DOI:
10.1039/C8MH00888D
(Communication)
Mater. Horiz., 2019,
6, 160-168
Soft stimuli-responsive grippers and machines with high load-to-weight ratios†
Received
27th July 2018
, Accepted 15th October 2018
First published on 15th October 2018
Abstract
Soft untethered grippers and machines are emerging fields of research due to their wide range of desirable properties and capabilities over traditional hard robots. Stimuli-responsive hydrogels are naturally soft and untethered (i.e., they respond to a signal from the environment); however, they are not used as grippers due to the weak mechanical properties of the hydrogels. This manuscript describes the fabrication of a general class of stimuli-responsive grippers that are soft and untethered. Each gripper is composed of a stimuli-responsive hydrogel with a hole in the middle for gripping onto objects and a layer of coating around its sides. Importantly, these grippers exert surprisingly large gripping forces relative to their weights—∼10
000, which is the highest reported in the literature for a gripper. This high load-to-weight ratio is unexpected because the stimuli-responsive hydrogel is soft, not tethered to any external supply of energy, and fabricated by simple methods. The approach is general: high load-to-weight ratios were achieved for both pH- and temperature-responsive hydrogels. As soft grippers, they gripped onto objects of different shapes and sizes easily. A class of soft untethered machines can be fabricated by assembling these soft grippers with different modular blocks of stimuli-responsive hydrogels for performing complex operations.
Conceptual insights
A general class of stimuli-responsive grippers and actuators is introduced. Stimuli-responsive hydrogels are “smart” materials with a wide range of applications; however, hydrogels are mechanically weak and not used as grippers and actuators. This manuscript describes a composite material that consists of a stimuli-responsive hydrogel and a layer of coating around it. This stimuli-responsive gripper has a surprisingly high load-to-weight ratio: ∼10 000. This high ratio is unexpected because the gripper is soft, not tethered to an external source of energy, fabricated using simple methods, and primarily made of hydrogels that are not known to be mechanically strong. In addition, it is also the highest ratio reported in the literature for other types of (tethered or untethered) gripper, including those made from other types of materials. The phenomenon is general: high load-to-weight ratios were achieved for temperature-responsive and pH-responsive grippers. Therefore, the transformations of the hydrogels at the molecular level (i.e., hydrophobic to hydrophilic transition or electrostatic repulsion) due to the stimuli are capable of exerting large forces on the surroundings. A novel general class of soft untethered machines can be fabricated by assembling building blocks of stimuli-responsive hydrogels and grippers for the emerging and exciting field of soft autonomous robotics.
|
Introduction
The development of soft untethered machines is an emerging field of research due to the wide range of features and potential applications that the machines can provide.1,2 Being soft, these machines have many desirable properties (e.g., deformable, stretchable, and the ability to absorb shock), and can be highly sensitive and adaptive.1,3,4 Being untethered, these machines can be used, for example, in typically inaccessible (e.g., the human body) or hostile (e.g., corrosive) environments. Nature has demonstrated that many soft autonomous organisms are capable of performing exceedingly complex and functional operations; hence, the development of soft untethered machines as biomimetic systems ultimately promises to approach the level of complexity of biological systems.
In particular, it is advantageous to use soft untethered systems for gripping onto objects. Gripping is one of the most important and fundamental operations of machines, and has a wide range of applications including manufacturing, surgery, and professional cooking.5 Traditional grippers are made from hard materials, and typically consist of two or more pointed tips (or “fingers”). It can, however, be technically challenging for these grippers to pick up objects of different sizes, shapes, and mechanical properties. Complex algorithms are needed to determine the many degrees of freedom required for these operations (e.g., amount of displacement, rotation, and bending of the fingers). For gripping onto objects that are soft and/or easily breakable (e.g., an uncooked egg), the operation becomes even more challenging (e.g., pressure sensors, controllers, and feedback mechanisms are needed). On the other hand, grippers made of soft materials can greatly reduce the complexity of the operation (e.g., using only one external variable such as air pressure or electric potential), can grip onto objects of different sizes and shapes easily, and are less likely to deform or break soft objects.1,6–10
However, many soft grippers reported in previous studies are tethered: they need to be connected to an external source for supplying the energy of actuation (e.g., supply of air or electric power). Untethered grippers have many important biomedical applications, such as for surgery, biopsy, and drug delivery.11–14 The untethered grippers reported in previous studies, however, are typically composed of significant proportions of hard materials (e.g., metals), are sophisticated in design (e.g., they consist of complex shapes, many parts, and different types of materials), are technically challenging to fabricate, and/or have low gripping strength.13,15–17
Stimuli-responsive hydrogels are a class of materials that can expand or contract when an external stimulus is applied. These hydrogels have been studied widely, and can be fabricated to respond to a wide variety of stimuli, such as pH, temperature, light, electric field, pressure, gases, ions, alcohols, glucose, and many types of biomolecule (e.g., enzymes and antigens).18,19 Stimuli-responsive hydrogels are naturally soft, untethered, and responsive to their surroundings; hence, they may be candidates for soft untethered machines. However, being soft materials, these stimuli-responsive hydrogels have weak mechanical properties and are not known to exert a significant amount of force on their surroundings.20–22 This property of the hydrogels has greatly limited their range of potential applications. In general, any gripper that is soft and untethered is not expected to have significant gripping strength.
In this study, we fabricated a general class of soft untethered grippers that are responsive to the stimulus of the local environment. The gripper consists of a piece of a soft stimuli-responsive hydrogel with a hole in the middle for gripping onto objects, and a layer of coating around its sides. We found that they are able to hold onto surprisingly heavy objects relative to their weights—the load-to-weight ratios are on the order of 10
000. Our results thus showed that stimuli-responsive hydrogels can actually exert large amounts of force on their surroundings when properly designed and fabricated with other components (e.g., with a layer of coating) despite being a soft material.
