITECTOR MODEL 5, CENTRAL KAGAKU CORP.).
3. Results and discussions
3.1. Ozone and H2O2 production during discharge treatment
Typical waveforms of the discharge voltage and discharge current using pure oxygen and argon as carrier gases are shown in Fig. S2 (a) and (b),† respectively, which were applied at the same pulse discharge energy of 12.0 mJ. The measured peak pulse voltage and peak discharge current in the oxygen gas were 26.0 kV and 19.6 A, respectively, and were 13.3 kV and 15.3 A in the argon gas, respectively. This was because compared to oxygen, much lower breakdown voltage was needed to discharge for argon.
Fig. 2(a) shows time variations of hydrogen peroxide (H2O2) concentrations during discharge treatment with the above discharge conditions. Hydrogen peroxide dissolved in the treatment solution is mainly formed by the recombination of HO˙ radicals in the gas phase generated by the plasma. In the oxygen conditions, hydrogen peroxide concentration gradually increased with treatment time and almost reached the maximum of 0.8 mg L−1 at about 10 min, then gradually decreased. The produced H2O2 further reacted with ozone, inducing HO˙ production in the solutions, which further decomposed the pollutants. While in argon conditions, hydrogen peroxide concentration almost increased linearly with treatment time. Recombination of HO˙ radicals produced H2O2, while different from oxygen conditions, no O-based species (ozone etc.) were formed in this system. Moreover, H2O2 did not significantly react with most organic compounds. Thus, residual H2O2 in the solution increased continuously as discharge time increased. Fig. 2(b) shows the ozone produced during oxygen discharge treatment. As indicated, a stable gaseous ozone concentration of 18.5–19.0 g Nm−3 is produced, which plays a very important role for the plasma treatment because ozone can further diffuse from the gas into the liquid and react directly or indirectly with the pollutant molecules. The dissolved ozone concentration was almost zero for the first two minutes, increased to a stable concentration of about 0.8 mg L−1, and then decreased slowly after 20 min. Due to the existence of H2O2, ozone can transform into HO˙ radicals, consequently expressing a high oxidation capacity as the AOP. Correspondingly, while reducing the gas ozone concentration to about half of the initial level by circulating the gas to pass an O3 decomposing catalyst, the maximum dissolved ozone concentration was also reduced to a much lower level (about 0.4 mg L−1, Fig. 2(c)). Conversely, the residual H2O2 concentrations were higher than the oxygen discharge plasma conditions without catalyst.
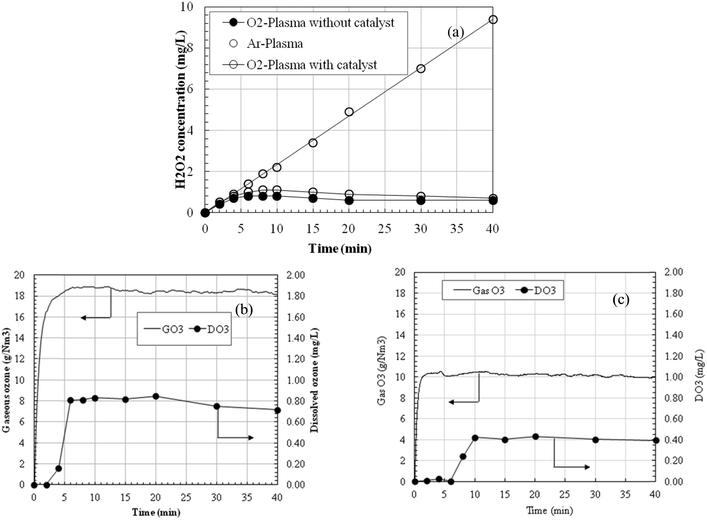 |
| Fig. 2 Time variation of (a) hydrogen peroxide and ozone concentration during the plasma treatment without (b) and with (c) ozone decomposing catalyst. | |
3.2. UV254 and DOC removal by plasma and O3 treatment
The variation of the UV254 and DOC during the O3 and plasma processes is shown in Fig. 3. For plasma treatment with Ar carrier gas, almost no UV254 or DOC was removed. This meant that for this plasma reactor, although HO˙ radicals and hydrogen peroxide were produced, direct reaction of HO˙ with HA in the water droplets was minor due to its short diffusion length (a few μm) from the gas–water interface,27 and H2O2 was not active with most compounds. This further implied that almost no interfacial reaction occurred in the reactor. When using oxygen as carrier gas, ozone can be formed during the discharge process. As shown in Fig. 