Aerobic microbial electrochemical technology based on the coexistence and interactions of aerobes and exoelectrogens for synergistic pollutant removal from wastewater†
Received
6th August 2018
, Accepted 22nd October 2018
First published on 23rd October 2018
Abstract
Microbial electrochemical technologies (METs), like microbial fuel cells, usually involve an anoxic bioanode. These technologies face considerable challenges during practical wastewater treatment due to the relatively low performance in terms of COD and pollutant removal as well as power output. In this study, we present a new concept of aerobic microbial electrochemical technology (A-MET) based on the coexistence and synergistic interactions of exoelectrogens and aerobes under aerobic conditions. It was realized by using the aerobic rotating electrode bioreactor (AREBR) concept to include/integrate microbial electrochemical reactions into the traditional rotating biological contractor (RBC) technology. Electrically conductive materials with electrocatalytic activity for oxygen reduction reaction (ORR) served as biofilm supports in the AREBRs instead of the non-conductive materials (e.g., polymer) in the traditional RBC. The AREBR exhibited multifunctional behavior that included aerobic and electrode-dependent respiration for organic carbon oxidation, electrode-dependent denitrification and ORR. Microbial community analysis revealed the dominance of the genus Neomegalonema as a potential biofilm producer and of the genus Arcobacter as an exoelectrogen within the biofilms of the AREBRs. The Neomegalonema spp. biofilm may have served as a protective layer and blocked the diffusion of oxygen by aerobic respiration of i.e. acetate. In situ ORR activity most likely consumed the electrons and sustained the respiration of Arcobacter. Higher ORR electrocatalytic activity of the biofilm support was found to be associated with the enhanced growth of Arcobacter in the AREBR. Thus, the A-MET concept allowed combining the merits of both aerobes and exoelectrogens at the same solid–liquid interface under aerobic conditions for efficient and synergistic pollutants removal from wastewater.
Water impact
Emerging microbial electrochemical technologies (MET), such as microbial fuel cells, usually involve an anoxic bioanode for growth of exoelectrogens, thus facing the challenge for its integration into the traditional aerobic wastewater treatment system. Here, we present a new concept of aerobic MET (A-MET), which allows combining the merits of both aerobes (high efficiency COD removal through aerobic respiration) and exoelectrogens (multifunctional bioelectrocatalytic activity of electrode respiration and bioelectrochemical denitrification) for efficient and synergistic pollutant removal from wastewater under aerobic conditions. It could thus offer an easy and promising way for the integration of novel METs into existing wastewater treatment facilities.
|
1. Introduction
Microbial electrochemical technologies (METs) utilize electrochemically active bacteria (also referred to as exoelectrogens, anodophilic bacteria, anode-respiring bacteria and electricigens)1–3 to oxidize organic matter, and to recover available energy and resources according to different cathodic reactions.4,5 These technologies can be applied in wastewater treatment (WWT) to recover the compounds or energy contained in different types of wastewaters.6–8 For instance, in microbial fuel cells (MFCs), the organic matter (commonly represented in terms of chemical oxygen demand, COD) in wastewater is used as fuel and removed at the anode by bioelectrocatalytic oxidation, and the nitrate, sulfate and metal ions serve as the electron acceptors and are removed at the cathode through reduction reactions.5,9,10
Though fundamental theory and technology has been advanced in the past decade,11,12 MFCs still face challenges for practical application in WWT. In one aspect, MFCs show a comparatively low rate of COD and pollutant removal and recover electric power at a low level. In another aspect, MFC usually involves an anoxic bioanode and thus faces the challenge to be combined with the traditional aerobic WWT system. The exoelectrogenic biofilms enriched from wastewater and grown on the MFC anode, such as those dominated by Geobacter species are anaerobic and require anoxic conditions to ensure a high bioelectrocatalytic activity. Although these biofilms are reportedly capable of withstanding low levels of dissolved oxygen and even possess the ability to use O2 as an electron acceptor,13,14 longer exposures to O2 inhibit their bioelectrocatalytic activity,15 and eventually greatly limit their electron generation ability.16 Furthermore, conventional MFCs consist of two compartments for the anode and cathode reactions, separated by a separator necessary to maintain different working conditions. This design makes its integration into WWT plants more complex.
In order to overcome the design and operational condition related disadvantages of MFC technology for WWT, we developed and tested a new concept of aerobic microbial electrochemical technology (A-MET) in this study. This concept is realized by using an aerobic rotating electrode bioreactor (AREBR), which is based on the design of the reported rotating biological contactor (RBC)17 but used conductive materials with electrocatalytic activity for oxygen reduction reaction (ORR) as biofilm supports instead of non-conductive materials (e.g., polymer) (Fig. 1, left panel). The AREBRs were firstly started under aerobic conditions using a set potential to accumulate exoelectrogens and then operated without potential control under aerobic conditions. We report for the first time that exoelectrogens (a) could be accumulated in the presence of oxygen along with aerobes and (b) sustained by the in situ ORR catalyzed by the conductive support with intrinsic ORR electrocatalytic activity under aerobic conditions in the AREBR design. The key aspect of the proposed A-MET concept is the integration of microbial electrochemical reactions into traditional aerobic biological wastewater treatment technologies, e.g., RBC. The synergistic interactions of both aerobes and exoelectrogens were demonstrated at an electrode, which was operated continuously under aerobic conditions in the presence of different electron acceptors. We propose that the aerobes ensure high efficiency of COD removal through aerobic respiration (AR) and the exoelectrogens possess multifunctional bioelectrocatalytic activity, such as electrode respiration (ER) and bioelectrochemical denitrification (BE-DN) in the AREBR (Fig. 1 right panel/zoom in section).