Experimental
Materials
Acrylic acid, 2-hydroxyethyl methacrylate, ethylene glycol dimethacrylate, 2,2-dimethoxy-2-phenylacetophenone, N-isopropylacrylamide, ammonium persulfate, N,N,N′,N′-tetramethylethylenediamine, methacrylic acid, and 2-acrylamido-2-methylpropanesulfonic acid were purchased from Sigma-Aldrich. Methanol was purchased from Fisher Chemical. Sodium hydroxide was purchased from Merck Millipore. Acrylamide was purchased from Amresco. N,N′-Methylenebisacrylamide was purchased from Alfa Aesar. The adhesive (Scotch) was purchased from 3M. The Sylgard 184 silicone elastomer was purchased from Dow Corning for preparing polydimethylsiloxane (PDMS). Syringe needles were purchased from Terumo Corporation. The SU-8 photoresist was purchased from Microchem Corporation.
Fabricating PDMS molds
In order to fabricate the stimuli-responsive grippers of specific shapes and sizes, we made PDMS molds with cavities of the required shapes and sizes. A 3D printer (UP Plus 2, Axpert Global Pte. Ltd) was first used to print rectangular blocks made of acrylonitrile butadiene styrene (ABS) of different sizes. These polymeric blocks were then adhered onto the bottom surface of a Petri dish using a double-sided tape. In a separate step, we prepared PDMS by first mixing the base and the curing agent from the Sylgard 184 kit in a ratio of 10
:
1. After stirring and mixing the liquid for 2 h, we used a vacuum pump to degas the mixture for 30 min. This PDMS pre-polymer solution was poured into the Petri dish containing the ABS blocks. The volume of the pre-polymer solution used was sufficient to cover the top surface of the blocks completely. The Petri dish was then placed in an oven at 80 °C for 30 min. After curing the PDMS, we extracted the ABS blocks out of the PDMS; thus, the PDMS had cavities that can be used as the mold for fabricating the stimuli-responsive grippers.
Fabricating the temperature-responsive hydrogel (without any adhesive coating)
We mixed N-isopropylacrylamide (0.3 g), N,N′-methylenebisacrylamide (5 mg) as the cross-linker, deionized water (1.5 mL), 5 wt% ammonium persulfate solution (50 μL) as the initiator, and N,N,N′,N′-tetramethylethylenediamine (30 μL) as the accelerator in a vial. This mixture was poured into the cavity of a PDMS mold. A syringe needle of 400 μm diameter was placed vertically through the center of the cavity (i.e., it pierced through the bottom center of the mold). The mold was then placed in a humidity chamber (humidity of ∼90%) for 24 h. After removing the syringe needle, the polymerized piece of the hydrogel (with a hole in the center) was extracted from the mold. The procedure was repeated for molds with cavities of different lengths that ranged from 2 mm to 6 mm. Each of the cavities had the same length and width. All the cavities had a depth of 2 mm.
Fabricating the temperature-responsive gripper
We mixed N-isopropylacrylamide (0.3 g), N,N′-methylenebisacrylamide (5 mg) as the cross-linker, deionized water (1.5 mL), 5 wt% ammonium persulfate solution (50 μL) as the initiator, and N,N,N′,N′-tetramethylethylenediamine (30 μL) as the accelerator in a vial. This mixture was poured into a PDMS mold with a cavity of dimensions 30 mm × 30 mm × 5 mm. The relatively large dimensions of this mold were used for making nine grippers at the same time. In the mold, we placed nine syringe needles (each of them had a diameter of 1.2 mm) arranged in a 3 by 3 configuration for creating the hole in the middle of each gripper. The syringe needles were placed vertically through the mold (i.e., they pierced through the bottom of the mold). The mold was then placed in a humidity chamber (humidity of ∼90%) for 24 h. After removing the syringe needles, the polymerized piece of the hydrogel (with nine holes) was extracted from the mold. The hydrogel was then immersed in deionized water at 20 °C. After 2 hours, the hydrogel absorbed water and expanded; consequently, the size of the holes increased from 1.2 mm in diameter to 1.8 mm. At this state, we removed the hydrogels from water and cut it into nine smaller pieces of hydrogels of equal size. The dimensions of each piece of the hydrogel were 2.5 mm × 2.5 mm × 1.0 mm. We placed each piece of the hydrogel on a PDMS support, and inserted a syringe needle of 0.8 mm diameter into the hole of the hydrogel. The hydrogel was dried in an oven at 80 °C for 30 min. The heat from the oven caused the water in the hydrogel to evaporate and the size of the hydrogel reduced. As a result, the hole shrunk and the hydrogel wrapped itself around the syringe needle. At this state, the hydrogel had a size of 1.2 mm × 1.2 mm × 0.5 mm, and a hole of 0.8 mm diameter. After drying, it was quickly coated with the adhesive on its four sides. After 20 min, we removed the syringe needle, and the gripper was ready for use.