3, for ozonation and plasma treatment, almost the highest UV254 removal efficiency was obtained with comparable UV254 removal rates of 90.3% and 96.5% at 15 min, respectively, which is similar to previous findings that ozone can remove UV254 effectively. The removal efficiency of DOC was 49.2% and 41.4% for plasma and ozonation at 15 min, respectively. However, different from that DOC removal was limited even with prolonged ozonation treatment time; DOC removal could be further improved to 69.1% at plasma treatment of 40 min. Plasma treatment was more efficient than ozonation for DOC and UV254 reduction, indicating that HO˙ radicals generated via reaction of dissolved ozone with H2O2 could degrade the HA effectively. While decreasing the gaseous and dissolved ozone accordingly in plasma treatment using ozone decomposing catalyst (Fig. 2), the removal of UV254 and DOC was also decreased to some extent before 10 min because high transfer efficiency of ozone could be achieved in high gas ozone conditions, leading to high reaction efficiency. But after 10 min, almost no difference could be observed. As seen in Fig. 2, a stable dissolved ozone concentration was observed after 10 min, where ozone was enough to react with H2O2 for similar HO˙ production in both conditions.
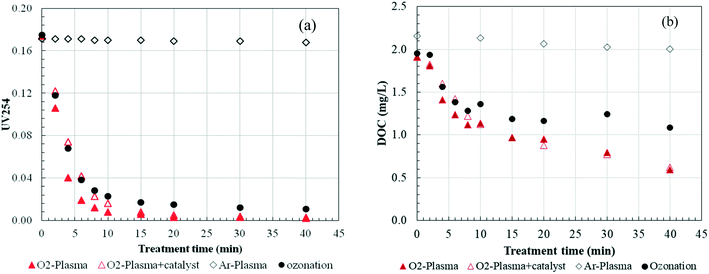 |
| Fig. 3 UV254 (a) and DOC (b) variation of the humic acid during ozonation and plasma process. | |
Furthermore, as clearly seen in Fig. 3, the removal of UV254 was preferred to the removal of DOC both for ozonation and plasma. This indicated an incomplete mineralization of the organics after the breakup of large aromatic and conjugated structures. Similar results were also reported by Huang et al.28 who observed that a fraction of the DOC could not be removed completely, implying the presence of some refractory compounds, either present in the beginning or generated during the treatment process.
3.3. UV/vis spectroscopic analysis
Fig. S3† further gives UV/vis absorption spectra of HA during ozonation and plasma treatment process. The absorbance spectrum of raw water contained a shoulder with a maximum at around 265 nm. With increasing treatment time during ozonation and plasma treatment, the UV/vis absorbance was observed to generally decrease as a result of the partial oxidation of chromophoric moieties of HA, which decreased much faster for plasma treatment. These observations were in agreement with previous findings that ozone especially attacked some moieties absorbing in the 250–280 nm UV regions.29,30 This preferential reaction was also the case in this study, which resulted in a total disappearance of the shoulder after treatment of ozone and plasma, which also have high concentration of ozone. The UV/vis absorbance showed no significant change for ozonation treatment after 15 min, indicating that complete mineralization could not be achieved. Moreover, for plasma treatment, although in the initial phase (10 min before), UV/vis absorbance decreased slowly after decreasing the ozone concentration with ozone decomposing catalyst. The same performance could still be achieved, which showed much lower absorbance compared to ozonation, indicating that further mineralization could be achieved due to radical reaction. Below the wavelength of 230 nm, different from the ozonation process, much higher absorbance was found and increased with time. This might be due to the absorbance of residual H2O2 and some intermediates produced during oxidation, which could still have absorbance at lower UV regions.