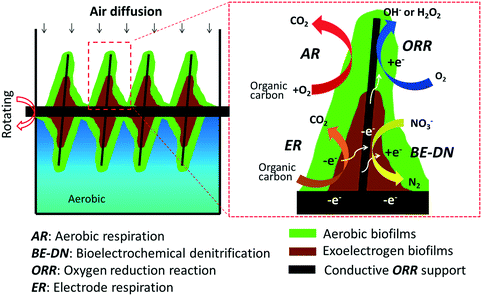 |
| Fig. 1 Schematic diagrams of a cross-sectional view of an aerobic rotating electrode bioreactor and the possible biological and bioelectrochemical processes, showing the concept of the aerobic microbial electrochemical technology. | |
2. Materials and methods
2.1 Materials
Graphite fiber brushes (GBs) with a size of 26 mm in diameter and 42 mm in length were made by fixing graphite fibers (TORAY) on a twisted titanium wire and had a projected area of approximately 10.9 cm2 (calculated by multiplying the diameter and the length). The twisted titanium wire had a diameter of about 2 mm. All chemicals including ammonium persulfate, aniline and phytic acid [50% (w/w) in water] were purchased from Sigma Aldrich. N,P co-doped carbon was coated onto the GB to form a GB/NPC electrode with higher ORR electrocatalytic activity following the method reported by Chen et al. (2018),18 and as described in the ESI.† The experiments were conducted with both GB and GB/NPC electrodes to check the efficacy and applicability of the reported phenomenon with both unmodified and modified electrodes.
2.2 Wastewater and inoculum source
The wastewater taken from the influent entrance of the WWTP (Wolfsburg-Hattorf, Wolfsburg, Germany) was used as both basal medium and inoculum in this study. All the wastewater used in this study was taken at one time, packaged in plastic bottles [500 mL, pre-sterilized by 70% ethanol (v/v)], and stored at −20 °C to ensure its constant composition. The wastewater was unfrozen and warmed up to about 20 °C before use. The initial COD of the wastewater was 155 mg L−1. It was measured with a COD meter (CADAS 100, Hach Lange) using tap water as a blank control. Before COD measurements, the wastewater was filtered to remove the microorganisms and solids using a microfiltration membrane with a pore size of 0.2 μm.
2.3 Experimental setup
The GB and GB/NPC were arranged horizontally at the water surface, connected to motors (Sol Expert G298-2, 5–75 U min−1) and served as the working electrodes in bioreactors (Plexiglass, volume of 150 mL). Without specification, the referred aerobic condition was natural air-diffusion in open air, and all the experiments were conducted at a temperature of 20 ± 2 °C. The rotation speed of the AREBRs was 20 rpm. The GB and GB/NPC electrodes, always connected as the working electrode, were half-submerged in the wastewater. Real wastewater with additional 20 mM acetate (total COD around 1400 mg L−1) was used as the growth medium and was refreshed/replenished every 24 hours during the potentiostat-controlled electrochemical accumulation or growth of the exoelectrogen process, while real wastewater with 10 mM acetate (total COD around 740 ± 85 mg L−1) was used during open circuit operation of the reactors.
2.4 Start-up and electrochemical tests of the AREBRs
The start-up of the AREBRs was conducted by potentiostat control to accumulate exoelectrogens. The accumulation or growth of exoelectrogens was achieved by using a three-electrode electrochemical system controlled by a potentiostat (MPEG, Biologic) under aerobic conditions with natural air-diffusion, as shown in Fig. S1A.† An Ag/AgCl electrode (saturated KCl, +0.198 V vs. standard hydrogen electrode) was used as a reference electrode and a graphite plate with a size of 4 × 5 cm2 was used as the counter electrode. All the potential values reported in this study refer to the Ag/AgCl (sat. KCl) electrode. A constant potential of +0.2 V was applied to the working electrodes during the potential controlled accumulation of exoelectrogens. Cyclic voltammograms (CVs) were recorded within a potential range of +0.4 to −0.5 V at a scan rate of 0.5 mV s−1. CVs were conducted at the blank electrodes and during the turnover and non-turnover states with biofilm electrodes. Current densities are normalized to the cross-sectional area of the GB (length × diameter, 42 mm × 26 mm). Average values are calculated from single cycles of three independent replicates. To investigate the different (bio)electrochemical reactions occurring at the AREBRs, chronoamperometry (CA) was performed by applying different constant potentials at the working electrodes, such as −0.2 V for ORR, −0.2 and +0.2 for ER of the exoelectrogens and −0.3 V for BE-DN.
Once stable maximum current densities were achieved at the GB and GB/NPC electrode supports, stable exoelectrogen biofilms were successfully selected and accumulated onto the GB and GB/NPC supports and the corresponding rotating electrode bioreactors had been started up successfully.
2.5 Unregulated operation of the AREBRs
After being started up, the reactors (denoted as GB-AREBR and GB/NPC-AREBR) were switched to unregulated operation, i.e., disconnected from the potentiostat and connected to a potentiometer (digital multimeter, Keithley 2701) to record their potentials using a back slot multiplexer card (Integra series 7700, Keithley). The connection between the reference electrode and the rotating GFB of AREBRs is equivalent to that of an open circuit measurement i.e. the potential of the electrode was not regulated. The reactors were rotated at 20 rpm as shown in Fig. S1B.† The GB and GB/NPC electrodes were connected to the HI port and the Ag/AgCl reference electrodes to the LO port. The measured voltage values are the actual potentials of the AREBRs versus the Ag/AgCl reference. The medium was refreshed when the potentials of the GB-AREBR and GB/NPC-AREBR rose to over 0 V.