Fabricating the pH-responsive gripper
We mixed acrylic acid (4.3 wt%), 2-hydroxyethyl methacrylate (64.3 wt%), deionized water (28.6 wt%), ethylene glycol dimethacrylate (0.7 wt%) as the cross-linker, and 2,2-dimethoxy-2-phenylacetophenone (2.1 wt%) as the photoinitiator in a vial. The mixture was poured into a PDMS mold with a cavity of dimensions 5 mm × 5 mm × 2 mm. A syringe needle (800 μm in diameter) was placed vertically through the center of the mold (i.e., it pierced through the bottom center of the mold). The hydrogel was then cured with ultraviolet light (Omnicure S2000) for 20 min with a wavelength of 365 nm and an intensity of 10 mW cm−2. After curing, we extracted the hydrogel from the PDMS mold, and cut the hydrogel around the hole manually. The hydrogel had dimensions of 1.3 mm × 1.3 mm × 0.6 mm, and a hole of 0.8 mm diameter in the center after cutting. We then inserted a syringe needle of diameter 0.8 mm through the hole of the pH responsive hydrogel, and dried the hydrogel in an oven at 80 °C for 30 min. After drying, the size of the hydrogel reduced. As a result, the hole shrunk, and the hydrogel wrapped itself around the syringe needle. At this state, the hydrogel had a size of 1.2 mm × 1.2 mm × 0.5 mm, and a hole of 0.8 mm diameter. We then coated the four sides of the hydrogel with the adhesive, and left it to dry for 20 min. After removing the syringe needle, the gripper was ready for use.
Fabricating objects of different shapes and sizes
In order to test if the soft gripper was capable of gripping onto different types of object, we made a few objects of different shapes and sizes. The objects were made of the SU-8 photoresist using a standard procedure in photolithography. First, we pre-baked the SU-8 at 65 °C for 1 min, and then at 95 °C for 20 min. After that, the SU-8 was cooled down to room temperature for 10 min. Subsequently, we placed a piece of photomask (that was printed with the specific sizes and shapes of the objects) over the SU-8, and exposed the photomask to UV light for 3 min. The SU-8 was then baked at 55 °C for 10 min. The temperature was gradually ramped up to 70 °C for 10 min, then 95 °C for 5 min, and then 110 °C for 20 min. After heating, the SU-8 was allowed to cool at room temperature for at least 2 h. It was then soaked in a developer solution for 20–30 min, while changing the solution every 10 min. Finally, it was rinsed with acetone and soaked in diethyl ether before use.
Measuring the load that the gripper can hold onto
The platform that the gripper rested on was a hollow cylindrical object (polyethylene) with an inner diameter of 900 μm, an outer diameter of 2.0 mm, and a height of 5 mm. This cylindrical platform was clamped and secured in place. A metallic rod (i.e., a syringe needle with a diameter that ranged from 400 μm to 800 μm) was inserted through the hole of the hollow cylinder and the hole of the gripper. At the bottom end of the rod, we attached a bin made of aluminium foil. The experimental setup is illustrated in Fig. 2a.
In one set of experiments, we first contracted the hydrogel using an appropriate external stimulus, and opened the hole of the gripper. For rods with diameters of 500 μm or less, the gripper was not able to hold onto the rod and the bin; hence, the rod and the bin fell freely downward under their own weights (0.5 g). For rods with larger diameters, the rod and the bin did not fall down under their own weights due to the adhesiveness of the surface of the hydrogel. In this case, we placed nylon 6/6 pellets (the weight of each pellet is ∼12 mg) one by one into the bin until the gripper lost its grip. We then measured the total weight of the load (i.e., the sum of the weight of the bin, the nylon pellets, and the rod). The maximum load that the gripper can hold onto is the total weight of the load for which the hydrogel lost its grip minus one nylon pellet (i.e., the load just before the gripper lost its grip).
In another set of experiments, we applied an appropriate stimulus to the gripper so that the hole closed and gripped tightly onto the rod. We then repeated the experiment described above in which we added nylon pellets until the gripper lost its grip, and measured the load.
We applied the appropriate stimulus to the grippers as follows. For the temperature-responsive hydrogel without any adhesive coating, we first unclamped the setup and immersed it in a water bath maintained at 40 °C. After 1–2 h, the hydrogel contracted and gripped onto the rod. We then extracted the setup out of the water bath, and clamped the hollow cylindrical platform back in place. For measuring the load, the pellets were placed into the bins one by one. At the same time, we dropped deionized water at 40 °C on the hydrogel every one minute for two purposes: to keep the hydrogel in its contracted state at 40 °C, and to keep it hydrated with water.
For the temperature-responsive gripper that consisted of a hydrogel coated on all its four lateral sides, the procedure for measuring the maximum load is similar to that described in the previous paragraph for the case when the hole of the gripper was opened. However, for measuring the maximum load when the hole of the gripper was closed, we used deionized water at room temperature instead of water at 40 °C. When the gripper was used for gripping the syringe needle with a diameter of more than 400 μm, the hole closed at around 20 min; however, we waited longer (∼1–2 h) in order for the gripper to have a better grip on the objects. For gripping onto the syringe needle of 400 μm diameter, we needed to wait for a longer period of time (∼4–5 h) because the hydrogel needed more time to expand and grip onto the smaller needle. In order to maintain the closed state of the hole, we added droplets of deionized water at room temperature onto the gripper every minute.
For the pH-responsive gripper, we first immersed the setup into a pH 2 solution and allowed the hole to open. After the hole fully opened, we clamped the cylindrical platform back in place. Subsequently, we maintained the fully opened state of the hole by adding drops of the pH 2 solution every one minute onto the pH-responsive hydrogel. For measuring the maximum load when the hole of the pH-responsive gripper was closed, the procedure is the same except that a pH 10 solution was used instead. The hole closed around 30 min; however, we waited longer for around 4–5 h in order for the gripper to have a better grip onto the objects. In order to maintain the fully opened state of the hole, we added droplets of the pH 10 solution onto the gripper every minute.
The weight of the gripper was measured immediately after it lost its grip. Excess water on the surface of the gripper was wiped away gently; however, the bulk volume of the hydrogel remained hydrated.