Specific UV absorbance parameters SUVA254, SUVA280, SUVA365, and SCOA436 were calculated as indicated in Table 1. The specific UV absorbance is defined as the UV absorbance per milligram of DOC (SUVA, L mg−1 m−1), and the specific color absorbance is defined as Color436 per milligram of DOC (SCOA436, L mg−1 m−1).31 For NOM materials, the parameters SUVA254 and SUVA280 are usually associated with the UV-absorbing aromatic structures and double bonds.3 It is reported that high SUVA254 values indicate that the organic matter is composed predominantly of hydrophobic compounds with high apparent molecular weights.32 In addition, SUVA365 has been demonstrated to increase with increasing molecular weight, while SCOA436 represents functional groups lending yellow to brown color to the samples.31,33 This parameter of SCOA436 is also used to estimate the content of quinines.32 As shown, a considerable decrease can be observed in all SUVA values during ozonation and plasma degradation of HA, implying a reduction in aromatic halogen attack sites, a decrease in the molecular size, and the elimination of quinones. Of course, much higher reduction was achieved using plasma treatment, even 100% removal for SUVA365 after 20 min and for SCOA436 only after 8 min treatment, but there was almost no further removal after 15 min of ozonation treatment. This further indicated that much effective radical reactions had occurred during the plasma process, although radical reactions could also participate during the ozonation process.
Table 1 Specific absorbance parameters of the remaining HA after ozonation and plasma treatment
Treatment time (min) |
Plasma treatment (L mg−1 m−1) |
Plasma treatment with catalyst (L mg−1 m−1) |
Ozonation treatment (L mg−1 m−1) |
SUVA (254) |
SUVA (280) |
SUVA (365) |
SCOA (436) |
SUVA (254) |
SUVA (280) |
SUVA (365) |
SCOA (436) |
SUVA (254) |
SUVA (280) |
SUVA (365) |
SCOA (436) |
0 |
9.11 |
8.17 |
3.98 |
2.10 |
9.09 |
8.15 |
3.92 |
2.09 |
8.96 |
8.04 |
3.94 |
2.15 |
2 |
5.87 |
4.87 |
2.32 |
1.22 |
6.69 |
5.65 |
2.74 |
1.48 |
6.10 |
5.17 |
2.53 |
1.40 |
4 |
2.84 |
2.13 |
0.85 |
0.43 |
4.62 |
3.74 |
1.68 |
0.87 |
4.36 |
3.52 |
1.67 |
0.90 |
6 |
1.54 |
0.97 |
0.32 |
0.08 |
2.95 |
2.25 |
0.91 |
0.42 |
2.75 |
2.02 |
0.87 |
0.43 |
8 |
1.07 |
0.63 |
0.18 |
0.00 |
1.89 |
1.31 |
0.41 |
0.16 |
2.19 |
1.48 |
0.55 |
0.23 |
10 |
0.70 |
0.44 |
0.09 |
0.00 |
1.42 |
0.89 |
0.27 |
0.09 |
1.69 |
1.10 |
0.37 |
0.15 |
15 |
0.62 |
0.41 |
0.21 |
0.00 |
0.83 |
0.52 |
0.10 |
0.00 |
1.43 |
0.84 |
0.25 |
0.08 |
20 |
0.31 |
0.21 |
0.00 |
0.00 |
0.57 |
0.34 |
0.11 |
0.00 |
1.29 |
0.77 |
0.26 |
0.09 |
30 |
0.38 |
0.25 |
0.00 |
0.00 |
0.52 |
0.26 |
0.00 |
0.00 |
0.97 |
0.56 |
0.16 |
0.08 |
40 |
0.34 |
0.34 |
0.00 |
0.00 |
0.48 |
0.32 |
0.00 |
0.00 |
1.01 |
0.55 |
0.18 |
0.09 |
Removal % |
96.28 |
95.85 |
100.00 |
100.00 |
94.68 |
96.05 |
100.00 |
100.00 |
88.71 |
93.13 |
95.33 |
95.72 |
3.4. EEM fluorescence spectroscopic analysis
Fig. 4 shows the detailed EEM contour plots of the HA solution before and after ozonation/plasma treatment at 4 and 10 min. Based on previous results,10,34 excitation and emission boundaries were operationally defined into five regions. The regions are described as follows: (I) aromatic protein, (II) aromatic protein II, (III) fulvicacid-like, (IV) soluble microbial byproduct-like, and (V) humic acid-like. Additionally, f450/500 corresponded to the ratio of emission intensity at 450 nm over 500 nm at 370 nm excitation and lower f450/500 may refer to the compounds with more aromatic structures. The f450/500 for raw water solution was 0.99. As shown in Fig. 4, the peak of raw HA solution appeared in Region V. Additionally, this HA solution almost did not exhibit a fluorescence signal near the λex/λem = 225–237 nm/309–321 nm region, which is associated with tyrosine-like compounds; but a weak signal near the λex/λem = 275 nm/340 nm and λex/λem = 225–237 nm/340–380 nm regions, which are associated with tryptophan-like compounds. Tyrosine- and tryptophan-like compounds are labile organics under microbial activity and are usually predominant in wastewater.35 These data further suggested that this HA solution mainly contained humic acid-like substances, that is, many aromatic sites.