2.6 Microbial community analysis
The RNA was extracted from the GB and the GB/NPC samples after open circuit mode operation of AREBRs according to a modified protocol after Lueders.19 The DNA was removed, and the remaining RNA was reversely transcribed into cDNA employing the GoScript Reverse Transcription System of Promega. The amplicons of the V3 region of the 16S rRNA gene were prepared with the primer pair 341f and 515r as described by Bartram et al.20 Subsequently, the Bartram amplicon libraries were sequenced on a NextSeq500/550 (Illumina®, San Diego, CA, U.S.) in the 150 bp paired-end mode. Due to quality loss at the end of the sequences, which was checked by FastQC version 0.10.1 (Simon Andrews; http://www.bioinformatics.babraham.ac.uk/projects/fastqc/), the sequences were trimmed to 130 bp and then processed with the amplicon analysis pipeline. This included a primer dimer purification step by a JAVA program called DimerFilter, a joining step of the forward and reverse reads with a 20 percent mismatch and a minimum overlap of 6 bp via Fastq-join,21 a conversion step of the joined sequence reads to the FASTA format, a chimera check step performed with Uchime (Usearch 5.2.32;22) against the gold database provided by ChimeraSlayer (http://drive5.com/otupipe/gold.tz) and finally a classification step via the RDP classifier 2.10.1 (ref. 23 and 24) with a confidence value of 0.5, which is recommended for short read amplicon data to perform taxonomic dependent analyses of the bacterial community.
3. Results and discussion
3.1 Start-up of the AREBRs
The bioelectrocatalytic CA and CV profiles of the GB and GB/NPC during the accumulation and growth of exoelectrogens under aerobic conditions are shown in Fig. 2. The CVs show that the initial negative ORR current response at the blank electrodes decreased from −0.17 to −0.05 mA cm−2 (Fig. 2B traces 1 and 2) and −0.4 to −0.15 mA cm−2 (Fig. 2D traces 1 and 2), for the GB and GB/NPC electrodes, respectively. It suggests that aerobic biofilms had already grown onto the GB as well as the GB/NPC supports due to favorable conditions for anodic respiration after 3 to 6 days, thus blocking oxygen diffusion to the electrode surface and reducing the ORR current. This occurred in combination with negligible current flow from the anodes during CA operation until 6 to 8 days as can be seen in Fig. 2A and C. After this initial period, both reactors showed a steady increase in the oxidation current in subsequent cycles and reached a maximum of approx. 0.65 ± 0.11 mA cm−2 by 10 to 12 days of operation (Fig. 2A and C).
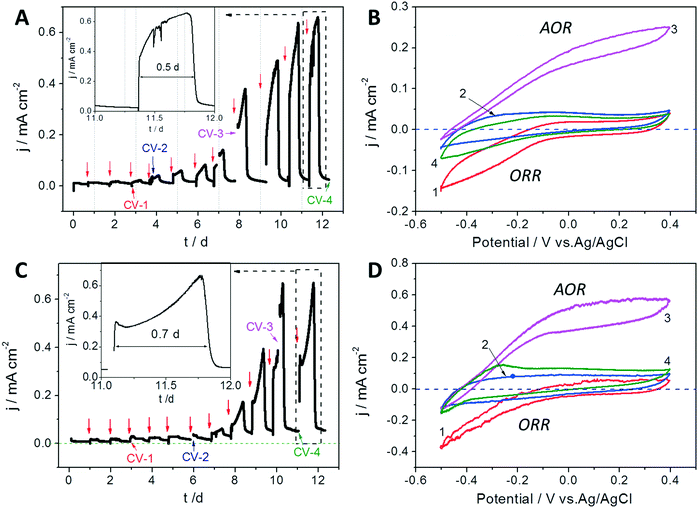 |
| Fig. 2 Bioelectrocatalytic current generation (CA profiles) of the rotating (A) GB and (C) GB/NPC electrodes operated under aerobic conditions. CVs of the rotating (B) GB and (D) GB/NPC electrodes recorded during different stages of the CA operation. CV numbers correspond to A to B and C to D. The red arrows indicate an exchange of the medium. Note: ORR: oxygen reduction reaction, AOR: acetate oxidation reaction. | |
In addition to the development of a plateau in the maximum current output, the CVs obtained under substrate turnover conditions (trace 3 in both Fig. 2B and D) show profiles approaching typical sigmoidal shapes as reported for electrochemically active biofilms cultivated under anoxic conditions.25 Also, the CVs recorded under substrate non-turnover conditions (trace 4 in Fig. 2B and D) do not show any definite indication of the ORR current. Notably, the non-turnover CV of the GB/NPC shows an oxidative peak at approx. −0.3 V. The plateau in the maximum current generation and typical electrochemically active biofilm CV profiles demonstrate that stable exoelectrogen biofilms were cultivated on the surfaces of GB and GB/NPC supports. The cultivation of exoelectrogens is usually conducted under anoxic conditions. However, herein we show that the exoelectrogens could also be directly co-cultured together with aerobic microorganisms on rotating GB and GB/NPC electrodes.
3.2 Operation of the AREBRs
After achieving stable maximum current generation, the GB and GB/NPC supports were disconnected from the potentiostat and shifted to unregulated operation, named GB-AREBR and GB/NPC-AREBR, respectively, using the setup shown in Fig. S1B.†Fig. 3A shows that after being shifted to unregulated operation under aerobic conditions the GB-AREBR and GB/NPC-AREBR firstly displayed a negative potential of about −0.550 V, which lasted for variable time periods. Then, the potential increased gradually to above zero. After refilling the reactors with fresh media, their potentials rapidly reduced again to around −0.550 V and then gradually increased again. The shift from positive to negative potential corresponded exactly to the periodic media exchange. Notably, the GB-AREBR showed this behavior immediately, whereas the GB/NPC-AREBR required 120 h to achieve this state. Once the COD of the media reduced below 100 mg L−1, the potentials of both GB-AREBR and GB/NPC-AREBR started to increase (Fig. 3A and B). The periodic potential change was most likely caused by the development of mixed potential, a phenomenon reported earlier.26 The mixed potential in this study was generated by the multiple (bio)electrochemical processes likely to be occurring simultaneously at the AREBRs. These include bioelectrochemical acetate oxidation (AOR) by the exoelectrogens and electrochemical ORR by the supports.