Fabricating the soft machine
The soft machine we fabricated consisted of three stimuli-responsive components: a temperature-responsive gripper, a salt-responsive hydrogel that served as the base of the machine, and a pH-responsive hydrogel that served as the actuating arm of the machine. The salt-responsive hydrogel was prepared by dissolving 2-acrylamido-2-methylpropanesulfonic acid (0.211 g), acrylamide (0.289 g), sodium hydroxide (0.041 g), N,N′-methylenebisacrylamide (13.3 mg) and ammonium persulfate (4 mg) in deionized water (10 mL). We then added N,N,N′,N′-tetramethylethylenediamine (24 μL), and allowed the solution to polymerize overnight. After polymerization, the hydrogel was soaked in deionized water for 4 h; it was then cut into a size of 5 mm × 3 mm × 3 mm before use.
The pH-responsive hydrogel was prepared by first mixing N,N′-methylenebisacrylamide (1 mg), 2-hydroxyethyl methacrylate (127 mg), methacrylic acid (81 mg), deionized water (0.44 mL), methanol (0.44 mL), 5 wt% ammonium persulfate solution (0.06 mL), and N,N,N′,N′-tetramethylethylenediamine (0.04 mL) in a vial. After mixing, the solution was poured into a mold (ABS) with a cavity of dimensions 4 mm × 2 mm × 1 mm. It was left overnight for the solution to polymerize. After polymerization, it was soaked in a pH 2 solution before use. This pH-responsive hydrogel was prepared in a different way from that described for the other pH-responsive hydrogel used in this study because it responded faster and had a larger swelling ratio (i.e., the ratio of the size of the expanded hydrogel over the size of the contracted hydrogel was larger).
The soft machine was assembled by first adhering the salt-responsive hydrogel onto the surface of a platform (cell culture flask) using an adhesive. One end of the pH-responsive hydrogel was adhered onto the top surface of the salt-responsive hydrogel. On the other end of the pH-responsive hydrogel, we adhered a piece of polypropylene (2 mm × 2 mm × 0.6 mm). Finally, the temperature-responsive gripper was adhered onto the other surface of the piece of polypropylene as illustrated in Fig. 6a.
Results and discussion
Stimuli-responsive grippers without coating
In order to understand the way stimuli-responsive hydrogels exert forces on their surroundings, we first considered possibly the simplest soft untethered gripper: a rectangular slab of a stimuli-responsive hydrogel with a hole in the center. When the hydrogel changes its size due to a stimulus, the hole may close; hence, the hydrogel can grip onto any object placed within the hole. The question is: does the hole close when the hydrogel expands or contracts (Fig. 1a)? Previous studies have reported that when a hydrogel swelled (e.g., in deionized water), the hole in the hydrogel closed.23,24 These studies, however, used hydrogels that were adhered onto a rigid substrate.
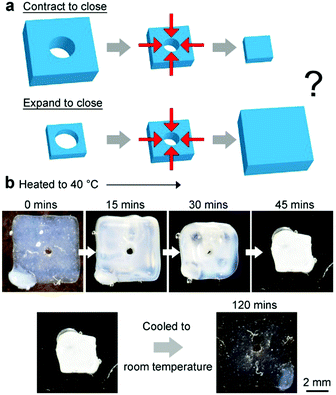 |
| Fig. 1 Stimuli-responsive hydrogels as grippers. Grippers can be fabricated by making a hole in the center of a slab of a stimuli-responsive hydrogel. (a) By applying a stimulus on the hydrogel, the hydrogel changes its size and closes the hole; thus, it can grip onto an object placed within the hole. The question is: will the hole close when the hydrogel expands or contracts? (b) Images showed that after heating a temperature-responsive hydrogel to 40 °C, the hydrogel contracted and the hole closed. The process was reversible: when the hydrogel expanded at room temperature, the hole reopened. | |
We found from our experiments that for free-standing hydrogels (without coating), the hole closed when the hydrogel contracted (Fig. 1b). Experimentally, we fabricated a series of temperature-responsive hydrogels (PNIPAM) with the same diameter of the hole, Dh (400 μm), the same thickness, tgel (2 mm), but different lengths, Lgel (see Experimental). For all these hydrogels of different lengths, the hole shrunk when the hydrogel contracted (at 40 °C), and opened when the hydrogel expanded (at room temperature). The process was reversible.
Subsequently, we determined the gripping strength of this uncoated piece of the hydrogel (Lgel = 6 mm) with a hole in the center (400 μm). Fig. 2a shows the schematic of the experimental setup for measuring the maximum load that the hydrogel can hold onto. In brief, the hydrogel was allowed to grip onto a rod (400 μm in diameter) that had a bin attached to its bottom end through the hole in the middle of the hydrogel (see Experimental). When the hole of the hydrogel was fully opened at room temperature, the rod (and the bin) fell freely downward through the hole due to gravity. At 40 °C, the hole closed and the hydrogel gripped onto the rod. Small polymeric pellets (12 mg) were then placed into the bin one by one. When there was sufficient weight in the bin, the hydrogel lost its grip, and the load fell downward. The maximum load (2.9 ± 0.5 g for five measurements) was the total weight of the rod, bin, and all the polymeric pellets just before the hydrogel lost its grip. We measured the weight of the hydrated hydrogel (22 mg) immediately after it lost its grip. The load-to-weight ratio was determined to be a reasonably high value of 130. On the other hand, the hole with Dh = 400 μm closed completely only when Lgel ≥ 6 mm; hence, the gripper is unnecessarily bulky (i.e., Lgel ≫ Dh).