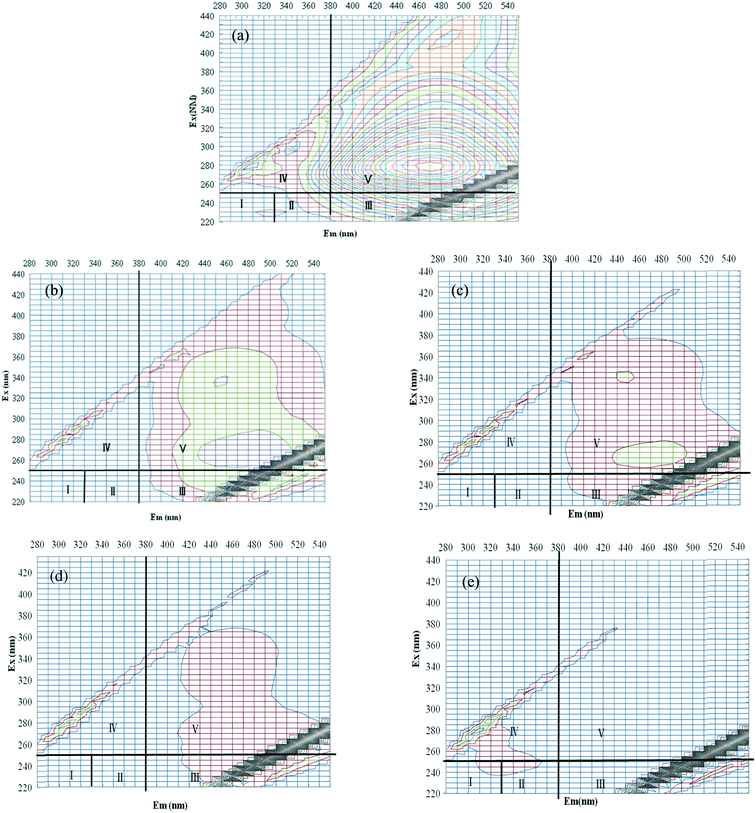 |
| Fig. 4 EEM fluorescence diagrams for the remaining HA after ozonation and plasma treatment (peak A: FA-like, peak C: HA-like.): (a) raw water, (b) ozonation treatment-4 min, (c) ozonation treatment-10 min, (d) plasma treatment-4 min, (e) plasma treatment-10 min. | |
The oxidation treatment led to a continuous reduction of the fluorescence intensity and changes in the shape of the EEM plots of the peak. For ozonation treatment, the fluorescence intensity of peak at 280/470 (Ex/Em (nm)) in Region V decreased from 26.5 (a.u.) to 3.3 (4 min) and 2.0 (10 min). While treating within plasma, the peak in Region V almost disappeared even at 4 min. It was easy to understand because the breakup of aromatic structures was easily achieved by ozone treatment. Specifically, the blue shift of the peak could be seen along the excitation and emission axis while treating with ozone. A blue shift is associated with a decomposition of condensed aromatic moieties and the break-up of large molecules into smaller fragments, such as a reduction in the degree of the π-electron systems. A blue shift is also associated with a decrease in the number of aromatic rings, fewer conjugated bonds in a chain structure, the conversion of a linear system to a nonlinear systems or/and elimination of particular functional groups including carbonyl, hydroxyl, and amine groups.36 The result suggested that HA with the large molecular weight fraction was decomposed to small molecular weight fraction during the ozonation process. As an advanced oxidation process, similar with ozone or O3/H2O2, O3 and HO˙ would be mainly responsible for the removal of the pollutants during the plasma treatment process.37 Some lower molecular weight by-products such as aldehydes and carboxylic acids would be produced while treating HA.38,39 Thus, it would be better to combine with biological activated carbon (BAC) to further eliminate the oxidation intermediates while applying plasma in drinking water industry. Moreover, for the source water bearing bromide, the bromate formation and control measures should be considered.40 Further studies should be conducted in the future.