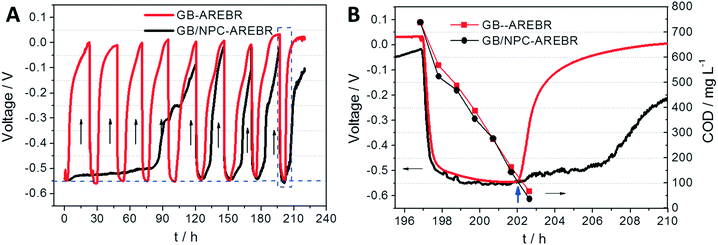 |
| Fig. 3 (A) Potential profiles of GB-AREBR and GB/NPC-AREBR under aerobic conditions. Arrows in (A) represent refreshment of media composed of real wastewater with extra 10 mM acetate. (B) Zoomed in potential plots of the dashed frame in (A) along with the corresponding COD plots for the GB-AREBR and GB/NPC-AREBR. The blue arrow points out the start in the increase of potential when COD reduces below 100 mg L−1. | |
Fig. 3B shows that the decrease of COD values from 740 ± 85 mg L−1 to lower than 100 ± 12 mg L−1 took about 0.21 day (5 h) by the AREBRs during unregulated operation. In the case of a potentiostatically controlled process, the removal of 1400 ± 124 mg L−1 COD under aerobic conditions occurred within about 0.5 to 0.7 days (insets of Fig. 2A and C), while the removal of the half COD (740 ± 85 mg L−1) by the anoxic GB and GB/NPC bioanodes required nearly one day (Fig. S2†). These results demonstrate that the AREBRs had a higher COD removal efficiency than the anoxic bioanode, although further research in this direction is warranted. Moreover, the COD consumption rates of the AREBRs of 103.6 ± 11.9 gCOD m−2 d−1, normalized to the surface area of the brush was higher than the removal rates of the traditional RBC of 11–47 gCOD m−2 d−1 during the treatment of domestic wastewater.27 This demonstrates the feasibility of the process for wastewater treatment.
After operation for 210 h, the status of the exoelectrogens in the AREBRs was investigated by CV tests. The CVs of both GB-AREBR and GB/NPC-AREBR delivered acetate oxidation reaction (AOR) current response in the presence of the substrate under aerobic conditions (Fig. 4A and B). This suggested the survival and activity of exoelectrogens in the GB-AREBR and GB/NPC-AREBR, which was later confirmed by the microbial community analysis (section 3.4). Moreover, the GB/NPC-AREBR could generate a high AOR current density of 0.56 ± 0.08 mA cm−2, slightly lower than its initial maximum value of 0.65 ± 0.11 mA cm−2 attained during the selection process (Fig. 2C and 4B). However, the GB-AREBR generated a much lower AOR current density of only about 0.10 ± 0.01 mA cm−2 (Fig. 4A), which is one sixth of its initial value (Fig. 2A). The higher AOR current density suggests the survival and activity of more exoelectrogens in the GB/NPC-AREBR than in the GB-AREBR (see section 3.4). It also indicates that most of the pre-grown exoelectrogens survived in the GB/NPC-AREBR, but only a small part was preserved in the GB-AREBR.
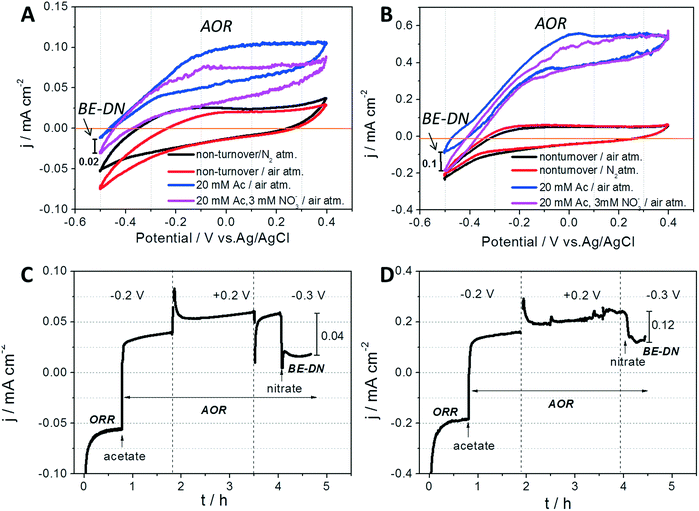 |
| Fig. 4 CVs of the (A) GB-AREBR and (B) GB/NPC-AREBR recorded after the period of unregulated operation in a N2 and air atmosphere (black line), and under non-turnover (no substrate; red line) and in the presence of 20 mM acetate (Ac) without (blue line) and with 3 mM nitrate (pink line). CA curves of the (C) GB-AREBR and (D) GB/NPC-AREBR operated under an air atmosphere and obtained at different conditions (no acetate, with acetate, and with acetate and nitrate under applied potentials of −0.2, +0.2 and −0.3 V, respectively). The vertical arrows represent the injection of 20 mM acetate (Ac) and 3 mM nitrate, respectively. Note, AOR: acetate oxidation reaction, ORR: oxygen reduction reaction, BE-DN: bioelectrochemical denitrification. | |
3.3 Synergistic bioelectrochemical denitrification
As discussed in the preceding paragraph, the AREBRs possess functionalities of AR and AOR by the exoelectrogens and electrocatalyzed ORR. These were confirmed further through the CA tests which revealed that the current generation by both the GB-AREBR and GB/NPC-AREBR shifted from the negative ORR current to positive AOR current after injection of 20 mM acetate (Fig. 4C and D). Interestingly, the AREBRs were also able to conduct bioelectrochemical denitrification under aerobic conditions simultaneously. This was evident from the decrease of positive AOR current after injection of 3 mM nitrate in both AREBRs operated under a constant potential of −0.3 V (Fig. 4C and D). The current density decrease at the GB/NPC-AREBR (0.12 mA cm−2) was greater than that at the GB-AREBR (0.04 mA cm−2). The BE-DN behavior of the AREBRs was also confirmed by the CV results. As shown in Fig. 4A and B, the CVs of the GB-AREBR and GB/NPC-AREBR recorded in the presence of 20 mM acetate and 3 mM nitrate (pink color) display an increase of negative current compared to that recorded in the presence of 20 mM acetate without nitrate (blue color). The negative current increase can be most likely ascribed to the BE-DN by the exoelectrogens biofilms.28,29 The BE-DN current density at −0.5 V potential for the GB/NPC-AREBR is approximately 0.1 mA cm−2, which is much higher than that for the GB-AREBR (0.02 mA cm−2). This could be linked to the survival and activity of more exoelectrogens at the GB/NPC-AREBR. However, the detected BE-DN at the AREBR might be explained by the presence of Arcobacter spp. (see section 3.4) although a recent study suggested that Arcobacter spp. are more likely nitrate reducers rather than denitrifiers based on genome analyses data.30