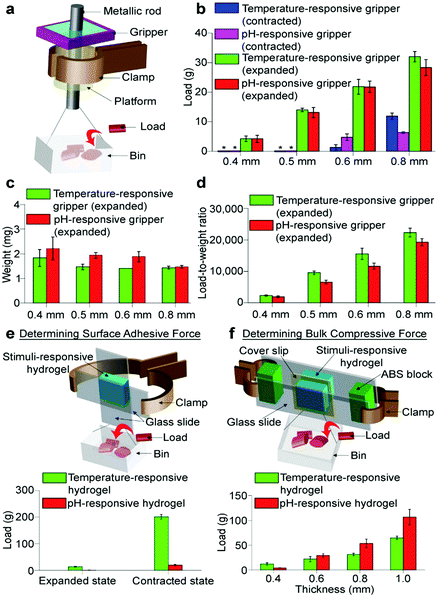 |
| Fig. 2 Determining and understanding the high load-to-weight ratios of the grippers. (a) Scheme illustrating the experimental setup for measuring the maximum load that the gripper can hold onto. (b) The maximum load that the temperature-responsive and pH-responsive grippers (either expanded or contracted) could hold onto. The grippers gripped onto rods of different diameters as indicated in the x-axis. The asterisk (*) indicates that the load was less than 0.5 g. The corresponding (c) weights of the grippers and (d) the load-to-weight ratios. (e) The adhesive force of the surface and (f) the bulk compressive force of the stimuli-responsive hydrogels. The schemes on top illustrate the experimental setups for measuring the amount of load that the hydrogel(s) can hold onto. | |
In order to achieve higher load-to-weight ratios, we fabricated another gripper that consisted of a temperature-responsive hydrogel (Lgel = 1.2 mm and Dh = 800 μm) with its four lateral sides coated with the adhesive in its contracted state (Fig. 3a and b). The thickness of the layer of the adhesive was ∼100 μm (see the ESI,† Fig. S1). When expanded at room temperature, the hydrogel did not expand laterally outward due to the coating—instead, it expanded inward into the hole (ESI,† Video S1). Importantly, the force as a result of the expansion is directed only into the hole for gripping due to the layer of coating; hence, a large amount of force can be obtained. We determined the maximum load of the gripper, and repeated the measurement using rods of different diameters (i.e., ranging from 400–800 μm; Fig. 2b) connected to the bin as the load (ESI,† Videos S2 and S3). We also measured the weight of the hydrated gripper immediately after the measurement of the maximum load (Fig. 2c). The load-to-weight ratios of this gripper are surprisingly high—they are on the order of ∼10
000 for rods that have diameters of more than 500 μm (Fig. 2d). For a rod with a diameter of 800 μm, the load-to-weight ratio is more than 20
000. For this gripper, we optimized the chemical composition of the hydrogel for a larger gripper strength (ESI,† Section S1 and Fig. S2). This gripping force generally increased when the temperature-responsive hydrogels were fabricated to be mechanically stronger by increasing the amount of cross-linker used to polymerize the hydrogels (see the ESI,† Section S2 for more details on the experiment and results). The hole could be opened and closed reversibly (Fig. 3b and see the ESI,† Section S3 for the rate at which the hole closed and opened with time and methods for optimizing the time). The load-to-weight ratio was similar after we repeatedly contracted and expanded the gripper for five cycles. Previous studies have shown that stimuli-responsive hydrogels can be expanded and contracted many times without any decrease in performance.25–28 High load-to-weight ratios were also measured when the gripper gripped onto rods made of materials with relatively low coefficients of friction (ESI,† Section S4). The hole closed completely when the hydrogel expanded for a reasonably large Dh value relative to Lgel; hence, the gripper is not unnecessarily bulky and can grip onto small objects.
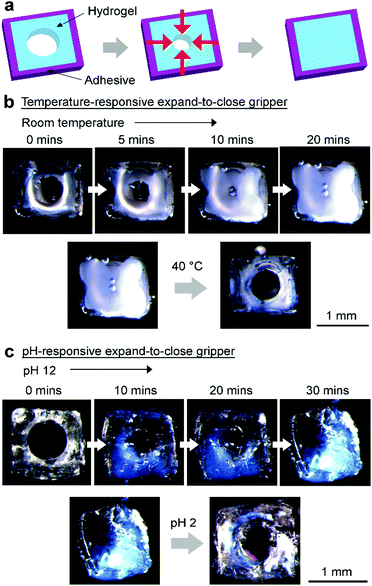 |
| Fig. 3 Soft responsive grippers with high load-to-weight ratios. (a) The gripper with a high load-to-weight ratio was fabricated by coating a slab of the stimuli-responsive hydrogel with a layer of the adhesive around its four lateral sides. The hole in the center closes when the hydrogel expands inward. (b) Experimental images of a temperature-responsive gripper. When the hydrogel expanded at room temperature, the hole closed (top row). When the hydrogel contracted at 40 °C, the hole reopened (bottom row). (c) Experimental images of a pH-responsive gripper. When the hydrogel expanded at pH = 12, the hole closed (top row). When the hydrogel contracted at pH = 2, the hole reopened (bottom row). | |
Stimuli-responsive hydrogels can be fabricated to respond to many different types of stimuli. We demonstrated the generality of the approach by fabricating a pH-responsive gripper of the same size and shape, and found that the hole in the center closed completely after the hydrogel expanded in a basic solution (e.g., pH 12; Fig. 3c). The load-to-weight ratios of this pH-responsive gripper were similarly high (Fig. 2d). In addition, we demonstrated that similar high load-to-weight ratios were obtained for another pH-responsive gripper with a different chemical composition (ESI,† Section S5).