The transformations caused by the two different processes could be further clarified using the ASI. The ASI was calculated as the ratio of the slope of the absorbance between 254 and 272 nm and the slope between 220 and 230 nm, which was found to be strongly correlated with the specific UV absorbance (SUVA) and the trihalomethane (THM) formation potential of NOM originating from different natural waters.41 The ASIs were calculated for the oxidation of HA during ozonation and plasma treatment in this study. As shown in Fig. 5, a prolonged treatment resulted in an overall decrease of the ASI. However, during the initial treatment phase, the ASI tended to increase. Possibly, some unknown transformations of HA might have occurred which led to this result, and similar results were also reported in previous studies.42 It might be possible that during the early stage of ozonation some new products were formed that absorbed at 254 nm. While we investigated the slope calculations according to the equation, the increase in ASIs was due to the increase of the numerator, which supported this hypothesis. Most likely, transformation products that absorbed at lower wavelengths were formed. Nöthe et al.43 also hypothesized that highly conjugated material (e.g. aromatic compounds) strongly absorbing at higher wavelengths (e.g. 436 nm) can be transformed into compounds that still exhibit absorption at lower wavelengths (e.g. 254 nm) after ozonation. When we decreased the dissolved ozone using catalyst, by which the dissolved ozone concentration was almost zero and the reaction process was mainly driven by HO˙, no significant transformations happened, thus the increase of ASI did not appear. After 4 min, dissolved ozone was still present similar to the plasma process without using catalyst, thus almost the same ASI variations occurred. Of course, this hypothesis needed to be further confirmed.
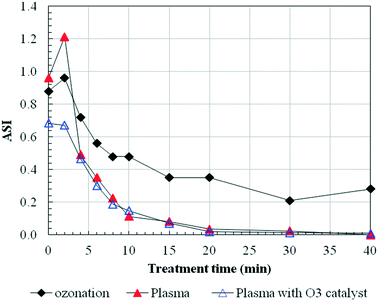 |
| Fig. 5 Evolution of the absorbance slope index during ozone and plasma (values obtained from UV/vis spectra). | |
Conclusions
In this experiment, removal of HA by plasma and ozone treatment processes and the basic mechanism of the plasma treatment were studied. The experimental results demonstrated that typical species including ozone, hydrogen peroxide and HO˙ were produced by discharging in water droplet spray. The plasma process in oxygen atmosphere had higher ability for DOC and UV254 reduction than ozonation, indicating that HO˙ radicals generated via the reaction of dissolved ozone with H2O2 could degrade the HA effectively. UV/vis absorbance and EEM fluorescence spectra showed that HA with large molecular weight fraction was decomposed to small molecular weight fraction during plasma with oxygen carrier gas and ozonation process.
Conflicts of interest
There are no conflicts to declare.