3.4 Microbial community composition
In order to confirm the existence and synergistic interactions of exoelectrogens with other microorganisms in AREBRs, the microbial community analysis of the biofilms grown at the GB and GB/NPC was conducted. The results based on a mean value of the six GB and the six GB/NPC samples are summarized in Table S1 and Fig. S3.† The microbial communities in the GB/NPC-AREBR and GB-AREBR show a dominance of Alphaproteobacteria of 44.58% ± 4.84% and 54.65% ± 3.40%, respectively. This is in contrast to the dominance of Deltaproteobacteria (over 80%) in biofilms grown at electrodes under anoxic conditions.31 The dominant microbial genera at the GB/NPC-AREBR and GB-AREBR electrodes were Neomegalonema (40.39% ± 4.80% vs. 51.90% ± 3.74%), Arcobacter (15.16% ± 4.03% vs. 1.21% ± 1.14%) and Thauera (2.29% ± 0.20% vs. 9.20% ± 1.35%), respectively. The predominant genus in both AREBRs, Neomegalonema, consists of filamentous bacteria capable of forming biofilms and belongs to the family of the Methylobacteriaceae within the Alphaproteobacteria. They are usually aerobic and were originally isolated from mixed liquors of activated sludge systems in wastewater treatment plants.32 Their dominance can thus be linked to the substrate oxidation reactions through AR of the supplemented acetate in the aerobically operated bioreactors in this study. Among the identified genera, the biggest difference between the GB/NPC-AREBR and the GB-AREBR bacterial composition was the presence of the genus Arcobacter. The Arcobacter are Campylobacter-like organisms of the class Epsilonproteobacteria and have been demonstrated to be electrochemically active and able to generate electrons using acetate as the carbon source.33 The abundance of the Arcobacter in the GB/NPC-AREBR (>15%) was 10-fold higher than that in the GB-AREBR (only about 1.2%) probably explaining the 6-fold decrease of the AOR current density mentioned in section 3.2. Additionally, Arcobacter can also survive under aerobic conditions because they show excellent aerotolerant performance34 perfectly matching the conditions in the GB/NPC-AREBR and the GB-AREBR systems introduced in the current study. The Thauera genus displays higher abundance values in the GB-AREBR than in the GB/NPC-AREBR. Interestingly, one species, Thauera humireducens, had even been isolated from a microbial fuel cell.35 The decrease of Arcobacter in the GB-AREBR compared to the GB/NPC-AREBR resulted in the increase of Thauera in the GB-AREBR compared to the GB/NPC-AREBR as Thauera spp. are able to grow on now available acetate as reported earlier.35 However, the discrete function of Thauera in the AREBRs was not studied in detail. The Geobacter genus, which is a well-known exoelectrogen and responsible for electricity generation under anoxic conditions, was detected in a relatively small quantity in the biofilm of the GB/NPC-AREBR (0.033% ± 0.047%) and the GB-AREBR (0.0029% ± 0.0015%) due to the aerobic conditions used for experiments in this study. This in turn confirms the assumption that Arcobacter as an aerotolerant organism was the responsible exoelectrogen producing electricity in the GB/NPC-AREBR and the GB-AREBR systems.
3.5 Growth and synergistic interactions of aerobes and exoelectrogens under aerobic conditions
It is well known that the metabolism and propagation of aerobic microbes under aerobic conditions is a spontaneous process and usually self-assembling onto supports to form a biofilm. Meanwhile, the growth and eventual survival of the exoelectrogens typically require an anoxic environment and continuous electrode respiration via some form of extracellular electron transfer. In typical METs like MFCs, exoelectrogens associated with the anode usually attach to the solid conductor, which acts as the terminal acceptor for electrons generated by AOR metabolism under anoxic conditions. External devices, e.g., a potentiostat or an ORR cathode, connected through an external circuit are usually employed to accept electrons and ensure continuous electrode respiration.1,3,5 Although the growth of aerobes was obvious, the growth of exoelectrogens was not under the aerobic experimental conditions used in this study. How did the exoelectrogens grow and metabolically interact in the AREBRs under such conditions?