It is interesting to note that the different types of stimuli-responsive hydrogels have similar load-to-weight ratios although they undergo fundamentally different molecular transformations for changing their sizes under the influence of different types of external stimulus (Fig. 4). Specifically, for pH-responsive hydrogels, the polymeric structure of the hydrogel contains functional groups that can be ionized under the influence of the pH of the surrounding medium. Ionization of these groups results in the electrostatic repulsion between the polymeric structures, thus causing the hydrogel to swell.29–31 For the temperature-responsive hydrogel (e.g., poly(N-isopropylacrylamide); PNIPAM), however, it has been proposed that the polymer may have undergone a hydrophobic to hydrophilic transition when the temperature is lowered; hence, the hydrogel absorbs water and expands.32–34
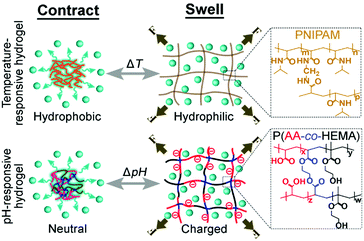 |
| Fig. 4 Chemical transformations of stimuli-responsive hydrogels. Schemes showing the chemical transformations of a temperature-responsive hydrogel (top row) and a pH-responsive hydrogel (bottom row) under the influence of the respective stimulus. The temperature-responsive hydrogel undergoes a hydrophobic to hydrophilic transition, while the pH-responsive hydrogel expands due to electrostatic repulsion of the ionized groups within the polymeric structures. When the hydrogels swell, they absorb water molecules (green spheres) and exert a force (F) on their surroundings. | |
Mechanisms of the high load-to-weight ratios
We determined that the high gripping strength of the hydrogels is due to both the adhesiveness of the surface of the hydrogel35 and the bulk compressive force derived from the directed expansion of the hydrogel into the hole. First, we observed that the surfaces of the hydrogels generally provided an adhesive force for sticking onto objects placed on their surfaces. Experimentally, we placed the stimuli-responsive gripper (i.e., either the temperature- or pH-responsive gripper) onto a rigid substrate (i.e., a piece of a glass slide), which was fixed in position using a clamp. On the opposite surface of the gripper, we placed the load that consisted of a piece of glass attached to a bin with small pieces of loads (Fig. 2e; see the ESI,† Section S6, for more details on the experimental method). The adhesiveness of the surface of the hydrogel was measured by placing an appropriate amount of small pieces of load in the bin until the load fell. Results showed that the surfaces of the grippers can adhere onto objects with significant amounts of force, except for the pH-responsive gripper in its expanded state. They adhered to higher amounts of load when contracted; this is probably because when the hydrogel absorbed more water in its expanded state, the surface became less adhesive.
For determining the bulk compressive force, we assembled a setup that consisted of two hydrogels, each resting on a rigid substrate (Fig. 2f; see the ESI,† Section S7, for more details on the experimental method); the two rigid substrates were fixed in position using a clamp and separated at a specific distance apart. At room temperature, the two hydrogels expanded toward each other and gripped onto a load. After measuring the maximum load that the hydrogels could grip onto, we repeated the experiment by using pairs of hydrogels of different thicknesses. Results showed that the gripping force increased when the thickness of the hydrogels increased (Fig. 2f); hence, more bulk material of the hydrogel contributed to a higher compressive force. This result indicated that the bulk material of the hydrogel exerted a compressive force when expanded.
A clearer comparison of the relative contribution of the forces (i.e., the surface adhesive force versus the bulk compressive force) can be made by considering the case when the hole of the gripper was fully opened (Dh = 800 μm) at the contracted state, with a rod of diameter 800 μm placed in it (Fig. 2b). The maximum load that the gripper could hold onto was 12 g for the temperature-responsive gripper, and 6 g for the pH-responsive gripper; in this case, only the surface adhesive force was responsible for holding onto the load. On the other hand, when the grippers expanded, the maximum loads were around 30 g. A majority of this force thus seemed to be due to the bulk compressive force directed into the hole since surface adhesiveness reduced when the hydrogel expanded. Hence, depending on the state of the hydrogel, both types of forces can contribute significantly to gripping the loads.
Soft grippers and machines
One important feature of these grippers is that they are soft; hence, they may grip onto objects of different shapes and sizes easily. For a demonstration, we used the temperature-responsive gripper to hold onto objects of four different shapes and cylindrical objects of two sizes (i.e., diameters of 300 μm and 500 μm; Fig. 5). When the hole closed at room temperature, we observed that the hydrogel simply wrapped itself around the object of a specific shape and size. These objects were found to be held firmly within the gripper after agitating and rotating the gripper in all directions manually and could not fall off from the gripper easily.
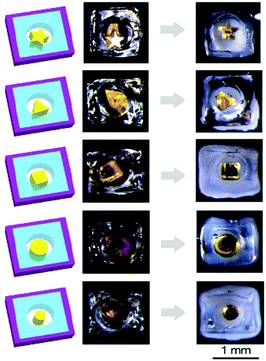 |
| Fig. 5 Gripping onto objects of different shapes and sizes easily using the soft temperature-responsive gripper. The schemes on the left illustrate the shapes and sizes of the objects used. The experimental images in the middle column show the gripper with its hole opened; the images on the right show the gripper with its hole closed and gripping onto the objects. | |
For performing more complex operations, we fabricated a soft untethered machine by assembling a temperature-responsive gripper, a block of the salt-responsive hydrogel (i.e., a hydrogel that contracts in a salt solution) that served as the base, and a block of the pH-responsive hydrogel that served as the actuating arm as illustrated in Fig. 6a. A cylindrical rod was placed below the soft machine. Initially, the medium was acidic (pH = 2), did not contain any salt, and was at 40 °C; hence, the hole of the gripper opened, the salt-responsive hydrogel expanded, and the pH-responsive hydrogel contracted. We then changed the medium to a solution that contained salt (0.01 M NaCl), while maintaining the same temperature and pH. In this medium, the salt-responsive hydrogel contracted, and the cylindrical rod was inserted into the fully opened hole of the gripper (Fig. 6b). Subsequently, the medium was cooled down to room temperature, and the gripper gripped onto the cylindrical rod. The medium was then changed to a basic solution (pH = 12), while keeping the temperature and the concentration of the salt the same. The pH-responsive hydrogel expanded, and moved the object forward horizontally (Fig. 6c). Finally, we heated the medium to 40 °C, and the gripper released the rod (Fig. 6d). The overall process thus involved moving toward the rod, gripping it, moving it forward, and releasing it at a different location.