Acknowledgements
One of the authors (Jianwei Yu) gratefully acknowledges the support from the JAPAN TRUST International Research Cooperation Project of the New Energy and Industrial Technology Development Organization (NEDO) for his research stay in Japan. This study was supported by Funds for the National Natural Science Foundation of China (No. 51778602), the Major Science and Technology Program for Water Pollution Control and Treatment (No. 2015ZX07406001, No. 2017ZX07207004). This study was exclusively conducted at the Research Center for Eco-Environmental Sciences, Chinese Academy of Sciences and the Advanced Technology R&D Center, Mitsubishi Electric Corporation.
References
- L. Joseph, J. R. V. Flora, Y. G. Park, M. Badawy, H. Saleh and Y. Yoon, Removal of natural organic matter from potential drinking water sources by combined coagulation and adsorption using carbon nanomaterials, Sep. Purif. Technol., 2012, 95, 64–72 CrossRef CAS.
- C. Lu and C. Chu, Effects of acetic acid on the regrowth of heterotrophic bacteria in the drinking water distribution system, World J. Microbiol. Biotechnol., 2005, 21, 989–998 CrossRef CAS.
- S. R. Sarathy, M. The impact of UV/H2O2 advanced oxidation on molecular size distribution of chromophoric natural organic matter, Mohseni, Environ. Sci. Technol., 2007, 41, 8315–8320 CrossRef CAS.
- C. Postigo, C. I. Cojocariu, S. D. Richardson, P. J. Silcock and D. Barcelo, Characterization of iodinated disinfection by-products in chlorinated and chloraminated waters using Orbitrap based gas chromatography-mass spectrometry, Anal. Bioanal. Chem., 2016, 408, 3401–3411 CrossRef CAS PubMed.
- S. D. Richardson and C. Postigo, A new technique helps to uncover unknown peptides and disinfection by-products in water, J. Environ. Sci., 2016, 42, 6–8 CrossRef PubMed.
- C. Postigo, S. D. Richardson and D. Barcelo, Formation of iodo-trihalomethanes, iodo-haloacetic acids, and haloacetaldehydes during chlorination and chloramination of iodine containing waters in laboratory controlled reactions, J. Environ. Sci., 2017, 58, 127–134 CrossRef.
- Y. Yoon, G. Amy and J. Cho, N. Her, Effects of retained natural organic matter (NOM) on NOM rejection and membrane flux decline with nanofiltration and ultrafiltration, Desalination, 2015, 173, 209–221 CrossRef.
- N. Her, G. Amy, J. Chung, J. Yoon and Y. Yoon, Characterizing dissolved organic matter and evaluating associated nanofiltration membrane fouling, Chemosphere, 2008, 70, 495 CrossRef CAS PubMed.
- G. Zhao, X. Lu, Y. Zhou and Q. Gu, Simultaneous humic acid removal and bromate control by O3 and UV/O3 processes, Chem. Eng. J., 2013, 232, 74–80 CrossRef CAS.
- A. Li, X. Zhao, H. Liu and J. Qu, Characteristic transformation of humic acid during photoelectrocatalysis process and its subsequent disinfection byproduct formation potential, Water Res., 2011, 45, 6131–6140 CrossRef CAS PubMed.
- T. Bond, E. H. Goslan, S. A. Parsons and B. Jefferson, Disinfection by-product formation of natural organic matter surrogates and treatment by coagulation, MIEX® and nanofiltration, Water Res., 2010, 44, 1645–1653 CrossRef CAS PubMed.
- G. U. Von, Ozonation of drinking water: part I. Oxidation kinetics and product formation, Water Res., 2003, 37, 1443–1467 CrossRef.
- R. Rosal, A. Rodríguez, J. A. Perdigón-Melón, A. Petre and E. García-Calvo, Oxidation of dissolved organic matter in the effluent of a sewage treatment plant using ozone combined with hydrogen peroxide (O3/H2O2), Chem. Eng. J., 2009, 149, 311–318 CrossRef CAS.
- M. M. Huber, S. Canonica, A. Gunyoung Park and U. V. Gunten, Oxidation of pharmaceuticals during ozonation and advanced oxidation processes, Environ. Sci. Technol., 2003, 37, 1016 CrossRef CAS PubMed.