Based on the bioelectrochemical results and microbial community data, we propose that the required anoxic environment for the growth of exoelectrogens was most likely provided by the spontaneously formed aerobic biofilm layer at the electrode surfaces. An anoxic environment is usually formed and anaerobes are grown in the presence of sufficient organic substrate transport/supply but insufficient oxygen diffusion, e.g., in the conventional aerobic biofilm reactor used for WWT.36 The AR of the aerobic biofilms could thus be linked to the blockage of oxygen diffusion and formation of anoxic microenvironments on electrodes under aerobic conditions. It has been reported that under the protection of a layer of hydrogel declining oxygen diffusion, exoelectrogens grown on the bioanode of the MFC exhibited similar bioelectrocatalytic activity in media purged with pure oxygen (aerobic) to one being purged with N2 (anaerobic).37 Though the use of artificial hydrogel a protective layer might be impractical and unsustainable for the application in WWT, it demonstrated that exoelectrogens, if given protection from immediate exposure to oxygen, could maintain bioelectrochemical activity.
During unregulated operation of the AREBRs under aerobic conditions, the in situ ORR of the electrode supports most likely sustained the continuous electrode respiration of the exoelectrogens. As for this assumption, the in situ catalyzed ORR by the GB and GB/NPC supports could accept the electrons released by the exoelectrogens as would occur in a MFC where the anode and cathode are short-circuited. As shown in Fig. 5A, the GB support shows a lower intrinsic ORR electrocatalytic activity with an onset potential at about −0.02 V. After modifying the GB with a layer of N,P co-doped carbon through a binder-free coating method, the obtained GB/NPC support showed a much more positive ORR onset potential at +0.15 V and delivered two times higher reduction current density of 1.64 mA cm−2 (Fig. 5B) at a potential of −0.5 V compared to the pristine GB with 0.82 mA cm−2 (Fig. 5A). The better bioelectrocatalysis accompanied by the increased presence of the Arcobacter sp. exoelectrogen at the GB/NPC-AREBR than the GB-AREBR during unregulated operation could thus be attributed to the higher ORR electrocatalytic activity, and in turn, more efficient electrode respiration of the exoelectrogens at the GB/NPC support (Fig. 5B).
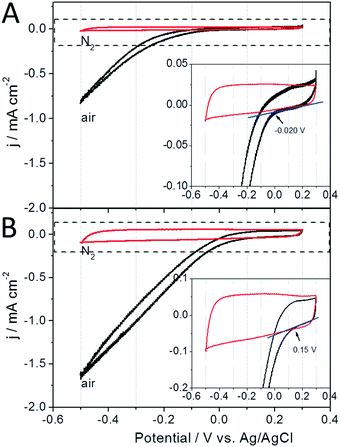 |
| Fig. 5 Cyclic voltammograms of abiotic rotating (at 20 rpm speed) (A) GB and (B) GB/NPC electrodes recorded at a scan rate 0.5 mV s−1 in 50 mM PBS with a pH of 7.0. Inset diagrams show the zoomed in view of the respective dashed boxes. | |
Based on the obtained results and different possible microbial interactions, the biofilm structure during the potential-controlled and operation processes is proposed in Fig. 6. The description for the proposed biofilm model is presented below.
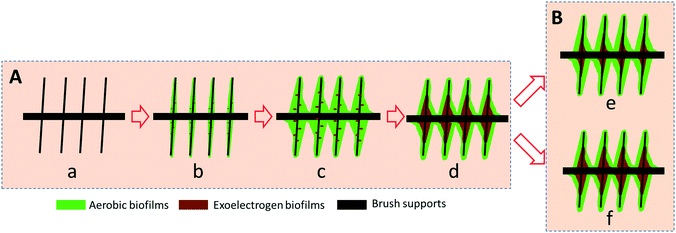 |
| Fig. 6 Schematic diagrams show cross-sectional view of the proposed biofilm structures at the GB and GB electrodes. (A) Potential-controlled selection and growth of exoelectrogens and simultaneous assembly of aerobic biofilms: (a) initial brush support, (b and c) aerobic biofilms with a small amount of exoelectrogens covered brush support and (c) aerobic (outer) and exoelectrogen (inner) biofilms covered brush support. (B) GB-AREBR (d) and GB/NPC-AREBR (e) after operation for 210 h. | |
A) In the beginning of the potential-controlled process, a few aerotolerant Arcobacter species most likely co-attached to the conductive support along with the aerobic microbes (Fig. 6Aa–c). Once the biofilms dominated by Neomegalonema achieved a sufficient thickness to block the diffusion of oxygen and provide an anoxic environment, the Arcobacter species started to propagate and form biofilms on the surface of the conductive brush supports. Eventually, a two-layer biofilm structure comprised of exoelectrogens and aerobic microbes was formed in the AREBRs (Fig. 6Ad).
B) When the AREBRs were subjected to unregulated operation, the quantity of the active Arcobacter biofilm at the GB/NPC-AREBR was preserved due to the high in situ ORR electrocatalytic activity and thus efficient electrode respiration at the GB/NPC support (Fig. 6Be). However, the active exoelectrogens at the GB-AREBR decreased due to the low ORR electrocatalytic activity of the GB support (Fig. 6Bd).
The possibility of the multilayered biofilm that can achieve the different metabolic reactions discussed so far based on the presence of different electron acceptors namely oxygen, nitrate, and conductive supports is not ruled out. However, this needs thorough investigation into this direction.
4. Conclusions
This study presents a new concept of A-MET in which aerobes and exoelectrogens are cultivated together as a multilayered biofilm on the same conductive support under aerobic conditions and are able to metabolize synergistically in order to grow and survive. The underlying principles of the A-MET were tested through the use of the AREBR reactor concept.