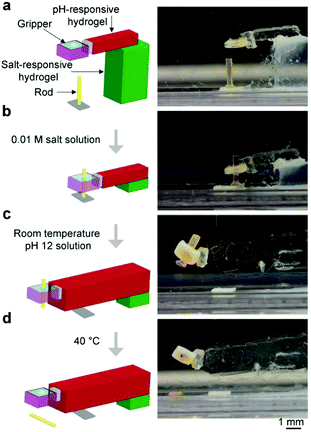 |
| Fig. 6 A soft responsive machine that grips and moves objects. (a) The machine was composed of a salt-responsive hydrogel that served as the base, a pH-responsive hydrogel that served as the actuating arm, and a temperature-responsive gripper. By changing the appropriate stimulus, the machine was controlled to (b) move toward a cylindrical rod, (c) grip onto the rod, move the rod horizontally forward, and (d) release the rod at another location. | |
Conclusions
In general, we have introduced a general class of soft untethered grippers that are stimuli-responsive and have large gripping forces of ∼10
000 times their weights. Being stimuli-responsive, this class of grippers has the unique capability of gripping onto objects specifically at a targeted site in which the stimulus is present. The design is simple: it consists of only a stimuli-responsive hydrogel with a hole in the middle and a layer of coating around its sides. The phenomenon seems to be general. We showed that both the temperature-responsive and pH-responsive hydrogels can exert large forces; hence, these forces can be achieved regardless of the molecular mechanism that underlies the transformation of the polymeric structure of the hydrogels under the influence of different types of stimulus. It is particularly surprising to achieve these high load-to-weight ratios because the grippers are soft, untethered to an external source of energy (e.g., air pressure or electricity), and involve a simple method of fabrication. In previous studies, hard materials were intentionally incorporated in order to increase the strength of the grippers.12 Other systems, such as bio-organisms (e.g., ants and walking-stick insects),36–38 untethered stimuli-responsive actuators,39 untethered robotic grippers,40,41 and tethered actuators (e.g., operated by air pressure or electricity)6,42 are reported to have lower load-to-weight ratios than the grippers we fabricated (see the ESI,† Section S8, for a summary of previously studied grippers). The load-to-weight ratio of ∼10
000 is the highest reported so far for grippers, to the best of our knowledge. Therefore, contrary to the common perception that stimuli-responsive hydrogels are soft and have weak mechanical properties, our results showed that they can nevertheless exert a large amount of force compared to other systems. We found that the large force was produced by a combination of the surface adhesiveness and the bulk compressive force as a result of the directed expansion of the hydrogel. Complex operations can be achieved by assembling modular blocks of these stimuli-responsive hydrogels with stimuli-responsive grippers for biomedical applications (e.g., minimally-invasive surgery and diagnosis).
Conflicts of interest
There are no conflicts to declare.
Acknowledgements
This work was financially supported by the Ministry of Education, Singapore, under grant R-279-000-398-133.
References
- D. Rus and M. T. Tolley, Nature, 2015, 521, 467–475 CrossRef CAS PubMed.
- M. Wehner, R. L. Truby, D. J. Fitzgerald, B. Mosadegh, G. M. Whitesides, J. A. Lewis and R. J. Wood, Nature, 2016, 536, 451–455 CrossRef CAS PubMed.
- S. A. Morin, R. F. Shepherd, S. W. Kwok, A. A. Stokes, A. Nemiroski and G. M. Whitesides, Science, 2012, 337, 828–832 CrossRef CAS PubMed.
- C. Larson, B. Peele, S. Li, S. Robinson, M. Totaro, L. Beccai, B. Mazzolai and R. Shepherd, Science, 2016, 351, 1071–1074 CrossRef CAS PubMed.
- P. C. Giulianotti, A. Coratti, M. Angelini, F. Sbrana, S. Cecconi, T. Balestracci and G. Caravaglios, Arch. Surg., 2003, 138, 777–784 CrossRef PubMed.
- J. Shintake, S. Rosset, B. Schubert, D. Floreano and H. Shea, Adv. Mater., 2016, 28, 231–238 CrossRef CAS PubMed.
- S. Shian, K. Bertoldi and D. R. Clarke, Adv. Mater., 2015, 27, 6814–6819 CrossRef CAS PubMed.
- E. Brown, N. Rodenberg, J. Amend, A. Mozeika, E. Steltz, M. R. Zakin, H. Lipson and H. M. Jaeger, Proc. Natl. Acad. Sci. U. S. A., 2010, 107, 18809–18814 CrossRef CAS.
- F. Ilievski, A. D. Mazzeo, R. E. Shepherd, X. Chen and G. M. Whitesides, Angew. Chem., Int. Ed., 2011, 50, 1890–1895 CrossRef CAS PubMed.
- R. V. Martinez, J. L. Branch, C. R. Fish, L. H. Jin, R. F. Shepherd, R. M. D. Nunes, Z. G. Suo and G. M. Whitesides, Adv. Mater., 2013, 25, 205–212 CrossRef CAS PubMed.