- M. J. Benotti, B. D. Stanford, E. C. Wert and S. A. Snyder, Evaluation of a photocatalytic reactor membrane pilot system for the removal of pharmaceuticals and endocrine disrupting compounds from water, Water Res., 2009, 43, 1513–1522 CrossRef CAS PubMed.
- B. Jiang, J. T. Zheng, S. Qiu, M. B. Wu, Q. H. Zhang, Z. F. Yan and Q. Z. Xue, Review on electrical discharge plasma technology for wastewater remediation, Chem. Eng. J., 2014, 236, 348–368 CrossRef CAS.
- Y. Li, R. J. Yi, C. W. Yi, B. Y. Zhou and H. J. Wang, Research on the degradation mechanism of pyridine in drinking water by dielectric barrier discharge, J. Environ. Sci., 2017, 53, 238–247 CrossRef PubMed.
- Q. Zhang, G. Qu, T. Wang, C. Li, H. Qiang, Q. Sun, D. Liang and S. Hu, Humic acid removal
from micro-polluted source water in the presence of inorganic salts in a gas-phase surface discharge plasma system, Sep. Purif. Technol., 2017, 187, 334–342 CrossRef CAS.
- Y. Wang, Q. Wang, B. Y. Gao, Q. Yue and Y. Zhao, The disinfection by-products precursors removal efficiency and the subsequent effects on chlorine decay for humic acid synthetic water treated by coagulation process and coagulation–ultrafiltration process, Chem. Eng. J., 2012, s193–194, 59–67 CrossRef.
- M. C. Wei, K. S. Wang, T. E. Hsiao, I. C. Lin, H. J. Wu, Y. L. Wu, P. H. Liu and S. H. Chang, Effects of UV irradiation on humic acid removal by ozonation, Fenton and Fe0/air treatment: THMFP and biotoxicity evaluation, J. Hazard. Mater., 2011, 195, 324–331 CrossRef CAS PubMed.
- M. Magureanu, D. Piroi, N. B. Mandache, V. David, A. Medvedovici, C. Bradu and V. I. Parvulescu, Degradation of antibiotics in water by non-thermal plasma treatment, Water Res., 2011, 45, 3407–3416 CrossRef CAS PubMed.
- J. O. Jo, D. K. Sang, H. J. Lee and Y. S. Mok, Decomposition of taste-and-odor compounds produced by cyanobacteria algae using atmospheric pressure plasma created inside a porous hydrophobic ceramic tube, Chem. Eng. J., 2014, 247, 291–301 CrossRef CAS.
- D. Gerrity, B. D. Stanford, R. A. Trenholm and S. A, An evaluation of a pilot-scale nonthermal plasma advanced oxidation process for trace organic compound degradation, Snyder, Water Res., 2010, 44, 493–504 CrossRef CAS PubMed.
- T. C. Wang, G. Z. Qu, J. Y. Ren, Q. H. Yan, Q. H. Sun, D. L. Liang and S. B. Hu, Evaluation of the potentials of humic acid removal in water by gas phase surface discharge plasma, Water Res., 2016, 89, 28–38 CrossRef CAS PubMed.
- I. Panorel, I. Kornev, H. Hatakka and S. Preis, Pulsed corona discharge for degradation of aqueous humic substances, Water Sci. Technol.: Water Supply, 2011, 11, 238–245 CAS.
- Y. Minamitani, S. Shoji, Y. Ohba and Y. Higashiyama, Decomposition of dye in water solution by pulsed power discharge in a water droplet spray, IEEE Trans. Plasma Sci., 2008, 36, 2586–2591 CAS.
- P. J. Bruggeman, M. J. Kushner, B. R. Locke and J. G. E. Gardeniers, Plasma-liquid interactions: a review and roadmap, Plasma Sources Sci. Technol., 2016, 25, 2–45 CrossRef.
- X. Huang, M. Leal and Q. Li, Degradation of natural organic matter by TiO2 photocatalytic oxidation and its effect on fouling of low-pressure membranes, Water Res., 2008, 42, 1142–1150 CrossRef CAS PubMed.