(a) The start-up and operation of the AREBR was demonstrated under aerobic conditions.
(b) The anaerobic/anoxic microenvironment for the proliferation of aerotolerant exoelectrogens was provided by the aerobic microorganisms grown at the outer layers of the biofilms, which served as a protective layer to block the oxygen diffusion to the inner layer.
(c) The continuous electrode respiration of the exoelectrogens was sustained by the in situ ORR catalyzed by the conductive support with intrinsic ORR electrocatalytic activity. The GB/NPC support with a higher intrinsic ORR activity retained a higher degree of flexibility regarding its functionality.
(d) The AREBR possesses multi-functionalities of AR, AOR, ORR and BE-DN that could take place simultaneously and synergistically under aerobic conditions.
The proposed A-MET concept combining the novel interactions and metabolic capabilities of both aerobes and exoelectrogens at the same solid–liquid interface could have implications in simplifying the application and integration of MET based technologies in wastewater treatment processes.
Conflicts of interest
There are no conflicts to declare.
Acknowledgements
We thank Mrs. Alicia Geppert (DSMZ) for the preparation of the V3 amplicon libraries. S. Chen acknowledges the financial support from the China Scholarship Council, the National Natural Science Foundation of China (grant 51678281), the Science and Technology Project of Jiangxi Province (grants 20161BCB24005 and 20162BCB23024), and the project of the Education Department of Jiangxi Province (grants, GJJ150296). S. A. Patil acknowledges the Alexander von Humboldt Foundation for the financial support.
References
- A. P. Borole, G. Reguera, B. Ringeisen, Z.-W. Wang, Y. Feng and B. H. Kim, Electroactive biofilms: Current status and future research needs, Energy Environ. Sci., 2011, 4, 4813–4834 RSC.
- D. R. Lovley, Electromicrobiology, Annu. Rev. Microbiol., 2012, 66, 391–409 CrossRef CAS PubMed.
- B. E. Logan, Exoelectrogenic bacteria that power microbial fuel cells, Nat. Rev. Microbiol., 2009, 7, 375–381 CrossRef CAS PubMed.
- K. Rabaey and W. Verstraete, Microbial fuel cells: novel biotechnology for energy generation, Trends Biotechnol., 2005, 23, 291–298 CrossRef CAS PubMed.
- F. Harnisch and U. Schroder, From MFC to MXC: chemical and biological cathodes and their potential for microbial bioelectrochemical systems, Chem. Soc. Rev., 2010, 39, 4433–4448 RSC.
- B. E. Logan and J. M. Regan, Microbial challenges and applications, Environ. Sci. Technol., 2006, 40, 5172–5180 CrossRef CAS PubMed.
-
K. Rabaey, L. Angenent, U. Schroder and J. Keller, Bioelectrochemical Systems: From Extracellular Electron Transfer to Biotechnological Application, IWA Publishing, London, 1st edn, 2009 Search PubMed.
- M. Sun, L.-F. Zhai, W.-W. Li and H.-Q. Yu, Harvest and utilization of chemical energy in wastes by microbial fuel cells, Chem. Soc. Rev., 2016, 45, 2847–2870 RSC.
- W.-W. Li, H.-Q. Yu and Z. He, Towards sustainable wastewater treatment by using microbial fuel cells-centered technologies, Energy Environ. Sci., 2014, 7, 911–924 RSC.
- S. Sevda, T. R. Sreekishnan, N. Pous, S. Puig and D. Pant, Bioelectroremediation of perchlorate and nitrate contaminated water: A review, Bioresour. Technol., 2018, 255, 331–339 CrossRef CAS PubMed.
- B. E. Logan, B. Hamelers, R. A. Rozendal, U. Schrorder, J. Keller, S. Freguia, P. Aelterman, W. Verstraete and K. Rabaey, Microbial fuel cells: Methodology and technology, Environ. Sci. Technol., 2006, 40, 5181–5192 CrossRef CAS PubMed.
- U. Schroder, F. Harnisch and L. T. Angenent, Microbial electrochemistry and technology: terminology and classification, Energy Environ. Sci., 2015, 8, 513–519 RSC.
- K. Y. Cheng, G. Ho and R. Cord-Ruwisch, Anodophilic Biofilm Catalyzes Cathodic Oxygen Reduction, Environ. Sci. Technol., 2010, 44, 518–525 CrossRef CAS PubMed.
- E. Blanchet, S. Pecastaings, B. Erable, C. Roques and A. Bergel, Protons accumulation during anodic phase turned to advantage for oxygen reduction during cathodic phase in reversible bioelectrodes, Bioresour. Technol., 2014, 173, 224–230 CrossRef CAS PubMed.
- D. Millo, An Electrochemical Strategy to Measure the Thickness of Electroactive Microbial Biofilms, ChemElectroChem, 2015, 2, 288–291 CrossRef CAS PubMed.
- K. P. Nevin, P. Zhang, A. E. Franks, T. L. Woodard and D. R. Lovley, Anaerobes unleashed: Aerobic fuel cells of Geobacter sulfurreducens, J. Power Sources, 2011, 196, 7514–7518 CrossRef CAS.
- S. J. Tait and A. A. Friedman, Anaerobic Rotating Biological Contactor for Carbonaceous Wastewaters, J. - Water Pollut. Control Fed., 1980, 52, 2257–2269 Search PubMed.
- S. Chen, S. A. Patil and U. Schröder, A high-performance rotating graphite fiber brush air-cathode for microbial fuel cells, Appl. Energy, 2018, 211, 1089–1094 CrossRef CAS.