- K. Malachowski, M. Jamal, Q. R. Jin, B. Polat, C. J. Morris and D. H. Gracias, Nano Lett., 2014, 14, 4164–4170 CrossRef CAS PubMed.
- K. Malachowski, J. Breger, H. R. Kwag, M. O. Wang, J. P. Fisher, F. M. Selaru and D. H. Gracias, Angew. Chem., Int. Ed., 2014, 53, 8045–8049 CrossRef CAS PubMed.
- E. Gultepe, J. S. Randhawa, S. Kadam, S. Yamanaka, F. M. Selaru, E. J. Shin, A. N. Kalloo and D. H. Gracias, Adv. Mater., 2013, 25, 514–519 CrossRef CAS PubMed.
- R. Fernandes and D. H. Gracias, Adv. Drug Delivery Rev., 2012, 64, 1579–1589 CrossRef CAS PubMed.
- E. Diller and M. Sitti, Adv. Funct. Mater., 2014, 24, 4397–4404 CrossRef CAS.
- N. Bassik, A. Brafman, A. M. Zarafshar, M. Jamal, D. Luvsanjav, F. M. Selaru and D. H. Gracias, J. Am. Chem. Soc., 2010, 132, 16314–16317 CrossRef CAS PubMed.
- C. Yoon, R. Xiao, J. Park, J. Cha, T. D. Nguyen and D. H. Gracias, Smart Mater. Struct., 2014, 23, 094008 CrossRef.
- T. Miyata, T. Uragami and K. Nakamae, Adv. Drug Delivery Rev., 2002, 54, 79–98 CrossRef CAS PubMed.
- Y. Qiu and K. Park, Adv. Drug Delivery Rev., 2001, 53, 321–339 CrossRef CAS PubMed.
- Y. Chen, W. Liu and G. Zeng, RSC Adv., 2016, 6, 87422–87432 RSC.
- Y. Wang and D. Chen, J. Colloid Interface Sci., 2012, 372, 245–251 CrossRef CAS PubMed.
- A. B. Imran, T. Seki and Y. Takeoka, Polym. J., 2010, 42, 839–851 CrossRef.
- G. X. Wu, Y. Xia and S. Yang, Soft Matter, 2014, 10, 1392–1399 RSC.
- X. L. Zhu, G. X. Wu, R. Dong, C. M. Chen and S. Yang, Soft Matter, 2012, 8, 8088–8093 RSC.
- Y. Li, W. Liu, L. Cheng, P. Huang, Y. Peng, Y. Wu, X. Li, X. Li and X. Fan, J. Funct. Biomater., 2016, 7, 25 CrossRef PubMed.
- F. Guo and Z. Guo, RSC Adv., 2016, 6, 36623–36641 RSC.
- B. Xin and J. Hao, Chem. Soc. Rev., 2010, 39, 769–782 RSC.
- L. Chen, M. Liu, L. Lin, T. Zhang, J. Ma, Y. Song and L. Jiang, Soft Matter, 2010, 6, 2708–2712 RSC.
- B. Kim, K. La Flamme and N. A. Peppas, J. Appl. Polym. Sci., 2003, 89, 1606–1613 CrossRef CAS.
- N. Peppas, P. Bures, W. Leobandung and H. Ichikawa, Eur. J. Pharm. Biopharm., 2000, 50, 27–46 CrossRef CAS PubMed.
- A. R. Khare and N. A. Peppas, Biomaterials, 1995, 16, 559–567 CrossRef CAS PubMed.
- Z. Ahmed, E. A. Gooding, K. V. Pimenov, L. Wang and S. A. Asher, J. Phys. Chem. B, 2009, 113, 4248–4256 CrossRef CAS PubMed.
- H. G. Schild, Prog. Polym. Sci., 1992, 17, 163–249 CrossRef CAS.
- F. Ilmain, T. Tanaka and E. Kokufuta, Nature, 1991, 349, 400–401 CrossRef CAS.
- A. Synytska, E. Svetushkina, N. Puretskiy, G. Stoychev, S. Berger, L. Ionov, C. Bellmann, K. J. Eichhorn and M. Stamm, Soft Matter, 2010, 6, 5907–5914 RSC.
-
M. J. Madou, Fundamentals of Microfabrication: The Science of Miniaturization, CRC Press, Boca Raton, 2nd edn, 2002 Search PubMed.
- M. Burd, Behav. Ecol., 2000, 11, 125–131 CrossRef.
-
G. A. Bekey, Autonomous Robots: From Biological Inspiration to Implementation and Control, MIT Press, Cambridge, Massachusetts, 2005 Search PubMed.
- M. M. Ma, L. Guo, D. G. Anderson and R. Langer, Science, 2013, 339, 186–189 CrossRef CAS PubMed.
-
Y. Y. Liu, X. Y. Wu, H. H. Qian, D. Zheng, J. Q. Sun and Y. S. Xu, presented in part at the 2012 IEEE International Conference on Robotics and Automation, Minnesota, 14 May, 2012.
-
W. K. Chung, J. B. Li, Y. Q. Chen and Y. S. Xu, presented in part at the 2011 IEEE International Conference on Robotics and Automation, New York, 9 May, 2011.
- R. V. Martinez, C. R. Fish, X. Chen and G. M. Whitesides, Adv. Funct. Mater., 2012, 22, 1376–1384 CrossRef CAS.
Footnotes |
† Electronic supplementary information (ESI) available. See DOI: 10.1039/c8mh00888d |
‡ These authors contributed equally to this work. |
|
This journal is © The Royal Society of Chemistry 2019 |
Click here to see how this site uses Cookies. View our privacy policy here.