- V. Nanaboina and G. V. Korshin, Evolution of absorbance spectra of ozonated wastewater and its relationship with the degradation of trace-Level organic species, Environ. Sci. Technol., 2010, 44, 6130 CrossRef CAS PubMed.
- S. Liu, M. Lim, R. Fabris, C. W. K. Chow, M. Drikas, G. Korshin and R. Amal, Multi-wavelength spectroscopic and chromatography study on the photocatalytic oxidation of natural organic matter, Water Res., 2010, 44, 2525–2532 CrossRef CAS PubMed.
- S. Valencia, J. M. Marín, G. Restrepo and F. H. Frimmel, Application of excitation–emission fluorescence matrices and UV/Vis absorption to monitoring the photocatalytic degradation of commercial humic acid, Sci. Total Environ., 2013, 442, 207–214 CrossRef CAS PubMed.
- C. S. Uyguner and M. Bekbolet, Evaluation of humic acid photocatalytic degradation by UV–vis and fluorescence spectroscopy, Catal. Today, 2005, 101, 267–274 CrossRef CAS.
- J. Peuravuori and K. Pihlaja, Preliminary study of lake dissolved organic matter in light of nanoscale supramolecular assembly, Environ. Sci. Technol., 2004, 38, 5958 CrossRef CAS.
- W. Chen, P. Westerhoff, J. A. Leenheer and K. Booksh, Fluorescence excitation-emission matrix regional integration to quantify spectra for dissolved organic matter, Environ. Sci. Technol., 2003, 37, 5701–5710 CrossRef CAS.
- R. K. Henderson, A. Baker, K. R. Murphy, A. Hambly, R. M. Stuetz and S. J. Khan, Fluorescence as a potential monitoring tool for recycled water systems: a review, Water Res., 2009, 43, 863–881 CrossRef CAS.
- P. G. Coble, Mar. Characterization of marine and terrestrial DOM in seawater using excitation-emission matrix spectroscopy, Chem, 1996, 51, 325–346 CAS.
- K. H. Hama Aziz, H. Miessner, S. Mueller, D. Kalass, D. Moeller, I. Khorshid and M. A. M. Rashid, Degradation of pharmaceutical diclofenac and ibuprofen in aqueous solution, a direct comparison of ozonation, photocatalysis, and non-thermal plasma, Chem. Eng. J., 2017, 313, 1033–1041 CrossRef CAS.
- C. Sarangapani, P. Lu, P. Behan, P. Bourke and P. J. Cullen, Humic acid and trihalomethane breakdown with potential by-product formations for atmospheric air plasma water treatment, J. Ind. Eng. Chem., 2018, 59, 350–361 CrossRef CAS.
- X. Zhong, C. Cui and S. Yu, Identification of oxidation intermediates in humic acid oxidation, Ozone: Sci. Eng., 2017, 40, 93–104 CrossRef.
- N. Fridman and O. Lahav, Formation and minimization of bromate ions within non-thermal-plasma advanced oxidation, Desalination, 2011, 280, 273–280 CrossRef CAS.
- G. Korshin, C. W. Chow, R. Fabris and M. Drikas, Absorbance spectroscopy-based examination of effects of coagulation on the reactivity of fractions of natural organic matter with varying apparent molecular weights, Water Res., 2009, 43, 1541–1548 CrossRef CAS PubMed.
- W. T. M. Audenaert, D. Vandierendonck, S. W. H. V. Hulle and I. Nopens, Comparison of ozone and HO induced conversion of effluent organic matter (EfOM) using ozonation and UV/H2O2 treatment, Water Res., 2013, 47, 2387 CrossRef CAS PubMed.
- T. Nöthe, H. Fahlenkamp and C. V. Sonntag, Ozonation of wastewater: rate of ozone consumption and hydroxyl radical yield, Environ. Sci. Technol., 2009, 43, 5990 CrossRef.
Footnote |
† Electronic supplementary information (ESI) available. See DOI: 10.1039/c8ew00520f |
|
This journal is © The Royal Society of Chemistry 2019 |
Click here to see how this site uses Cookies. View our privacy policy here.