- T. Lueders, M. Manefield and M. W. Friedrich, Enhanced sensitivity of DNA- and rRNA-based stable isotope probing by fractionation and quantitative analysis of isopycnic centrifugation gradients, Environ. Microbiol., 2004, 6, 73–78 CrossRef CAS PubMed.
- A. K. Bartram, M. D. J. Lynch, J. C. Stearns, G. Moreno-Hagelsieb and J. D. Neufeld, Generation of Multimillion-Sequence 16S rRNA Gene Libraries from Complex Microbial Communities by Assembling Paired-End Illumina Reads, Appl. Environ. Microbiol., 2011, 77, 3846–3852 CrossRef CAS PubMed.
- E. Aronesty, Comparison of Sequencing Utility Programs, Open Bioinf. J., 2013, 7, 1–8 CrossRef.
- R. C. Edgar, B. J. Haas, J. C. Clemente, C. Quince and R. Knight, UCHIME improves sensitivity and speed of chimera detection, Bioinformatics, 2011, 27, 2194–2200 CrossRef CAS PubMed.
- J. R. Cole, Q. Wang, J. A. Fish, B. Chai, D. M. McGarrell, Y. Sun, C. T. Brown, A. Porras-Alfaro, C. R. Kuske and J. M. Tiedje, Ribosomal Database Project: data and tools for high throughput rRNA analysis, Nucleic Acids Res., 2014, 42, D633–D642 CrossRef CAS PubMed.
- Q. Wang, G. M. Garrity, J. M. Tiedje and J. R. Cole, Naive Bayesian classifier for rapid assignment of rRNA sequences into the new bacterial taxonomy, Appl. Environ. Microbiol., 2007, 73, 5261–5267 CrossRef CAS PubMed.
- K. Fricke, F. Harnisch and U. Schroder, On the use of cyclic voltammetry for the study of anodic electron transfer in microbial fuel cells, Energy Environ. Sci., 2008, 1, 144–147 RSC.
- F. Harnisch, S. Wirth and U. Schroeder, Effects of substrate and metabolite crossover on the cathodic oxygen reduction reaction in microbial fuel cells: Platinum vs. iron(II) phthalocyanine based electrodes, Electrochem. Commun., 2009, 11, 2253–2256 CrossRef CAS.
- A. Tawfik, H. Temmink, G. Zeeman and B. Klapwijk, Sewage Treatment in a Rotating Biological Contactor (RBC) System, Water, Air, Soil Pollut., 2006, 175, 275–289 CrossRef CAS.
- K. Y. Cheng, M. P. Ginige and A. H. Kaksonen, Ano-cathodophilic biofilm catalyzes both anodic carbon oxidation and cathodic denitrification, Environ. Sci. Technol., 2012, 46, 10372–10378 CAS.
- N. Pous, A. A. Carmona-Martínez, A. Vilajeliu-Pons, E. Fiset, L. Bañeras, E. Trably, M. D. Balaguer, J. Colprim, N. Bernet and S. Puig, Bidirectional microbial electron transfer: Switching an acetate oxidizing biofilm to nitrate reducing conditions, Biosens. Bioelectron., 2016, 75, 352–358 CrossRef CAS PubMed.
- I. Roalkvam, K. Dronen, R. Stokke, F. L. Daae, H. Dahle and I. H. Steen, Physiological and genomic characterization of Arcobacter anaerophilus IR-1 reveals new metabolic features in Epsilonproteobacteria, Front Microbiol, 2015, 6, 987 Search PubMed.
- S. Riedl, R. K. Brown, S. Kloeckner, K. J. Huber, B. Bunk, J. Overmann and U. Schroeder, Successive Conditioning in Complex Artificial Wastewater Increases the Performance of Electrochemically Active Biofilms Treating Real Wastewater, ChemElectroChem, 2017, 4, 3081–3090 CrossRef CAS.
- T. R. Thomsen, L. L. Blackall, M. A. de Muro, J. L. Nielsen and P. H. Nielsen, Meganema perideroedes gen. nov., sp nov., a filamentous alphaproteobacterium from activated sludge, Int. J. Syst. Evol. Microbiol., 2006, 56, 1865–1868 CrossRef CAS PubMed.
- V. Fedorovich, M. C. Knighton, E. Pagaling, F. B. Ward, A. Free and I. Goryanin, Novel Electrochemically Active Bacterium Phylogenetically Related to Arcobacter butzleri, Isolated from a Microbial Fuel Cell, Appl. Environ. Microbiol., 2009, 75, 7326–7334 CrossRef CAS PubMed.
- V. Prouzet-Mauleon, L. Labadi, N. Bouges, A. Menard and F. Megraud, Arcobacter butzleri: Underestimated enteropathogen, Emerging Infect. Dis., 2006, 12, 307–309 CrossRef PubMed.
- G.-Q. Yang, J. Zhang, S.-W. Kwon, S.-G. Zhou, L.-C. Han, M. Chen, C. Ma and L. Zhuang, Thauera humireducens sp nov., a humus-reducing bacterium isolated from a microbial fuel cell, Int. J. Syst. Evol. Microbiol., 2013, 63, 873–878 CrossRef CAS PubMed.
-
C. L. Grady Jr, G. T. Daigger, N. G. Love and C. D. Filipe, Biological wastewater treatment, CRC press, 2011 Search PubMed.
- M. Li, K. Lv, S. Wu and S. Chen, Immobilization of Anodophilic Biofilms for Use in Aerotolerant Bioanodes of Microbial Fuel Cells, ACS Appl. Mater. Interfaces, 2016, 8, 34985–34990 CrossRef CAS PubMed.
Footnote |
† Electronic supplementary information (ESI) available. See DOI: 10.1039/c8ew00530c |
|
This journal is © The Royal Society of Chemistry 2019 |
Click here to see how this site uses Cookies. View our privacy policy here.